Stimulating endogenous cardiac repair
- Centre National de la Recherche Scientifique United Medical Resource 9214, Institut National de la Santé et de la Recherche Médicale U1046, Physiology and Experimental Medicine of the Heart and Muscles, University of Montpellier, Montpellier, France
The healthy adult heart has a low turnover of cardiac myocytes. The renewal capacity, however, is augmented after cardiac injury. Participants in cardiac regeneration include cardiac myocytes themselves, cardiac progenitor cells, and peripheral stem cells, particularly from the bone marrow compartment. Cardiac progenitor cells and bone marrow stem cells are augmented after cardiac injury, migrate to the myocardium, and support regeneration. Depletion studies of these populations have demonstrated their necessary role in cardiac repair. However, the potential of these cells to completely regenerate the heart is limited. Efforts are now being focused on ways to augment these natural pathways to improve cardiac healing, primarily after ischemic injury but in other cardiac pathologies as well. Cell and gene therapy or pharmacological interventions are proposed mechanisms. Cell therapy has demonstrated modest results and has passed into clinical trials. However, the beneficial effects of cell therapy have primarily been their ability to produce paracrine effects on the cardiac tissue and recruit endogenous stem cell populations as opposed to direct cardiac regeneration. Gene therapy efforts have focused on prolonging or reactivating natural signaling pathways. Positive results have been demonstrated to activate the endogenous stem cell populations and are currently being tested in clinical trials. A potential new avenue may be to refine pharmacological treatments that are currently in place in the clinic. Evidence is mounting that drugs such as statins or beta blockers may alter endogenous stem cell activity. Understanding the effects of these drugs on stem cell repair while keeping in mind their primary function may strike a balance in myocardial healing. To maximize endogenous cardiac regeneration, a combination of these approaches could ameliorate the overall repair process to incorporate the participation of multiple cellular players.
Introduction
Despite the significant research effort that has gone into improving therapeutics and interventions for coronary heart disease, it still remains a great societal, economic and clinical burden throughout the world. In particular, myocardial infarction is attributable to more than half of the cardiovascular disease related mortality in the industrialized world (Go et al., 2014). The coronary occlusion in a myocardial infarct results in significant cardiac cell damage and death. Current therapies are able to attenuate symptoms and prolong life. However, an intervention has not yet been developed that can replace or regenerate the damaged myocardium after an infarct. This is due in part to the absence of understanding and the potential of how the heart tissue can be repaired or regenerated.
Evidence has accumulated over the past 15 years to demonstrate that there is some amount of cardiac turnover, both in healthy and injured tissue. Particular interest has focused on cardiac regeneration after ischemic events due to the large amount of myocyte death, but reports also describe cardiac proliferation in models of cardiac pressure overload and idiopathic dilated cardiomyopathy (Kajstura et al., 1998; Urbanek et al., 2003). Cardiac cell proliferation is highest in youth, reducing with increased age (Rumyantsev and Borisov, 1987; Mollova et al., 2013; Naqvi et al., 2014). In an average healthy adult, the cardiomyocyte turnover rate is controversial with reports predicting annual turnover rates ranging anywhere from 0.3% to more than 50% (Bergmann et al., 2009; Kajstura et al., 2010; Bergmann and Jovinge, 2014). Methods have been questioned in studies where turnover is on the higher end (Laflamme and Murry, 2011). Cardiomyocyte renewal is augmented in cardiac pathological settings such as myocardial infarction and heart failure (Rumyantsev and Borisov, 1987; Kajstura et al., 1998; Beltrami et al., 2001). Due to these evidences, it appears that the heart has some capacity for renewal though it is limited and unable to compensate for significant cardiac damage. It has been proposed that evolutionary mechanisms have limited pathways of cardiac regeneration to protect the heart from developing cancer (Penn, 2010). In fact, primary cardiac tumors are extremely rare, representing only 0.3–0.7% of all cardiac tumors (Leja et al., 2011). This endogenous capability to regenerate does provoke a potential target for cardiac therapies however. Reports demonstrate multiple cellular players participating in endogenous cardiac regeneration (Figure 1). The exact contribution of each cell type remains a topic of controversy in the cardiac regenerative medicine field. Focusing the repair potential to just one cell type may actually limit the therapeutic opportunities. Instead it is likely that provoking each of these players could provide an orchestrated and more complete regeneration.
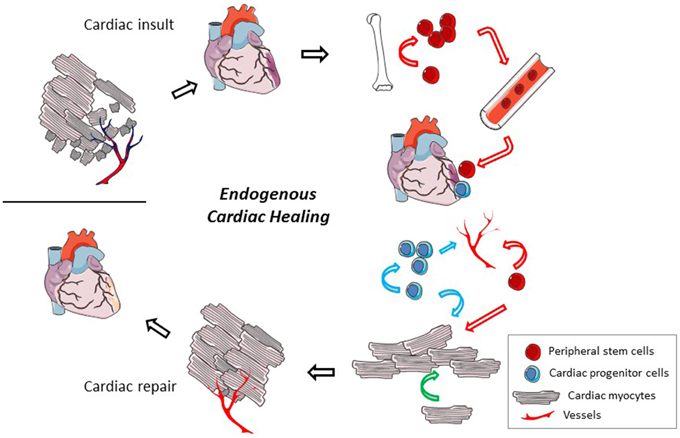
Figure 1. Endogenous cardiac regeneration. After a cardiac insult, a significant number of cardiac mycoytes die and vessel density gets reduced. In a very limited way, the heart has the ability to regenerate but it is insufficient to compensate for the total damage. The cellular participants in the endogenous regeneration process may include cardiac myocytes (pink cells, black lines), local cardiac progenitor cells (blue cells), and recruited peripheral stem cells (red cells). These cells have the potential to proliferate and participate in the regeneration of cardiac myocytes, angiogenesis, and the release of trophic factors that may reduce cardiac cell death. Modes of the cells participation are identified by colored arrows (peripheral stem cell, red; cardiac progenitor cell, blue; cardiac myocyte, green). Methods that could be employed to improve these endogenous mechanisms include cell and/or gene therapy and pharmacologic treatments.
Participants in Cardiac Regeneration
Cardiac Myocytes
Amputation studies in zebrafish and neonatal mice brought to light the potential for cardiac regeneration by cardiac myocytes themselves (Poss et al., 2002; Porrello et al., 2011). The mechanisms proposed include the activation of cardiogenesis and cell cycle genes and dedifferentiation (Jopling et al., 2010; Kikuchi et al., 2010; Porrello et al., 2011; Mahmoud et al., 2013). However, this capacity for complete regeneration in the mouse is lost by 1 week of age (Porrello et al., 2011). Studies are incongruous on whether cardiac myocyte proliferation continues into adult life (Walsh et al., 2010; Senyo et al., 2013). It does appear likely that myocardial injury can induce to some extent pre-existing cardiac myocyte renewal (Senyo et al., 2013).
Cardiac Progenitor Cells
Cardiac progenitor cells (CPC) are an adult stem cell population residing in the heart. These cells have been identified by a number of markers including c-kit, sca-1, Isl1, Wilms tumor 1, and the ability to form cardiospheres in vitro (Beltrami et al., 2003; Genead et al., 2010; Smart et al., 2011; Hsiao et al., 2014; Uchida et al., 2013). It is evident that after a cardiac injury these cell types proliferate and increase in number (Fransioli et al., 2008; Smart et al., 2011; Uchida et al., 2013). It has even been suggested that these cells are indispensable for cardiac regeneration (Ellison et al., 2013). Disagreement exists on the exact differentiation capabilities of these cells in vivo, particularly with the c-kit+ CPC population. Research consistently reports the ability of the cells to differentiate into an endothelial cell lineage but their contribution to cardiac myocytes has been questioned (Hsieh et al., 2007; Fransioli et al., 2008; Jesty et al., 2012). Ellison et al. found that c-kit+ CPC significantly contribute to de novo cardiac myocyte generation; whereas van Berlo et al. reported that differentiation of c-kit+ cells to cardiac myocytes is negligible (Ellison et al., 2013; Van Berlo et al., 2014). This discrepancy may in part be due to the different transgenic mouse lines used between the two studies. A study with the same mouse line used in the Ellison study demonstrated that of the c-kit+ cells identified by immunofluorescence, only 32% of the cells were positive for fluorescent protein driven by the c-kit promoter (Fransioli et al., 2008). This suggests that the results of the Ellison study may potentially underestimate the involvement of c-kit+ cells. Regardless of these issues, it is evident that CPC are responsive to cardiac injury and differentiate into endothelial cells, aiding in increased vessel density, a mechanism correlated to improved cardiac function (Kim et al., 2014).
Peripheral Stem Cells
The bone marrow is a reservoir for multiple populations of adult stem cells including, but not limited to, endothelial progenitor cells (EPC), c-kit+ stem cells, very small embryonic like stem cells, mesenchymal stem cells (MSC), and hematopoietic stem cells. Bone marrow cells are mobilized into the peripheral blood after cardiac injury and are recruited to the myocardium (Mouquet et al., 2005; Finan et al., 2012). The recruitment of bone marrow cells is associated with cardiac repair and along the same lines, in studies where the bone marrow stem cells response is attenuated, reduced accumulation of bone marrow cells is associated with worse outcomes (Fazel, 2006; Sopko et al., 2010; Finan et al., 2013; Gong et al., 2014). Bone marrow stem cells can incorporate into the vasculature, differentiate into endothelial cells, and participate in vascular remodeling (Finan et al., 2013; Wu et al., 2015). Studies of gender mismatched bone marrow and heart transplant patients demonstrated that bone marrow cells have the capacity to differentiate into cardiac myocytes (Quaini et al., 2002; Deb et al., 2003). However, a recent study suggests that bone marrow cells do not directly differentiate into cardiac myocytes and instead suggests their fusion with existing cardiac myocytes (Wu et al., 2015). Bone marrow cells may also replenish the diminished CPC pool after a cardiac injury (Mouquet et al., 2005; Barile et al., 2011).
