Common processes at unique volcanoes—a volcanological conundrum
- School of Earth Sciences, University of Bristol, Bristol, UK
An emerging challenge in modern volcanology is the apparent contradiction between the perception that every volcano is unique, and classification systems based on commonalities among volcano morphology and eruptive style. On the one hand, detailed studies of individual volcanoes show that a single volcano often exhibits similar patterns of behavior over multiple eruptive episodes; this observation has led to the idea that each volcano has its own distinctive pattern of behavior (or “personality”). In contrast, volcano classification schemes define eruption “styles” referenced to “type” volcanoes (e.g., Plinian, Strombolian, Vulcanian); this approach implicitly assumes that common processes underpin volcanic activity and can be used to predict the nature, extent and ensuing hazards of individual volcanoes. Actual volcanic eruptions, however, often include multiple styles, and type volcanoes may experience atypical eruptions (e.g., violent explosive eruptions of Kilauea, Hawaii, Fiske et al., 2009). The volcanological community is thus left with a fundamental conundrum that pits the uniqueness of individual volcanic systems against generalization of common processes. Addressing this challenge represents a major challenge to volcano research.
Classification of volcanic eruptions was pioneered by early volcanologists such as Mercalli (1907), who used observations of active volcanoes to define different explosion types. Critically, Mercalli used the nature of the eruptive products—fluid or solid—as a primary criterion for classification. On this basis he classified Strombolian and Hawaiian explosions, with their distinctively incandescent, and fluidal pyroclasts (and “red” eruption columns), as different from Vulcanian or Plinian eruptions, which were not only more violent but also produced solid pyroclasts and “gray” eruptive columns. Identification of these four eruptive “styles” comes directly from observations of activity at the “type” volcanoes Stromboli, Kilauea and Mauna Loa (Hawaii), Vulcano and Vesuvius (specifically, Pliny's account of the 79 AD eruption). These names for eruption styles have become embedded within the volcanological literature; recognition that eruption style, volcano morphology, and magma composition were linked provided the underpinning for a general classification of volcanological processes and products. At the same time, however, detailed observations of highly variable activity at both Stromboli and Vesuvius led Mercalli to caution that “le esplosioni presentano tutti I gradi dal debole al parossismico” [explosions have all gradations between weak and paroxysmal]. This work, as well as detailed descriptions of larger volcanic eruptions [e.g., Krakatau, Indonesia in 1883 (Simkin and Fiske, 1983); Mont Pelee, Martinique, 1902 (Lacroix, 1904)] provided ample evidence of the wide range in eruptive styles evidenced at even a single volcano, and initiated the modern era of volcano monitoring.
Recent dramatic advances in Volcanology as a science have been stimulated by both new technologies and well observed eruptions that have provided opportunities for multidisciplinary case studies. Doubtless major breakthroughs have resulted from studying individual systems in great detail; examples include the role of decompression-driven crystallization in arc volcanoes such as Mount Saint Helens (Cashman and Blundy, 2000) and the origin of rapid transitions from effusive to explosive activity that typify dome-forming events such as Soufriere Hills, Montserrat (Sparks, 1997). Moreover, opportunities for further, eruption-specific advances in understanding individual systems will continue to be provided by systematic volcano monitoring: currently, the World Organization of Volcano Observatories (WOVO) has 80 members and even volcanoes in remote and inhospitable environments can be monitored by satellite-based remote sensing techniques. For this reason, we, as a community, no longer have to rely on a handful of observed volcanic systems, but instead can compare and contrast measurements and processes from a wide range of eruption styles, magnitudes and settings. Both the richness and the heterogeneous nature of these data have, on the short term, served to emphasize the apparent uniqueness of well-monitored volcanoes. A critical challenge for the future is to use this wealth of information to examine possible commonalities in eruptive behavior, particularly in the context of global volcano hazard and risk assessment.
The question of uniqueness vs. commonality also raises philosophical issues, such as (1) the relative merits of studying a few individual systems very well as compared to many systems in moderate detail, (2) when (and how) the global dataset can be used to derive probabilities that are applicable to an individual system, (3) when (and how) results drawn from multi-disciplinary studies of an individual volcano can be generalized to infer global patterns of precursor and hazard scenarios, and (4) when (and how) experience from one volcanic crisis can be applied to another situation. The answers to these issues have strategic and social relevance. For example, the 2010 eruption of Eyjafjallajökull, Iceland, provided a graphic demonstration of the potential vulnerability of Europe to even small and distant volcanic eruptions (Stohl et al., 2011). Larger eruptions of the past few centuries [including the 1783–1784 Laki eruption in Iceland (Schmidt et al., 2011) and the 1815 explosive eruption of Tambora in Indonesia (Oppenheimer, 2003)] demonstrate the potential for protracted disturbances of regional (Laki) and global (Tambora) weather patterns caused by volcanic activity. It is thus worth asking to what extent accurate probabilistic models of hazard and risk assessment require detailed knowledge of each individual volcano (of the ~1500 in the Global Volcanism Program database1), or whether a handful of scenarios applied using a broad classification scheme is sufficient for global analysis.