Improvement of Endogenous Cardiac Healing
It is clear that the heart has some capacity to regenerate through various cell participants to support normal deterioration. Unfortunately, it is evident that the potential is limited and unable to compensate for the extent of damage that may occur after a myocardial infarction. A number of approaches have been attempted to improve cardiac regeneration, including cell and gene therapy. Reasonable benefits have been determined from these therapeutic approaches though the beneficial mechanisms lie in the trophic factors produced or through the activation of endogenous cardiac healing. Recently, it is has been identified that common drugs administered to patients with cardiovascular disease may alter endogenous stem cell populations. Profiting from these interactions may be a potential manner to assist in cardiac repair.
Cell Therapy
Attempts to repair the damaged myocardium with cardiac myocytes or skeletal myocytes have shown limited potential. A large amount of enthusiasm therefore has focused on the potential that stem cells could have in cardiac regenerative therapy. Many stem cell types have been examined including embryonic stem cells, induced pluripotent stem cells, various bone marrow stem cell types, and cardiac stem cells. Cell therapy efforts have been reviewed more exhaustively elsewhere (Goumans et al., 2014; Pavo et al., 2014).
Embryonic stem cells (ESC) and induced pluripotent stem cells have a great power to differentiate into all cell types making them an attractive choice for replacing lost myocardium. While efforts have been made to program these stem cell types to reduce tumor potential, it still remains a risk as well as a potential immunological challenge in the recipient (Anderson et al., 2014; De Almeida et al., 2014; Zhang et al., 2015). Cardiac myocytes derived from human pluripotent stem cells were shown to be anti-arrhythmic in a guinea pig model (Shiba et al., 2012). However, in a large primate model, the transplantation of human ESC derived cardiac myocytes was associated with ventricular arrhythmias, although they were transient and did not induce sudden death (Chong et al., 2014). As the human heart has a significantly slower heart rate and larger size than the primate model, the propensity of arrhythmias from ESC transplant increases (Lalit et al., 2014). For this reason, the transplantation of ESC derived cardiac myocytes needs to be tested with caution in humans (Anderson et al., 2014).
Due to the issues that still remain with pluripotent stem cells, adult stem cells could be a more plausible option for cell therapy. Autologous adult stem cells can be delivered to patients reducing any risk for immune complications. As these cell types have a more differentiated lineage, no concerns for tumor formation remain. In animal models, cardiac cell therapy with bone marrow stem cells or cardiac stem cells can improve cardiac function, decrease infarct size, improve myocardial regeneration, increase vascular density, and activate endogenous stem cell populations (Figure 2; Yoon et al., 2010; Loffredo et al., 2011; Dong et al., 2012; Avolio et al., 2015). These benefits seem to translate into similar results in a portion of human clinical trials. Individual reports have described improved cardiac function, decreased scar size, and improved physical capabilities, such as improvements in the 6 min walking distance test, after adult stem cell therapy (Bolli et al., 2011; Malliaras et al., 2014b; Golpanian et al., 2015; Mathiasen et al., 2015). However, results from the meta-analysis of cell based cardiac studies revealed no clinical benefit 3–12 months after cell therapy (Gyöngyösi et al., 2015).
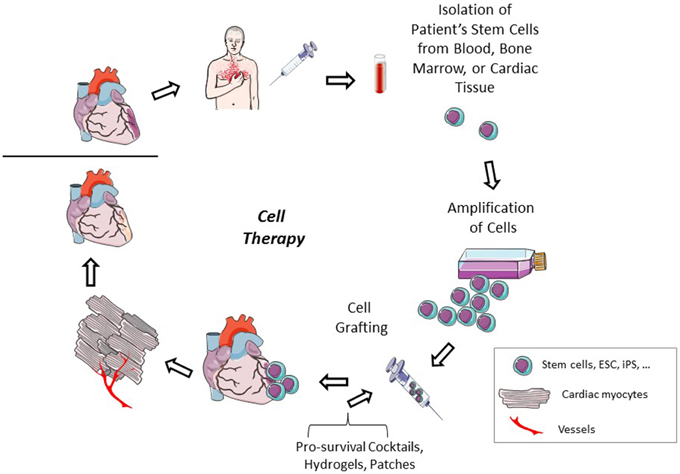
Figure 2. Cardiac cell therapy. After a cardiac injury, a patient's adult stem cells are isolated. These cells can be isolated from either cardiac tissue or from peripheral sites. The cells are amplified to have a sufficient number for therapeutic transplant. The cells are injected into the patient either intravenously or intracardiac, alone or in the presence of pro-survival cocktails, hydrogels, or extracellular matrix patches. The injected cells can participate themselves in cardiac regeneration or improve endogenous healing by excreting paracrine factors which reduce apoptosis and increase stem cell recruitment and activity and angiogenesis to repair the damaged cardiac tissue.
The beneficial mechanism of adult stem cells has not been direct differentiation to cardiac myocytes as originally hoped for. Instead the more prominent mechanism appears to involve the activation of endogenous healing pathways through paracrine factors provided by the transplanted cells. These pathways can reduce myocardial apoptosis, activate cardiac myocytes proliferation and endogenous stem cell recruitment (Zhang et al., 2007; Fischer et al., 2009; Malliaras et al., 2014a; Avolio et al., 2015). Cell therapy also aids in improving vascular density in the damaged myocardium either through direct differentiation or activating endogenous angiogenesis. The importance of this mechanism to cardiac repair was highlighted recently (Yoon et al., 2010). In this study, infarcted mice were treated with ganciclovir after MSC therapy to induce cytotoxicity in stem cell derived endothelial cells. The death of the endothelial cells and the subsequent decrease in vessel density led to a significant deterioration of cardiac function. Even though cell therapy approaches may not themselves replace the lost cardiac myocytes, their release of trophic factors that reduce apoptosis or recruit endogenous stem cells and their ability to aid in vascular remodeling may provide a significant benefit to cardiac repair.
An additional point to note is that the retention of the stem cells in the myocardium is extremely low, reducing their potential therapeutic effects. The transplantation of sheets of cells or in combination with hydrogels or patches improve the cell engraftment rate, thus improving cardiac function (Ishii et al., 2014; Roche et al., 2014; Tano et al., 2014; Zhang et al., 2015). Interestingly, even altering the growth media of the cells can improve their retention in the tissue (Kim et al., 2014). Pro-survival cocktails can also be used to improve the duration and number of the cells in the myocardium (Laflamme et al., 2007; Gautam et al., 2015). Components of the pro-survival cocktails include growth factors, anti-apoptotic molecules such as cell-permeant Bcl-XL and caspase inhibitors, immunosuppressants, and vasodilators.
Gene Therapy
Natural reparative signaling pathways occur in the heart after a cardiac injury. However, these signals are usually transient, not enduring long enough to support complete endogenous repair (Askari et al., 2003; Schenk et al., 2007). As discussed in the previous section, transplanted stem cells provide paracrine factors that support natural tissue repair. Gene therapy is a mechanism to capitalize on these natural factors to prolong or reactivate the signals. Cardiac diseases gene therapy has encompassed delivery options including adenovirus, direct plasmid transfer, microbubbles and modified RNA.
Growth factors and chemokines are greatly implicated in cardiac repair and their expression through gene therapy techniques can improve cardiac function (Kanashiro-Takeuchi et al., 2011). Beneficial mechanisms induced by this approach include elevated proliferation, mobilization, and recruitment of endogenous local and peripheral stem cell populations (Figure 3). A number of growth factor or cytokine candidates have been demonstrated to be favorable in cardiac repair, including, but not limited to, vascular endothelial growth factor, monocyte chemotactic protein 3, and placental growth factor (Schenk et al., 2007; Iwasaki et al., 2011; Zangi et al., 2013). In particular, stromal derived factor-1 expression can recruit various C-X-C chemokine receptor type 4 positive cell populations including CPC, MSC and very small embryonic like stem cells (Askari et al., 2003; Kucia et al., 2006; Dong et al., 2012). Stem cell factor gene therapy has been described to induce cardiac myocyte and CPC proliferation (Yaniz-Galende et al., 2012; Ishikawa et al., 2015). Gene therapy by direct injection of growth factor or chemokine plasmids, namely stromal derived factor-1 and vascular endothelial growth factor, has been demonstrated in patients with ischemic heart disease to be safe and show signs of a functional benefit (Favaloro et al., 2013; Penn et al., 2013). In particular, stromal derived factor-1 plasmid injection into cardiac heart failure patients has shown functional therapeutic promise by improving ventricular remodeling in patients with more advanced cardiac dysfunction (Chung et al., 2015).
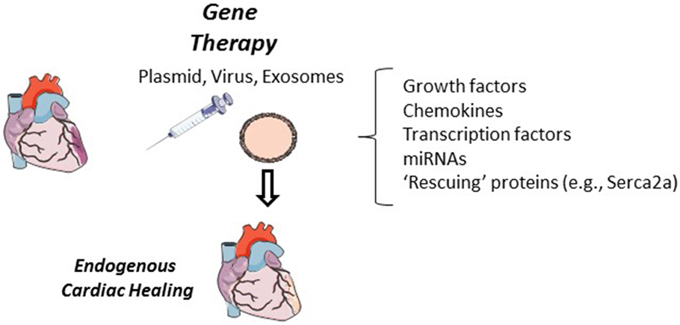
Figure 3. Cardiac gene therapy. In animal models, cardiac gene therapy has shown to be successful when delivered by direct plasmid, in a lenti- or adenovirus, or in exosomes. Growth factors, chemokines, transcription factors and miRNAs have all been tested in cardiac gene therapy. Expression of these proteins and miRNAs have the potential to improve endogenous cardiac regeneration through the recruitment, activation and differentiation of local and peripheral stem cells, improved vascular density, or by proliferation of cardiac myocytes. Additional cardiac “rescuing” gene therapy targets (e.g., Serca2a) can improve cardiac function but it has yet to been investigated if these approaches can alter endogenous cardiac regeneration.
Transcription factors have also been targeted in cardiac gene therapy. Hypoxia inducible factor 1-alpha has been an appealing target as it is protective by regulating angiogenesis and survival, as well as the expression of chemokines capable of recruiting stem cells (Semenza, 2014). Plasmid or adenovirus transfer of hypoxia inducible factor 1-alpha into the myocardium can improve cardiac repair, decrease cardiac myocyte apoptosis, mobilize bone marrow stem cells to the heart, and activate c-kit+ cells in the myocardium (Huang et al., 2011, 2014; Ong et al., 2014). In vivo reprogramming of fibroblasts with the cardiac transcription factors GATA4, Mef2c, and Tbx5 generates functional cardiac myocytes which are fully incorporated in the myocardium and decrease scar size, thus improving cardiac function (Inagawa et al., 2012; Qian et al., 2012). An additional tested approach is the manipulation directly of the proliferation of the cardiac myocytes. Injection of an adenovirus expressing cardiac specific telomerase or the Yes-associated protein can induce cardiac myocyte proliferation and improve overall cardiac repair (Bär et al., 2014; Lin et al., 2014).
MicroRNAs (miRNAs) have also been employed in gene therapy techniques to promote cardiac regeneration. miRNAs are small non-coding RNAs that bind mRNA and inhibit translation. Eulalio et al. performed a high throughput screening of miRNAs on neonatal rat cardiac myocytes to identify candidates that induce proliferation (Eulalio et al., 2012). They then demonstrated that in fact when an adenovirus expressing either miR-590-3p or miR-199a was injected at the time of myocardial infarction, they could induce cardiac myocyte proliferation resulting in decreased infarct size and improved cardiac function. Endogenous CPC proliferation was elevated after lentiviral delivery of miR-17-92 (Sirish et al., 2012). The in vivo reprogramming of cardiac fibroblasts to cardiac myocytes by lentiviral transfer of a combination of miRNAs (1, 133, 208, 499) has also been demonstrated to be a successful approach to induce endogenous cardiac regeneration (Jayawardena et al., 2015). miRNAs can also be a target of inhibition. To combat the anti-angiogenic properties of miR-92a, an antagomir was designed to inhibit the miRNA thus increasing capillary density after myocardial infarction (Bonauer et al., 2009). The potential of miRNAs in cardiac repair has been reviewed more thoroughly elsewhere (Hodgkinson et al., 2015).
Additional targets of cardiac gene therapy have included calcium cycling proteins, extracellular matrix alterations, adrenergic manipulation, and arrhythmias and have been reviewed elsewhere (Nagai and Komuro, 2012; Scimia et al., 2013). These approaches appear to have a sufficient effect on their target and improve cardiac function but besides the mention that targeting calcium cycling proteins can block cardiac remodeling, little is known on the effect of these strategies and the survival or regeneration of the tissue (Katz et al., 2014). It would be interesting to examine the cell proliferation or anti-apoptotic effects of these therapies especially as atrial fibrillation and changes in calcium channels and intracellular calcium changes can alter cardiac myocyte and CPC proliferation (Ferreira-Martins et al., 2009; Chernyavskaya et al., 2012; Gambini et al., 2012; Demion et al., 2014).
Pharmacological Interventions
The common pharmaceutics given to patients who have had a myocardial infarction include aspirin, beta blockers, ace inhibitors, angiotensin receptor blockers, and statins. These drugs aim at reducing blood clots, regulating blood pressure, stabilizing electrical activity, and reducing cholesterol. These approaches improve patient lifestyle and prolong life but they do not replace the damaged tissue that is lost after a myocardial infarction. However, evidence is mounting that these drugs may actually have either positive or negative effects on endogenous stem cell populations and cardiac myocyte survival and proliferation (Table 1). Potential may exist in refining current therapies to control their primary functions and generate a balance in myocardial healing. These drug regiments, as well as, patient's age and pathophysiological state likely have grave implications on endogenous stem cell activity as well as exogenous cell therapy approaches. Increased age and cardiovascular comorbidities have well been described to impair endogenous cardiac repair (Gambini et al., 2012; Lee and Poh, 2014; Hariharan and Sussman, 2015). Research regarding the effects of these parameters has been limited in animal studies. Awareness of these effects will allow clinicians to anticipate potential adverse effects and facilitate maximal results.
Aspirin
Aspirin can reduce the risk of myocardial infarction in high-risk patients and prevent an additional event. However, contradictions exist with aspirin including an increased risk of gastrointestinal bleeding. Chronic treatment with aspirin may inhibit the cardiac proteasome and reduce cardiac myocyte viability (Tan et al., 2013). Interestingly, aspirin may have negative effects on endogenous stem cell populations as well. Aspirin can reduce EPC number and migration capacity (Boer et al., 2011; Sato et al., 2013). Aspirin may also inhibit MSC proliferation and induce apoptosis (Deng et al., 2009). No reports have yet described the effects of aspirin on CPC but as non-steroidal anti-inflammatory drugs can negatively affect ESC cardiac differentiation, it could have an implication on in vivo cardiac differentiation as well (Chillar et al., 2011). The negative effects of aspirin on endogenous stem cells may be due to its anti-inflammatory effects. Pro-inflammatory signals have been demonstrated to aid in regulating and activating endogenous stem cell populations (Reber et al., 2006; Mirantes et al., 2014).
Beta Blockers
After a myocardial infarction, an excessive number of catecholamines are released. To reduce their deleterious action, beta adrenergic receptor blocking agents are administered to patients. These drugs aid in the prevention of additional infarcts and reduce cardiac arrhythmias. The majority of the inotropic effects of the beta adrenergic signaling pathway are mediated by the beta 1 receptor (Bristow et al., 1986). This pathway as well can promote cardiac myocytes apoptosis (Seqqat et al., 2012). Conversely, beta 2 adrenergic receptor signaling is anti-apoptotic and cardioprotective (Zhang et al., 2010). Interestingly, general blockade of beta adrenergic receptor signaling by propanolol in cardiac pressure overload actually induces cell cycle gene expression and increases the number of ki-67 proliferating cardiac myocytes primarily through the beta 2 receptor pathway (Musumeci et al., 2011).
Catecholamines can stimulate proliferation in endogenous CPC through beta 2 adrenergic receptor signaling which is the more highly expressed beta adrenergic receptor on these cells (Ellison et al., 2007). Similarly to cardiac myocytes, stimulation of beta 1 adrenergic receptor can induce CPC apoptosis (Khan et al., 2013). Remarkably, a higher number of isolated CPC has been associated with patients on beta blocker therapy (Gambini et al., 2012). Beta adrenergic signaling manipulation may also alter peripheral stem cell activity. The beta 1 specific adrenergic receptor blocker, nebivolol, can increase EPC number after myocardial infarction (Sorrentino et al., 2011). Clenbuterol, a beta 2 receptor agonist, along with the chemokine GCSF, augments peripheral blood CD34+ cells after myocardial infarction (Tanaka et al., 2013). Research is scarce on the beta adrenergic receptor signaling role in endogenous stem cell differentiation. As activation of this pathway can improve cardiac differentiation in ESC, it may be interesting in future studies to examine CPC and peripheral stem cell differentiation after manipulation of this pathway (Yan et al., 2011).
Clearly manipulation of the beta adrenergic receptor pathway may improve endogenous cardiac regeneration. Stimulating the beta 2 receptor could likely reduce cardiac myocytes apoptosis and improve the local and peripheral endogenous stem cell reservoir. It is likely that treatment with a beta 1 receptor specific blocker and a beta 2 specific agonist may provide a balance in cardiac therapy as recently demonstrated (Sun et al., 2014).
Angiotensin Converting Enzyme Inhibitors/Angiotensin Receptor Blockers
The renin/angiotensin aldosterone system (RAAS) is altered in cardiovascular diseases. In heart failure and after a myocardial infarction, drugs (e.g., angiotensin converting enzyme inhibitors, angiotensin receptor angatonists) which block this pathway are recommended to relax blood vessels, thereby reducing blood pressure (Mentz et al., 2013). These drugs may aid in reducing apoptosis of cardiac myocytes as well. Angiotensin II signaling can increase apoptosis of cardiac myocytes (Kajstura et al., 1997; Xu et al., 2010). Conversely, the benefits of these drugs are unclear for stem cells. For example, one report described that the activation of angiotensin like-1 receptor after myocardial infarction increased the accumulation of vascular stem cells, while another article found instead that blockade of the signaling increases the number of EPC (Jung et al., 2012; Li et al., 2012). Opposing results also exist on the angiogenic properties of EPC in the presence of RAAS signaling (Thum et al., 2011; Durik et al., 2012). The RAAS may actually be beneficial for other stem cell types; improving cardiac differentiation of ESC and MSC and rendering MSC more apoptotic resistant and amplifying their growth factor expression (Durik et al., 2012; Wu et al., 2013; Liu et al., 2015). Little has been investigated on the RAAS and CPC. The only article published to date identifies a specific angiotensin 1 receptor expressing CPC population that is senescent with impaired growth and an increased susceptibility to apoptosis (D'Amario et al., 2011). Overall, the use of drugs antagonizing the RAAS are beneficial toward cardiac function and cardiac myocyte survival, but their use in stem cell mediated regeneration requires a further, more detailed investigation.
Statins
Statins are cholesterol lowering drugs and have also been associated with having anti-inflammatory and anti-thrombotic effects (Angeli et al., 2012). Statin treatment has been described to block apoptosis of cardiac myocytes and can elevate the number of proliferating cardiac myocytes after a myocardial infarct (Ito et al., 2004; Suzuki et al., 2009; Tang et al., 2011; Zhang et al., 2012). Statins also have positive effects on endogenous stem cell populations. Progenitor cells in the heart are elevated after statin treatment and myocardial infarction (Suzuki et al., 2009). The amplification potential of CPC is significantly higher from patients on statins (Gambini et al., 2012). Bone marrow stem cells and EPC are also positively regulated by statins. Elevated number, mobilization, proliferation, improved differentiation capacity, and increases in survival have all been described in peripheral stem cell populations after statin treatment (Erbs et al., 2011; Shaw et al., 2011; Cai et al., 2013; Cohen et al., 2013). Taken all together, statin therapy appears to enhance endogenous cardiac repair pathways.