To answer this question, it is important to assess the goals of volcano research. One goal is to improve the safety of communities that live close to active volcanoes. Here identifying one or more reliable precursors to an impending eruption [e.g., identification of tornillos at Galeras volcano, Columbia (Lourdes et al., 1997)] may not only be sufficient to meet that goal (that is, knowledge of the individual volcano may be enough), but may be the most appropriate and accurate approach. Individual volcano knowledge, in fact, underpins recent advances in developing effective strategies for volcano monitoring (Ewert et al., 2005; Miller and Jolly, 2014) and forecasting (Lindsay et al., 2010; Marzocchi and Bebbington, 2012; Bebbington, 2013) using statistical approaches such as risk trees and probabilistic models. A second goal is global, and focuses on acquisition of volcano parameters that can be used to identify regions of high volcanic hazard (and risk), and to place hazard assessments of specific volcanoes within a global context. For example, Figure 1 illustrates ways in which high-impact eruptions may stimulate advances in both research and infrastructure (solid arrows). To complete the circle, however, requires both effective transfer of information to other volcanic crises, and, ultimately, synthesis of this information into a global framework (dashed arrows). Databases being developed for this purpose can be found under the umbrella of the Global Volcano Model (GVM2), and include the Global Volcanism Program (GVP) and the Volcano Global Risk Identification and Analysis Project (VOGRIPA3), both of which compile information about volcano locations and eruptive histories, as well as the WOVO database (WOVOdat4), which aims to assemble monitoring data for volcanoes around the world. The utility of such a database is demonstrated by a recent study of volcanic unrest in the 21st century (Phillipson et al., 2013; Biggs et al., 2014), which examines both the duration and types of unrest that may precede eruptive activity in different tectonic settings. Implicit in construction of these databases is the assumption of universal (global) parameters that allow transferal of information from one volcano to another.
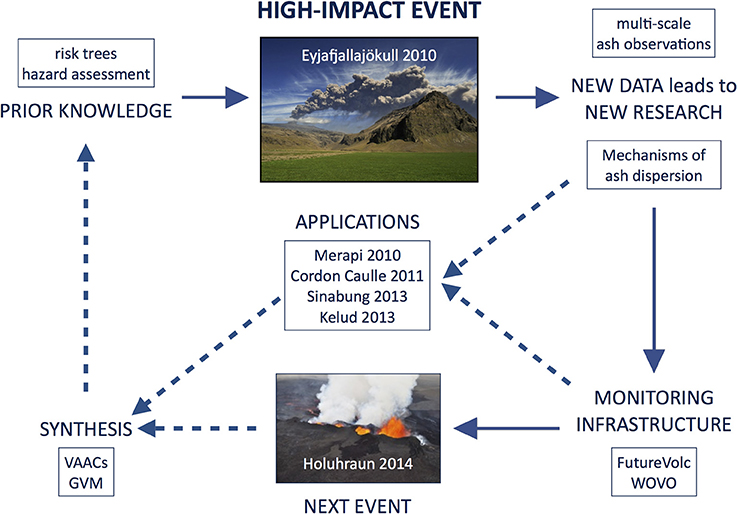
Figure 1. We illustrate both the power and limitations of focusing on high-impact events in the flow chart above, which uses as example two recent eruptions in Iceland. The high impact of the 2010 eruption of Eyjafjallajökull prompted an intense multi-disciplinary examination of ash generation, transport, deposition and impact (shown as the solid arrow to New Research). At the same time, resulting improvements to the monitoring capability in Iceland clearly enhanced the response to the on-going (non-explosive) Barğarbunga -Holuhraun event (solid arrow to monitoring infrastructure). Response to recent activity at other volcanoes in Indonesia and Chile has been influenced by the Eyjafjallajökull eruption, particularly with regard to civil aviation, but the information transfer is limited by differences in eruptive activity (shown as dashed arrows). The “synthesis” component is key to preparing for future eruptions and understanding the full spectrum of volcanic behavior, yet it is unclear, to what extent lessons learned from a specific event can (or should) be transferred directly to other, less intensively studied systems (shown as dashed arrows). This is of particular concern with regard to hazard assessment and risk tree development.
The most commonly used parameter to classify eruptions is the Volcano Explosivity Index (VEI, Newhall and Self, 1982), although there is ambiguity inherent in assigning VEI, which may use either plume height (assumed to be related to eruption intensity) or erupted volume (or magnitude, when determined by mass). More explicit is use of magnitude alone, which, together with the duration, defines the average eruption intensity (Pyle, 2000). Not easily encoded in current databases, however, are the details of eruptive events, including changes in eruptive style (e.g., from explosive to effusive, or from steady to pulsatory) that are the usual consequence of fluctuating eruption rates. This leads to the third goal of volcano studies, which is to identify the underlying principles of how volcanoes work. Although in the broadest sense the rationale for this work is the hope of defining parameters that can be used to forecast, or even predict, future activity, the complexity of volcanic systems means that we are still far from this goal. It is within this arena, however, that the fundamental conundrum presented above needs to be addressed.