Additional Approaches
A few novel approaches have been described in recent years to improve endogenous stem cell mediated cardiac regeneration. A unique idea proposed was to find a method to trap the circulating stem cells to the tissue where their action is required. Baumer et al. generated a bi-functional fusion protein that binds damaged vessels and CD133+ progenitor cells (Baumer et al., 2012). They were able to demonstrate that this molecule could improve progenitor cell adhesion thereby increasing capillary density and improving cardiac function. Tetravalent antibodies have also been employed for the same goal. These antibodies were generated to target an epitope on apoptotic cardiac myocytes, CD34, CD133, and c-kit cells (Malecki et al., 2013). This approach significantly improved the recruitment and retention of the various stem cell populations to the damaged myocardium.
As described above, stem cell populations provide trophic factors to the damaged myocardium. Due to this idea, exosomes have been exploited as a possible means to improve cardiac regeneration. In fact, ESC exosome delivery after a myocardial infarct improved overall cardiac function, increased capillary density and proliferating cardiac myocytes and CPC and decreased cardiac myocyte apoptosis (Khan et al., 2015). This suggests a unique strategy to improve endogenous cardiac repair. Future studies will determine if exosomes from other stem cells types, such as MSC which produce a large number of paracrine signals, can enhance regeneration even more.
Prospects and Conclusions
In summary, the heart has some capacity to regenerate in response to a large insult such as a myocardial infarct, though this process needs to be amplified to repair the tissue. The endogenous regeneration requires an orchestrated participation from various cell types including cardiac myocytes and local and peripheral stem cells. It is naïve to think that only one cell type can participate in this regenerative process and may actually limit therapeutic opportunities.
As discussed in this review, known pharmacological therapies may alter endogenous cardiac regeneration. Future studies are required to expand on this topic to identify treatments that will strike a balance between the drug's primary function and ability to aid or inhibit cardiac regeneration. For example, it may be beneficial to refine the treatments so that they target only specific pathways. A good example of this is in the use of beta blockers. Physicians could choose to treat patients with a beta 1 specific adrenergic blocker, as opposed to a non-specific beta blocker, in combination with a beta 2 specific adrenergic agonist accommodating blockade of the deleterious beta adrenergic signaling (beta 1) and amplifying regenerative downstream effects (beta 2). Statins also appear to be candidates that aid in endogenous cardiac regeneration. Further studies will need to validate this and may identify the mechanisms in which statins can alter endogenous cells, potentially leading to more focused regenerative therapeutic targets. The inhibitory repair effects of drugs such as aspirin may be taken into account in determining a patient's drug regimen.
Refining current pharmacological therapies to enhance endogenous cardiac regeneration has economic and practical benefits for treatment. These drugs are already in current use in clinical practice. As well, generic, low-cost versions of these drugs are available; statin treatment may cost as little as $4 dollars per month (Lazar et al., 2011). Cell and gene therapy approaches are much more expensive and still require approval from regulatory agencies. The costs of preparation and processing of these drugs under good manufacturing procedures is very high. The first gene therapy drug on the market, although for a genetic disease, costs more than $40,000 per vial (Burger and Hirschler, 2014). Clinical administration and multiple treatments augment the price for this type of therapy even more. However, if these therapies can eventually to be proven to heal the heart, then the cost-benefit may be worth the initial price.
To truly improve endogenous cardiac regeneration, the field needs to open itself to the possibility that therapy includes more participants. A multidimensional approach will likely ameliorate the overall repair process, resulting in a more complete regeneration. This is already evident in studies combining both cell and gene therapy where a greater cardiac repair is reported (Schenk et al., 2007; Huang et al., 2014; Ong et al., 2014). One can foresee a multi-faceted treatment plan for patients including pharmacological approaches and cell and gene therapy. This perspective has the ability to translate into other fields of regenerative medicine where endogenous healing may exist, including the central nervous system, diabetes, or bone and cartilage damage.
Conflict of Interest Statement
The authors declare that the research was conducted in the absence of any commercial or financial relationships that could be construed as a potential conflict of interest.
Acknowledgments
This work was supported by a Marie Curie Actions—International Incoming Fellowship, Heart Betas - 2013 - N° 329434. Figures were generated with aid of Servier Medical Art.
References
Anderson, M. E., Goldhaber, J., Houser, S. R., Puceat, M., and Sussman, M. A. (2014). Embryonic stem cell–derived cardiac myocytes are not ready for human trials. Circ. Res. 115, 335–338. doi: 10.1161/CIRCRESAHA.114.304616
Angeli, F., Reboldi, G., Mazzotta, G., Garofoli, M., Cerasa, M. F., and Verdecchia, P. (2012). Statins in acute coronary syndrome: very early initiation and benefits. Ther. Adv. Cardiovasc. Dis. 6, 163–174. doi: 10.1177/1753944712452463
Askari, A. T., Unzek, S., Popovic, Z. B., Goldman, C. K., Forudi, F., Kiedrowski, M., et al. (2003). Effect of stromal-cell-derived factor 1 on stem-cell homing and tissue regeneration in ischaemic cardiomyopathy. Lancet 362, 697–703. doi: 10.1016/S0140-6736(03)14232-8
Avolio, E., Meloni, M., Spencer, H. L., Riu, F., Katare, R., Mangialardi, G., et al. (2015). Combined intramyocardial delivery of human pericytes and cardiac stem cells additively improves the healing of mouse infarcted hearts through stimulation of vascular and muscular repair. Circ. Res. 115:306146. doi: 10.1161/CIRCRESAHA.115.306146
Bär, C., Bernardes de Jesus, B., Serrano, R., Tejera, A., Ayuso, E., Jimenez, V., et al. (2014). Telomerase expression confers cardioprotection in the adult mouse heart after acute myocardial infarction. Nat. Commun. 5:5863 doi: 10.1038/ncomms6863
Barile, L., Cerisoli, F., Frati, G., Gaetani, R., Chimenti, I., Forte, E., et al. (2011). Bone marrow-derived cells can acquire cardiac stem cells properties in damaged heart. J. Cell. Mol. Med. 15, 63–71. doi: 10.1111/j.1582-4934.2009.00968.x
Baumer, Y., Leder, C., Ziegler, M., Schönberger, T., Ochmann, C., Perk, A., et al. (2012). The recombinant bifunctional protein αCD133–GPVI promotes repair of the infarcted myocardium in mice. J. Thromb. Haemost. 10, 1152–1164. doi: 10.1111/j.1538-7836.2012.04710.x
Beltrami, A. P., Barlucchi, L., Torella, D., Baker, M., Limana, F., Chimenti, S., et al. (2003). Adult cardiac stem cells are multipotent and support myocardial regeneration. Cell 114, 763–776. doi: 10.1016/S0092-8674(03)00687-1
Beltrami, A. P., Urbanek, K., Kajstura, J., Yan, S.-M., Finato, N., Bussani, R., et al. (2001). Evidence that human cardiac myocytes divide after myocardial infarction. N. Engl. J. Med. 344, 1750–1757. doi: 10.1056/NEJM200106073442303
Bergmann, O., Bhardwaj, R. D., Bernard, S., Zdunek, S., Barnabé-Heider, F., Walsh, S., et al. (2009). Evidence for cardiomyocyte renewal in humans. Science 324, 98–102. doi: 10.1126/science.1164680
Bergmann, O., and Jovinge, S. (2014). Cardiac regeneration in vivo: mending the heart from within? Stem Cell Res. 13(3 Pt B), 523–531. doi: 10.1016/j.scr.2014.07.002
Boer, H. C., de Hovens, M. M., Oeveren-Rietdijk, A. M., van Snoep, J. D., Koning, E. J. P., de Tamsma, J. T., et al. (2011). Human CD34+/KDR+ cells are generated from circulating CD34+ cells after immobilization on activated platelets. Arterioscler. Thromb. Vasc. Biol. 31, 408–415. doi: 10.1161/ATVBAHA.110.216879
Bolli, R., Chugh, A. R., D'Amario, D., Loughran, J. H., Stoddard, M. F., Ikram, S., et al. (2011). Cardiac stem cells in patients with ischaemic cardiomyopathy (SCIPIO): initial results of a randomised phase 1 trial. Lancet 378, 1847–1857. doi: 10.1016/S0140-6736(11)61590-0
Bonauer, A., Carmona, G., Iwasaki, M., Mione, M., Koyanagi, M., Fischer, A., et al. (2009). MicroRNA-92a controls angiogenesis and functional recovery of ischemic tissues in mice. Science 324, 1710–1713. doi: 10.1126/science.1174381
Bristow, M. R., Ginsburg, R., Umans, V., Fowler, M., Minobe, W., Rasmussen, R., et al. (1986). Beta 1- and beta 2-adrenergic-receptor subpopulations in nonfailing and failing human ventricular myocardium: coupling of both receptor subtypes to muscle contraction and selective beta 1-receptor down-regulation in heart failure. Circ. Res. 59, 297–309. doi: 10.1161/01.RES.59.3.297
Burger, L., and Hirschler, B. (2014). First gene therapy drug sets million-euro price record. Reuters. Available online at: http://www.reuters.com/article/2014/11/26/us-health-genetherapy-price-idUSKCN0JA1TP20141126
Cai, A., Qiu, R., Li, L., Zheng, D., Dong, Y., Yu, D., et al. (2013). Atorvastatin Treatment of Rats with Ischemia-Reperfusion Injury Improves Adipose-Derived Mesenchymal Stem Cell Migration and Survival via the SDF-1α/CXCR-4 Axis. PLoS ONE 8:e79100. doi: 10.1371/journal.pone.0079100
Chernyavskaya, Y., Ebert, A. M., Milligan, E., and Garrity, D. M. (2012). Voltage-gated calcium channel CACNB2 (β2.1) protein is required in the heart for control of cell proliferation and heart tube integrity. Dev. Dyn. Off. Publ. Am. Assoc. Anat. 241, 648–662. doi: 10.1002/dvdy.23746
Chillar, A., So, S.-P., Ruan, C.-H., Shelat, H., Geng, Y.-J., and Ruan, K.-H. (2011). A profile of NSAID-targeted arachidonic acid metabolisms in human embryonic stem cells (hESCs): implication of the negative effects of NSAIDs on heart tissue regeneration. Int. J. Cardiol. 150, 253–259. doi: 10.1016/j.ijcard.2010.04.015
Chong, J. J. H., Yang, X., Don, C. W., Minami, E., Liu, Y.-W., Weyers, J. J., et al. (2014). Human embryonic-stem-cell-derived cardiomyocytes regenerate non-human primate hearts. Nature 510, 273–277. doi: 10.1038/nature13233
Chung, E. S., Miller, L., Patel, A. N., Anderson, R. D., Mendelsohn, F. O., Traverse, J., et al. (2015). Changes in ventricular remodelling and clincal status during the year following a single adminstration of stromal cell-derived factor-1 non-viral gene therapy in chronic ischaemic heart failure patients: the STOP-HF randomized Phase II trial. Eur. Heart J. 36, 2228–2238. doi: 10.1093/eurheartj/ehv254
Cohen, K. S., Cheng, S., Larson, M. G., Cupples, L. A., McCabe, E. L., Wang, Y. A., et al. (2013). Circulating CD34+ progenitor cell frequency is associated with clinical and genetic factors. Blood 121, e50–e56. doi: 10.1182/blood-2012-05-424846
D'Amario, D., Cabral-Da-Silva, M. C., Zheng, H., Fiorini, C., Goichberg, P., Steadman, E., et al. (2011). Insulin-like growth factor-1 receptor identifies a pool of human cardiac stem cells with superior therapeutic potential for myocardial regeneration. Circ. Res. 108, 1467–1481. doi: 10.1161/CIRCRESAHA.111.240648
de Almeida, P. E., Meyer, E. H., Kooreman, N. G., Diecke, S., Dey, D., Sanchez-Freire, V., et al. (2014). Transplanted terminally differentiated induced pluripotent stem cells are accepted by immune mechanisms similar to self-tolerance. Nat. Commun. 5:3903. doi: 10.1038/ncomms4903
Deb, A., Wang, S., Skelding, K. A., Miller, D., Simper, D., and Caplice, N. M. (2003). Bone marrow–derived cardiomyocytes are present in adult human heart a study of gender-mismatched bone marrow transplantation patients. Circulation 107, 1247–1249. doi: 10.1161/01.CIR.0000061910.39145.F0
Demion, M., Thireau, J., Gueffier, M., Finan, A., Khoueiry, Z., Cassan, C., et al. (2014). Trpm4 gene invalidation leads to cardiac hypertrophy and electrophysiological alterations. PLoS ONE 9:e115256. doi: 10.1371/journal.pone.0115256
Deng, L., Hu, S., Baydoun, A. R., Chen, J., Chen, X., and Cong, X. (2009). Aspirin induces apoptosis in mesenchymal stem cells requiring Wnt/β-catenin pathway. Cell Prolif. 42, 721–730. doi: 10.1111/j.1365-2184.2009.00639.x
Dong, F., Harvey, J., Finan, A., Weber, K., Agarwal, U., and Penn, M. S. (2012). Myocardial CXCR4 expression is required for mesenchymal stem cell mediated repair following acute myocardial infarction. Circulation 126, 314–324. doi: 10.1161/CIRCULATIONAHA.111.082453
Durik, M., Sevá Pessôa, B., and Roks, A. J. M. (2012). The renin-angiotensin system, bone marrow and progenitor cells. Clin. Sci. Lond. Engl. 123, 205–223. doi: 10.1042/cs20110660
Ellison, G. M., Torella, D., Karakikes, I., Purushothaman, S., Curcio, A., Gasparri, C., et al. (2007). Acute β-adrenergic overload produces myocyte damage through calcium leakage from the ryanodine receptor 2 but spares cardiac stem cells. J. Biol. Chem. 282, 11397–11409. doi: 10.1074/jbc.M607391200
Ellison, G. M., Vicinanza, C., Smith, A. J., Aquila, I., Leone, A., Waring, C. D., et al. (2013). Adult c-kitpos cardiac stem cells are necessary and sufficient for functional cardiac regeneration and repair. Cell 154, 827–842. doi: 10.1016/j.cell.2013.07.039
Erbs, S., Beck, E. B., Linke, A., Adams, V., Gielen, S., Kränkel, N., et al. (2011). High-dose rosuvastatin in chronic heart failure promotes vasculogenesis, corrects endothelial function, and improves cardiac remodeling—Results from a randomized, double-blind, and placebo-controlled study. Int. J. Cardiol. 146, 56–63. doi: 10.1016/j.ijcard.2010.02.019
Eulalio, A., Mano, M., Ferro, M. D., Zentilin, L., Sinagra, G., Zacchigna, S., et al. (2012). Functional screening identifies miRNAs inducing cardiac regeneration. Nature 492, 376–381. doi: 10.1038/nature11739
Favaloro, L., Diez, M., Mendiz, O., Janavel, G. V., Valdivieso, L., Ratto, R., et al. (2013). High-dose plasmid-mediated VEGF gene transfer is safe in patients with severe ischemic heart disease (Genesis-I). A phase I, open-label, two-year follow-up trial. Catheter. Cardiovasc. Interv. 82, 899–906. doi: 10.1002/ccd.24555
Fazel, S. (2006). Cardioprotective c-kit+ cells are from the bone marrow and regulate the myocardial balance of angiogenic cytokines. J. Clin. Invest. 116, 1865–1877. doi: 10.1172/JCI27019
Ferreira-Martins, J., Rondon-Clavo, C., Tugal, D., Korn, J. A., Rizzi, R., Padin-Iruegas, M. E., et al. (2009). Spontaneous calcium oscillations regulate human cardiac progenitor cell growth. Circ. Res. 105, 764–774. doi: 10.1161/CIRCRESAHA.109.206698
Finan, A., Kiedrowski, M., Turturice, B. A., Sopko, N. A., and Penn, M. S. (2012). Cardiac pressure overload initiates a systemic stem cell response. Cytotherapy 14, 983–993. doi: 10.3109/14653249.2012.684380
Finan, A., Sopko, N., Dong, F., Turturice, B., Kiedrowski, M., and Penn, M. S. (2013). Bone marrow SSEA1+ cells support the myocardium in cardiac pressure overload. PLoS ONE 8:e68528. doi: 10.1371/journal.pone.0068528
Fischer, K. M., Cottage, C. T., Wu, W., Din, S., Gude, N. A., Avitabile, D., et al. (2009). Enhancement of myocardial regeneration through genetic engineering of cardiac progenitor cells expressing pim-1 kinase. Circulation 120, 2077–2087. doi: 10.1161/CIRCULATIONAHA.109.884403
Fransioli, J., Bailey, B., Gude, N. A., Cottage, C. T., Muraski, J. A., Emmanuel, G., et al. (2008). Evolution of the c-kit-positive cell response to pathological challenge in the myocardium. Stem Cells Dayt. Ohio 26, 1315–1324. doi: 10.1634/stemcells.2007-0751
Gambini, E., Pesce, M., Persico, L., Bassetti, B., Gambini, A., Alamanni, F., et al. (2012). Patient profile modulates cardiac c-kit(+) progenitor cell availability and amplification potential. Transl. Res. J. Lab. Clin. Med. 160, 363–373. doi: 10.1016/j.trsl.2012.05.009
Gautam, M., Fujita, D., Kimura, K., Ichikawa, H., Izawa, A., Hirose, M., et al. (2015). Transplantation of adipose tissue-derived stem cells improves cardiac contractile function and electrical stability in a rat myocardial infarction model. J. Mol. Cell. Cardiol. 81, 139–149. doi: 10.1016/j.yjmcc.2015.02.012
Genead, R., Danielsson, C., Andersson, A. B., Corbascio, M., Franco-Cereceda, A., Sylvén, C., et al. (2010). Islet-1 cells are cardiac progenitors present during the entire lifespan: from the embryonic stage to adulthood. Stem Cells Dev. 19, 1601–1615. doi: 10.1089/scd.2009.0483
Go, A. S., Mozaffarian, D., Roger, V. L., Benjamin, E. J., Berry, J. D., Blaha, M. J., et al. (2014). Heart disease and stroke statistics—2014 update a report from the American heart association. Circulation 129, e28–e292. doi: 10.1161/01.cir.0000442015.53336.12
Golpanian, S., El-Khorazaty, J., Mendizabal, A., DiFede, D. L., Suncion, V. Y., Karantalis, V., et al. (2015). Effect of aging on human mesenchymal stem cell therapy in ischemic cardiomyopathy patients. J. Am. Coll. Cardiol. 65, 125–132. doi: 10.1016/j.jacc.2014.10.040
Gong, Y., Zhao, Y., Li, Y., Fan, Y., and Hoover-Plow, J. (2014). Plasminogen regulates cardiac repair after myocardial infarction through its noncanonical function in stem cell homing to the infarcted heart. J. Am. Coll. Cardiol. 63, 2862–2872. doi: 10.1016/j.jacc.2013.11.070
Goumans, M.-J., Maring, J. A., and Smits, A. M. (2014). A straightforward guide to the basic science behind cardiovascular cell-based therapies. Heart 100, 1153–1157. doi: 10.1136/heartjnl-2014-305646
Gyöngyösi, M., Wojakowski, W., Lemarchand, P., Lunde, K., Tendera, M., Bartunek, J., et al. (2015). Meta-analysis of cell-based CaRdiac stUdiEs (ACCRUE) in patients with acute myocardial infarction based on individual patient data. Circ. Res. 116, 1346–1360. doi: 10.1161/CIRCRESAHA.116.304346
Hariharan, N., and Sussman, M. A. (2015). Cardiac aging—Getting to the stem of the problem. J. Mol. Cell. Cardiol. 83, 32–36. doi: 10.1016/j.yjmcc.2015.04.008
Hodgkinson, C. P., Kang, M. H., Dal-Pra, S., Mirotsou, M., and Dzau, V. J. (2015). MicroRNAs and cardiac regeneration. Circ. Res. 116, 1700–1711. doi: 10.1161/CIRCRESAHA.116.304377
Hsiao, L.-C., Perbellini, F., Gomes, R. S., Tan, J. J., Vieira, S., Faggian, G., et al. (2014). Murine cardiosphere-derived cells are impaired by age but not by cardiac dystrophic dysfunction. Stem Cells Dev. 23, 1027–1036. doi: 10.1089/scd.2013.0388
Hsieh, P. C. H., Segers, V. F. M., Davis, M. E., MacGillivray, C., Gannon, J., Molkentin, J. D., et al. (2007). Evidence from a genetic fate-mapping study that stem cells refresh adult mammalian cardiomyocytes after injury. Nat. Med. 13, 970–974. doi: 10.1038/nm1618
Huang, B., Qian, J., Ma, J., Huang, Z., Shen, Y., Chen, X., et al. (2014). Myocardial transfection of hypoxia-inducible factor-1α and co-transplantation of mesenchymal stem cells enhance cardiac repair in rats with experimental myocardial infarction. Stem Cell Res. Ther. 5, 22. doi: 10.1186/scrt410
Huang, M., Nguyen, P., Jia, F., Hu, S., Gong, Y., de Almeida, P. E., et al. (2011). Double knockdown of prolyl hydroxylase and factor-inhibiting hypoxia-inducible factor with nonviral minicircle gene therapy enhances stem cell mobilization and angiogenesis after myocardial infarction. Circulation 124, S46–S54. doi: 10.1161/CIRCULATIONAHA.110.014019
Inagawa, K., Miyamoto, K., Yamakawa, H., Muraoka, N., Sadahiro, T., Umei, T., et al. (2012). Induction of cardiomyocyte-like cells in infarct hearts by gene transfer of Gata4, Mef2c, and Tbx5. Circ. Res. 111, 1147–1156. doi: 10.1161/CIRCRESAHA.112.271148
Ishii, M., Shibata, R., Shimizu, Y., Yamamoto, T., Kondo, K., Inoue, Y., et al. (2014). Multilayered adipose-derived regenerative cell sheets created by a novel magnetite tissue engineering method for myocardial infarction. Int. J. Cardiol. 175, 545–553. doi: 10.1016/j.ijcard.2014.06.034
Ishikawa, K., Fish, K., Aguero, J., Yaniz-Galende, E., Jeong, D., Kho, C., et al. (2015). Stem cell factor gene transfer improves cardiac function after myocardial infarction in swine. Circ. Heart Fail. 8, 167–174. doi: 10.1161/CIRCHEARTFAILURE.114.001711
Ito, M., Adachi, T., Pimentel, D. R., Ido, Y., and Colucci, W. S. (2004). Statins inhibit β-Adrenergic receptor–stimulated apoptosis in adult rat ventricular myocytes via a Rac1-dependent mechanism. Circulation 110, 412–418. doi: 10.1161/01.CIR.0000136088.18960.E6
Iwasaki, H., Kawamoto, A., Tjwa, M., Horii, M., Hayashi, S., Oyamada, A., et al. (2011). PlGF repairs myocardial ischemia through mechanisms of angiogenesis, cardioprotection and recruitment of myo-angiogenic competent marrow progenitors. PLoS ONE 6:e24872. doi: 10.1371/journal.pone.0024872
Jayawardena, T. M., Finch, E. A., Zhang, L., Zhang, H., Hodgkinson, C. P., Pratt, R. E., et al. (2015). MicroRNA induced cardiac reprogramming in vivo evidence for mature cardiac myocytes and improved cardiac function. Circ. Res. 116, 418–424. doi: 10.1161/CIRCRESAHA.116.304510
Jesty, S. A., Steffey, M. A., Lee, F. K., Breitbach, M., Hesse, M., Reining, S., et al. (2012). c-kit+ precursors support postinfarction myogenesis in the neonatal, but not adult, heart. Proc. Natl. Acad. Sci. U.S.A. 109, 13380–13385. doi: 10.1073/pnas.1208114109
Jopling, C., Sleep, E., Raya, M., Martí, M., Raya, A., and Belmonte, J. C. I. (2010). Zebrafish heart regeneration occurs by cardiomyocyte dedifferentiation and proliferation. Nature 464, 606–609. doi: 10.1038/nature08899
Jung, C., Florvaag, A., Oberle, V., Fritzenwanger, M., Kretschmar, D., Kuethe, F., et al. (2012). Positive effect of eplerenone treatment on endothelial progenitor cells in patients with chronic heart failure. J. Renin Angiotensin Aldosterone Syst. 13, 401–406. doi: 10.1177/1470320312447650
Kajstura, J., Cigola, E., Malhotra, A., Li, P., Cheng, W., Meggs, L. G., et al. (1997). Angiotensin II induces apoptosis of adult ventricular myocytes in vitro. J. Mol. Cell. Cardiol. 29, 859–870. doi: 10.1006/jmcc.1996.0333
Kajstura, J., Leri, A., Finato, N., Di Loreto, C., Beltrami, C. A., and Anversa, P. (1998). Myocyte proliferation in end-stage cardiac failure in humans. Proc. Natl. Acad. Sci. U.S.A. 95, 8801–8805. doi: 10.1073/pnas.95.15.8801
Kajstura, J., Urbanek, K., Perl, S., Hosoda, T., Zheng, H., Ogórek, B., et al. (2010). Cardiomyogenesis in the adult human heart. Circ. Res. 107, 305–315. doi: 10.1161/CIRCRESAHA.110.223024
Kanashiro-Takeuchi, R. M., Schulman, I. H., and Hare, J. M. (2011). Pharmacologic and genetic strategies to enhance cell therapy for cardiac regeneration. J. Mol. Cell. Cardiol. 51, 619–625. doi: 10.1016/j.yjmcc.2011.05.015
Katz, M. G., Fargnoli, A. S., Williams, R. D., Steuerwald, N. M., Isidro, A., Ivanina, A. V., et al. (2014). Safety and efficacy of high-dose adeno-associated virus 9 encoding sarcoplasmic reticulum Ca2+ adenosine triphosphatase delivered by molecular cardiac surgery with recirculating delivery in ovine ischemic cardiomyopathy. J. Thorac. Cardiovasc. Surg. 148, 1065–1073.e2. doi: 10.1016/j.jtcvs.2014.05.070
Khan, M., Mohsin, S., Avitabile, D., Siddiqi, S., Nguyen, J., Wallach, K., et al. (2013). β-Adrenergic regulation of cardiac progenitor cell death versus survival and proliferation. Circ. Res. 112, 476–486. doi: 10.1161/CIRCRESAHA.112.280735
Khan, M., Nickoloff, E., Abramova, T., Johnson, J., Verma, S. K., Krishnamurthy, P., et al. (2015). Embryonic stem cell-derived exosomes promote endogenous repair mechanisms and enhance cardiac function following myocardial infarction. Circ. Res. 115:305990. doi: 10.1161/CIRCRESAHA.117.305990
Kikuchi, K., Holdway, J. E., Werdich, A. A., Anderson, R. M., Fang, Y., Egnaczyk, G. F., et al. (2010). Primary contribution to zebrafish heart regeneration by gata4+ cardiomyocytes. Nature 464, 601–605. doi: 10.1038/nature08804
Kim, S.-W., Houge, M., Brown, M., Davis, M. E., and Yoon, Y. (2014). Cultured human bone marrow–derived CD31+ cells are effective for cardiac and vascular repair through enhanced angiogenic, adhesion, and anti-inflammatory effects. J. Am. Coll. Cardiol. 64, 1681–1694. doi: 10.1016/j.jacc.2014.06.1204
Kucia, M., Reca, R., Campbell, F. R., Zuba-Surma, E., Majka, M., Ratajczak, J., et al. (2006). A population of very small embryonic-like (VSEL) CXCR4+SSEA-1+Oct-4+ stem cells identified in adult bone marrow. Leukemia 20, 857–869. doi: 10.1038/sj.leu.2404171
Laflamme, M. A., Chen, K. Y., Naumova, A. V., Muskheli, V., Fugate, J. A., Dupras, S. K., et al. (2007). Cardiomyocytes derived from human embryonic stem cells in pro-survival factors enhance function of infarcted rat hearts. Nat. Biotechnol. 25, 1015–1024. doi: 10.1038/nbt1327
Laflamme, M. A., and Murry, C. E. (2011). Heart regeneration. Nature 473, 326–335. doi: 10.1038/nature10147
Lalit, P. A., Hei, D. J., Raval, A. N., and Kamp, T. J. (2014). Induced pluripotent stem cells for post-myocardial infarction repair: remarkable opportunities and challenges. Circ. Res. 114, 1328–1345. doi: 10.1161/CIRCRESAHA.114.300556
Lazar, L. D., Pletcher, M. J., Coxson, P. G., Bibbins-Domingo, K., and Goldman, L. (2011). Cost-effectiveness of statin therapy for primary prevention in a low-cost statin Era. Circulation 124, 146–153. doi: 10.1161/CIRCULATIONAHA.110.986349
Lee, P. S. S., and Poh, K. K. (2014). Endothelial progenitor cells in cardiovascular diseases. World J. Stem Cells 6, 355–366. doi: 10.4252/wjsc.v6.i3.355
Leja, M. J., Shah, D. J., and Reardon, M. J. (2011). Primary cardiac tumors. Tex. Heart Inst. J. 38, 261–262.
Li, L., Zeng, H., and Chen, J.-X. (2012). Apelin-13 increases myocardial progenitor cells and improves repair postmyocardial infarction. Am. J. Physiol. Heart Circ. Physiol. 303, H605–H618. doi: 10.1152/ajpheart.00366.2012
Lin, Z., von Gise, A., Zhou, P., Gu, F., Ma, Q., Jiang, J., et al. (2014). Cardiac-specific YAP activation improves cardiac function and survival in an experimental murine MI model. Circ. Res. 115, 354–363. doi: 10.1161/CIRCRESAHA.115.303632
Liu, C., Fan, Y., Zhou, L., Zhu, H.-Y., Song, Y.-C., Hu, L., et al. (2015). Pretreatment of mesenchymal stem cells with angiotensin II enhances paracrine effects, angiogenesis, gap junction formation and therapeutic efficacy for myocardial infarction. Int. J. Cardiol. 188, 22–32. doi: 10.1016/j.ijcard.2015.03.425
Loffredo, F. S., Steinhauser, M. L., Gannon, J., and Lee, R. T. (2011). Bone marrow-derived cell therapy stimulates endogenous cardiomyocyte progenitors and promotes cardiac repair. Cell Stem Cell 8, 389–398. doi: 10.1016/j.stem.2011.02.002
Mahmoud, A. I., Kocabas, F., Muralidhar, S. A., Kimura, W., Koura, A. S., Thet, S., et al. (2013). Meis1 regulates postnatal cardiomyocyte cell cycle arrest. Nature 497, 249–253. doi: 10.1038/nature12054
Malecki, M., Sabo, C., Putzer, E., Stampe, C., Foorohar, A., Quach, C., et al. (2013). Recruitment and retention of human autologous CD34+ CD117+ CD133+ bone marrow stem cells to infarcted myocardium followed by directed vasculogenesis: novel strategy for cardiac regeneration. Mol. Cell. Ther. 1:4. doi: 10.1186/2052-8426-1-4
Malliaras, K., Ibrahim, A., Tseliou, E., Liu, W., Sun, B., Middleton, R. C., et al. (2014a). Stimulation of endogenous cardioblasts by exogenous cell therapy after myocardial infarction. EMBO Mol. Med. 6, 760–777. doi: 10.1002/emmm.201303626
Malliaras, K., Makkar, R. R., Smith, R. R., Cheng, K., Wu, E., Bonow, R. O., et al. (2014b). Intracoronary cardiosphere-derived cells after myocardial infarction. J. Am. Coll. Cardiol. 63, 110–122. doi: 10.1016/j.jacc.2013.08.724
Mathiasen, A. B., Qayyum, A. A., Jørgensen, E., Helqvist, S., Fischer-Nielsen, A., Kofoed, K. F., et al. (2015). Bone marrow-derived mesenchymal stromal cell treatment in patients with severe ischaemic heart failure: a randomized placebo-controlled trial (MSC-HF trial). Eur. Heart J. 36, 1744–1753. doi: 10.1093/ eurheartj/ehv136
Mentz, R. J., Bakris, G. L., Waeber, B., McMurray, J. J. V., Gheorghiade, M., Ruilope, L. M., et al. (2013). The past, present and future of renin–angiotensin aldosterone system inhibition. Int. J. Cardiol. 167, 1677–1687. doi: 10.1016/j.ijcard.2012.10.007
Mirantes, C., Passegué, E., and Pietras, E. M. (2014). Pro-inflammatory cytokines: emerging players regulating HSC function in normal and diseased hematopoiesis. Exp. Cell Res. 329, 248–254. doi: 10.1016/j.yexcr.2014.08.017
Mollova, M., Bersell, K., Walsh, S., Savla, J., Das, L. T., Park, S.-Y., et al. (2013). Cardiomyocyte proliferation contributes to heart growth in young humans. Proc. Natl. Acad. Sci. U.S.A. 110, 1446–1451. doi: 10.1073/pnas.1214608110
Mouquet, F., Pfister, O., Jain, M., Oikonomopoulos, A., Ngoy, S., Summer, R., et al. (2005). Restoration of cardiac progenitor cells after myocardial infarction by self-proliferation and selective homing of bone marrow-derived stem cells. Circ. Res. 97, 1090–1092. doi: 10.1161/01.RES.0000194330.66545.f5
Musumeci, M., Maccari, S., Sestili, P., Signore, M., Molinari, P., Ambrosio, C., et al. (2011). Propranolol enhances cell cycle-related gene expression in pressure overloaded hearts. Br. J. Pharmacol. 164, 1917–1928. doi: 10.1111/j.1476-5381.2011.01504.x
Nagai, T., and Komuro, I. (2012). Gene and cytokine therapy for heart failure: molecular mechanisms in the improvement of cardiac function. Am. J. Physiol. Heart Circ. Physiol. 303, H501–H512. doi: 10.1152/ajpheart.00130.2012
Naqvi, N., Li, M., Calvert, J. W., Tejada, T., Lambert, J. P., Wu, J., et al. (2014). A proliferative burst during preadolescence establishes the final cardiomyocyte number. Cell 157, 795–807. doi: 10.1016/j.cell.2014.03.035
Ong, S.-G., Lee, W. H., Huang, M., Dey, D., Kodo, K., Sanchez-Freire, V., et al. (2014). Cross talk of combined gene and cell therapy in ischemic heart disease role of exosomal MicroRNA transfer. Circulation 130, S60–S69. doi: 10.1161/CIRCULATIONAHA.113.007917
Pavo, N., Charwat, S., Nyolczas, N., Jakab, A., Murlasits, Z., Bergler-Klein, J., et al. (2014). Cell therapy for human ischemic heart diseases: critical review and summary of the clinical experiences. J. Mol. Cell. Cardiol. 75, 12–24. doi: 10.1016/j.yjmcc.2014.06.016
Penn, M. S. (2010). SDF-1:CXCR4 axis is fundamental for tissue preservation and repair. Am. J. Pathol. 177, 2166–2168. doi: 10.2353/ajpath.2010.100803
Penn, M. S., Mendelsohn, F. O., Schaer, G. L., Sherman, W., Farr, M., Pastore, J., et al. (2013). An open-label dose escalation study to evaluate the safety of administration of nonviral stromal cell-derived factor-1 plasmid to treat symptomatic ischemic heart failure. Circ. Res. 112, 816–825. doi: 10.1161/CIRCRESAHA.111.300440
Porrello, E. R., Mahmoud, A. I., Simpson, E., Hill, J. A., Richardson, J. A., Olson, E. N., et al. (2011). Transient regenerative potential of the neonatal mouse heart. Science 331, 1078–1080. doi: 10.1126/science.1200708
Poss, K. D., Wilson, L. G., and Keating, M. T. (2002). Heart regeneration in zebrafish. Science 298, 2188–2190. doi: 10.1126/science.1077857
Qian, L., Huang, Y., Spencer, C. I., Foley, A., Vedantham, V., Liu, L., et al. (2012). In vivo reprogramming of murine cardiac fibroblasts into induced cardiomyocytes. Nature 485, 593–598. doi: 10.1038/nature11044
Quaini, F., Urbanek, K., Beltrami, A. P., Finato, N., Beltrami, C. A., Nadal-Ginard, B., et al. (2002). Chimerism of the transplanted heart. N. Engl. J. Med. 346, 5–15. doi: 10.1056/NEJMoa012081
Reber, L., Da Silva, C. A., and Frossard, N. (2006). Stem cell factor and its receptor c-Kit as targets for inflammatory diseases. Eur. J. Pharmacol. 533, 327–340. doi: 10.1016/j.ejphar.2005.12.067
Roche, E. T., Hastings, C. L., Lewin, S. A., Shvartsman, D. E., Brudno, Y., Vasilyev, N. V., et al. (2014). Comparison of biomaterial delivery vehicles for improving acute retention of stem cells in the infarcted heart. Biomaterials 35, 6850–6858. doi: 10.1016/j.biomaterials.2014.04.114
Rumyantsev, P. P., and Borisov, A. (1987). DNA synthesis in myocytes from different myocardial compartments of young rats in norm, after experimental infarction and in vitro. Biomed. Biochim. Acta 46, S610–615.
Sato, T., Amano, H., Ito, Y., Eshima, K., Minamino, T., Ae, T., et al. (2013). NSAID, aspirin delays gastric ulcer healing with reduced accumulation of CXCR4+VEGFR1+ cells to the ulcer granulation tissues. Biomed. Pharmacother. 67, 607–613. doi: 10.1016/j.biopha.2013.01.009
Schenk, S., Mal, N., Finan, A., Zhang, M., Kiedrowski, M., Popovic, Z., et al. (2007). Monocyte chemotactic protein-3 is a myocardial mesenchymal stem cell homing factor. Stem Cells Dayt. Ohio 25, 245–251. doi: 10.1634/stemcells.2006-0293
Scimia, M. C., Gumpert, A. M., and Koch, W. J. (2013). Cardiovascular gene therapy for myocardial infarction. Expert Opin. Biol. Ther. 14, 183–195. doi: 10.1517/14712598.2014.866085
Semenza, G. L. (2014). Hypoxia-inducible factor 1 and cardiovascular disease. Annu. Rev. Physiol. 76, 39–56. doi: 10.1146/annurev-physiol-021113-170322
Senyo, S. E., Steinhauser, M. L., Pizzimenti, C. L., Yang, V. K., Cai, L., Wang, M., et al. (2013). Mammalian heart renewal by preexisting cardiomyocytes. Nature 493, 433–436. doi: 10.1038/nature11682
Seqqat, R., Guo, X., Rafiq, K., Kolpakov, M. A., Guo, J., Koch, W. J., et al. (2012). Beta1-adrenergic receptors promote focal adhesion signaling downregulation and myocyte apoptosis in acute volume overload. J. Mol. Cell. Cardiol. 53, 240–249. doi: 10.1016/j.yjmcc.2012.05.004
Shaw, S. Y., Cheng, S., Cupples, L. A., Larson, M. G., McCabe, E. L., Ngwa, J. S., et al. (2011). Genetic and clinical correlates of early-outgrowth colony-forming units. Circ. Cardiovasc. Genet. 4, 296–304. doi: 10.1161/CIRCGENETICS.110.958470
Shiba, Y., Fernandes, S., Zhu, W.-Z., Filice, D., Muskheli, V., Kim, J., et al. (2012). Human ES-cell-derived cardiomyocytes electrically couple and suppress arrhythmias in injured hearts. Nature 489, 322–325. doi: 10.1038/nature11317
Sirish, P., López, J. E., Li, N., Wong, A., Timofeyev, V., Young, J. N., et al. (2012). MicroRNA profiling predicts a variance in the proliferative potential of cardiac progenitor cells derived from neonatal and adult murine hearts. J. Mol. Cell. Cardiol. 52, 264–272. doi: 10.1016/j.yjmcc.2011.10.012
Smart, N., Bollini, S., Dub,é, K. N., Vieira, J. M., Zhou, B., Davidson, S., et al. (2011). De novo cardiomyocytes from within the activated adult heart after injury. Nature 474, 640–644. doi: 10.1038/nature10188
Sopko, N. A., Turturice, B. A., Becker, M. E., Brown, C. R., Dong, F., Popoviæ, Z. B., et al. (2010). Bone marrow support of the heart in pressure overload is lost with aging. PLoS ONE 5:e15187. doi: 10.1371/journal.pone.0015187
Sorrentino, S. A., Doerries, C., Manes, C., Speer, T., Dessy, C., Lobysheva, I., et al. (2011). Nebivolol exerts beneficial effects on endothelial function, early endothelial progenitor cells, myocardial neovascularization, and left ventricular dysfunction early after myocardial infarction beyond conventional β1-blockade. J. Am. Coll. Cardiol. 57, 601–611. doi: 10.1016/j.jacc.2010.09.037
Sun, Y., Wang, Y., Zhang, L., Xu, C., Liu, Y., Kang, S., et al. (2014). Prevention of cardiac events caused by surgical stress in aged rats: simultaneously activating β2-adrenoceptor and inhibiting β1-adrenoceptor. Stress 17, 373–381. doi: 10.3109/10253890.2014.915392
Suzuki, G., Iyer, V., Cimato, T., and Canty, J. M. (2009). Pravastatin improves function in hibernating myocardium by mobilizing CD133+ and cKit+ bone marrow progenitor cells and promoting myocytes to reenter the growth phase of the cardiac cell cycle. Circ. Res. 104, 255–264. doi: 10.1161/CIRCRESAHA.108.188730
Tan, C., Chen, W., Wu, Y., Lin, J., Lin, R., Tan, X., et al. (2013). Chronic aspirin via dose-dependent and selective inhibition of cardiac proteasome possibly contributed a potential risk to the ischemic heart. Exp. Gerontol. 48, 812–823. doi: 10.1016/j.exger.2013.03.012
Tanaka, T. D., Lancaster, J. J., Juneman, E., Bahl, J. J., and Goldman, S. (2013). Clenbuterol plus granulocyte colony-stimulating factor regulates stem/progenitor cell mobilization and exerts beneficial effect by increasing neovascularization in rats with heart failure. J. Card. Fail. 19, 503–508. doi: 10.1016/j.cardfail.2013.05.010
Tang, X.-L., Sanganalmath, S. K., Sato, H., Bi, Q., Hunt, G., Vincent, R. J., et al. (2011). Atorvastatin Therapy during the Peri-Infarct Period Attenuates Left Ventricular Dysfunction and Remodeling after Myocardial Infarction. PLoS ONE 6:e25320. doi: 10.1371/journal.pone.0025320
Tano, N., Narita, T., Kaneko, M., Ikebe, C., Coppen, S. R., Campbell, N. G., et al. (2014). Epicardial placement of mesenchymal stromal cell-sheets for the treatment of ischemic cardiomyopathy; in vivo proof-of-concept study. Mol. Ther. 22, 1864–1871. doi: 10.1038/mt.2014.110
Thum, T., Schmitter, K., Fleissner, F., Wiebking, V., Dietrich, B., Widder, J. D., et al. (2011). Impairment of endothelial progenitor cell function and vascularization capacity by aldosterone in mice and humans. Eur. Heart J. 32, 1275–1286. doi: 10.1093/eurheartj/ehq254
Uchida, S., De Gaspari, P., Kostin, S., Jenniches, K., Kilic, A., Izumiya, Y., et al. (2013). Sca1-derived cells are a source of myocardial renewal in the murine adult heart. Stem Cell Rep. 1, 397–410. doi: 10.1016/j.stemcr.2013.09.004
Urbanek, K., Quaini, F., Tasca, G., Torella, D., Castaldo, C., Nadal-Ginard, B., et al. (2003). Intense myocyte formation from cardiac stem cells in human cardiac hypertrophy. Proc. Natl. Acad. Sci. U.S.A. 100, 10440–10445. doi: 10.1073/pnas.1832855100
Van Berlo, J. H., Kanisicak, O., Maillet, M., Vagnozzi, R. J., Karch, J., Lin, S.-C. J., et al. (2014). c-kit+ cells minimally contribute cardiomyocytes to the heart. Nature 509, 337–341. doi: 10.1038/nature13309
Walsh, S., Pontén, A., Fleischmann, B. K., and Jovinge, S. (2010). Cardiomyocyte cell cycle control and growth estimation in vivo—an analysis based on cardiomyocyte nuclei. Cardiovasc. Res. 86, 365–373. doi: 10.1093/cvr/cvq005
Wu, J. M. F., Hsueh, Y.-C., Ch'ang, H.-J., Luo, C.-Y., Wu, L.-W., Nakauchi, H., et al. (2015). Circulating cells contribute to cardiomyocyte regeneration after injury. Circ. Res. 116, 633–641. doi: 10.1161/CIRCRESAHA.116.304564
Wu, L., Jia, Z., Yan, L., Wang, W., Wang, J., Zhang, Y., et al. (2013). Angiotensin II promotes cardiac differentiation of embryonic stem cells via angiotensin type 1 receptor. Differentiation 86, 23–29. doi: 10.1016/j.diff.2013.06.007
Xu, J., Carretero, O. A., Liao, T.-D., Peng, H., Shesely, E. G., Xu, J., et al. (2010). Local angiotensin II aggravates cardiac remodeling in hypertension. Am. J. Physiol. Heart Circ. Physiol. 299, H1328–1338. doi: 10.1152/ajpheart.00538.2010
Yan, L., Jia, Z., Cui, J., Yang, H., Yang, H., Zhang, Y., et al. (2011). Beta-adrenergic signals regulate cardiac differentiation of mouse embryonic stem cells via mitogen-activated protein kinase pathways. Dev. Growth Differ. 53, 772–779. doi: 10.1111/j.1440-169X.2011.01284.x
Yaniz-Galende, E., Chen, J., Chemaly, E., Liang, L., Hulot, J.-S., McCollum, L., et al. (2012). Stem cell factor gene transfer promotes cardiac repair after myocardial infarction via in situ recruitment and expansion of c-kit+ cells. Circ. Res. 111, 1434–1445. doi: 10.1161/CIRCRESAHA.111.263830
Yoon, C.-H., Koyanagi, M., Iekushi, K., Seeger, F., Urbich, C., Zeiher, A. M., et al. (2010). Mechanism of improved cardiac function after bone marrow mononuclear cell therapy role of cardiovascular lineage commitment. Circulation 121, 2001–2011. doi: 10.1161/CIRCULATIONAHA.109.909291
Zangi, L., Lui, K. O., von Gise, A., Ma, Q., Ebina, W., Ptaszek, L. M., et al. (2013). Modified mRNA directs the fate of heart progenitor cells and induces vascular regeneration after myocardial infarction. Nat. Biotechnol. 31, 898–907. doi: 10.1038/nbt.2682
Zhang, L., Guo, J., Zhang, P., Xiong, Q., Wu, S. C., Xia, L., et al. (2015). Derivation and high engraftment of patient-specific cardiomyocyte sheet using induced pluripotent stem cells generated from adult cardiac fibroblast. Circ. Heart Fail. 8, 156–166. doi: 10.1161/CIRCHEARTFAILURE.114.001317
Zhang, M., Mal, N., Kiedrowski, M., Chacko, M., Askari, A. T., Popovic, Z. B., et al. (2007). SDF-1 expression by mesenchymal stem cells results in trophic support of cardiac myocytes after myocardial infarction. FASEB J. Off. Publ. Fed. Am. Soc. Exp. Biol. 21, 3197–3207. doi: 10.1096/fj.06-6558com
Zhang, Q., Xiang, J., Wang, X., Liu, H., Hu, B., Feng, M., et al. (2010). β2-adrenoceptor agonist clenbuterol reduces infarct size and myocardial apoptosis after myocardial ischaemia/reperfusion in anaesthetized rats. Br. J. Pharmacol. 160, 1561–1572. doi: 10.1111/j.1476-5381.2010.00813.x
Keywords: cell therapy, cardiac progenitor cells, regeneration, gene therapy, stem cells, beta blockers, statins
Citation: Finan A and Richard S (2015) Stimulating endogenous cardiac repair. Front. Cell Dev. Biol. 3:57. doi: 10.3389/fcell.2015.00057
Received: 24 May 2015; Accepted: 08 September 2015;
Published: 29 September 2015.
Edited by:
Cedric Viero, Saarland University Medical Faculty, GermanyReviewed by:
Tomo Saric, University of Cologne, GermanyJohannes Boltze, Fraunhofer-Society, Germany
Copyright © 2015 Finan and Richard. This is an open-access article distributed under the terms of the Creative Commons Attribution License (CC BY). The use, distribution or reproduction in other forums is permitted, provided the original author(s) or licensor are credited and that the original publication in this journal is cited, in accordance with accepted academic practice. No use, distribution or reproduction is permitted which does not comply with these terms.
*Correspondence: Sylvain Richard, Centre National de la Recherche Scientifique United Medical Resource 9214, Institut National de la Santé et de la Recherche Médicale U1046, Physiology and Experimental Medicine of the Heart and Muscles, University of Montpellier, CHU Arnaud de Villeneuve, 371 Avenue de Doyen Gaston Giraud, 34295 Montpellier, France, sylvain.richard@inserm.fr