The Global Volcanism program currently lists 1559 volcanoes that have erupted during the Holocene period. When viewed from the perspective of global monitoring, this seems an overwhelming target, but when considered from the perspective of sampling a complex system it is worryingly small. In some ways, this is the volcanological equivalent of the Nyquist criterion, which describes the sampling rate needed for a discrete record to represent a continuous signal without aliasing. With only a handful of monitored volcanoes, common processes controlled by a very small number of independent parameters would be sufficient to make each volcano appear unique. To describe each of the ~1500 volcanoes independently would require only 11 binary parameters (e.g., eruption style: explosive or effusive) or two parameters each with 40 discrete values (e.g., wt% SiO2). Perhaps more appropriately, 5 parameters each with 5 possible values (e.g., morphology: shield, stratovolcano, caldera, composite or fissure) would be sufficient to generate over 3000 apparently unique permutations.
Fortunately, volcanology is not the first discipline to face this problem, and we can draw inspiration from other fields. Every human being is both unique and immensely complex, yet medical science has defined “normal” parameters, as well as simple tests for distinguishing common from rare diseases. This example differs from the volcano problem, however, in that there are billions of accessible subjects and centuries of experience. Paleontology deals with an incomplete and biased fossil record using cladistics—a technique that groups organisms into evolutionary trees based on one or more characteristics inherited from common ancestors (Benton and Storrs, 1994). Progress has also been made on other natural phenomena, such weather forecasts, which combine research into a wide range of atmospheric processes, with vast computational resources and a probabilistic approach to prediction. We suggest that volcanology requires similar major advances. In fact, a pilot study of a cladistic approach to classifying volcanic landforms in Japan (Hone et al., 2007) shows that similar types of volcanoes tend to form spatial clusters, probably an influence of the deep feeder systems.
A long-term goal of volcanology is an overarching paradigm that integrates theory, experiments and observational data. Key elements of integrative science that could lead in this direction include (1) development and support of large databases that incorporate data that are heterogeneous in space, time and type (and include old as well as new observations); (2) new ways to integrate chemical and physical data; and (3) ways to track material movement (of magma and/or volatiles) in space and time. In fact, a specific goal of initiatives such as GVM, GVP and WOVOdata is to collate information in such a way that will enable such comparative and integrative studies. These endeavors will only be successful, however, if done within a framework that acknowledges the tension between uniqueness and commonality.
Developing a framework for volcanological systems that bridges from crustal (or even mantle) magma reservoirs to the surface and atmosphere will require contributions from all related research fields, including tectonics, geodesy and geophysics, petrology and geochemistry, fluid mechanics, physical volcanology, gas geochemistry and hydrology. It may be that all these aspects of volcanic systems need to be integrated before it will be possible to identify which (limited range of) parameters are required to characterize an individual volcano, and to group volcanoes as sufficiently “similar” that the Nyquist criterion is overcome. Nonetheless, current knowledge tells us what some of these parameters might look like. For example, it is clear that the material properties of magma (e.g., viscosity, which controls both transport properties and elemental diffusion, and volatile content) play a key role in eruptive behavior, as simply manifested in the difference in eruption style between Hawaiian basalts and hydrous rhyolites. Another set of parameters must relate to the crustal reservoir in which the magma is accumulated and stored prior to eruption: its extent, its location (both depth and tectonic setting) and the volume and distribution of “eruptible” melt. Also important are perturbations sufficient to trigger a change in state from non-eruptive (dormant) to eruptive (restless or active), such as magma input from below, gas accumulation within the reservoir and/or conduit, and changes in crustal stress. This (partial) list demonstrates the challenge in identifying a parsimonious parameter list for volcano classification.
In summary, although the idea of common processes at unique volcanoes is far from new, recognizing the conundrum this presents and critically assessing the transferability of lessons learned in one volcanic crisis to another presents a, and perhaps the, critical challenge for the volcanological community. In particular, we must ask whether existing detailed studies of a few volcanic eruptions (e.g., Mount St. Helens, USA, 1980-86 and 2004-2008; Pinatubo, Philippines, 1991; Unzen, Japan, 1991-1995; Soufriere Hills, Montserrat, 1995-present; Eyjafjallajökull, Iceland, 2010) are sufficient to cover the global spectrum. In our opinion, we currently run the risk of relying too heavily on individual “type examples” (e.g., Pinatubo for the effect of a large tropical eruption on global climate, or Eyjafjallajökull for ash transport and dispersion models) that may not provide the best guidance for future activity. We do not have a simple answer to this problem; we do feel, however, that the tension between the individual and the general provides an intellectually exciting perspective from which to view volcanological research, as well as a specific objective that, if solved, would go a long way toward improving our capability to handle global issues of volcanic hazard and risk.
Conflict of Interest Statement
The authors declare that the research was conducted in the absence of any commercial or financial relationships that could be construed as a potential conflict of interest.
Acknowledgments
This article began as a weekly discussion topic for the authors' respective research groups, inspired by the Acocella (2014) paper on “Grand Challenges in Volcanology.” It was further developed through discussions at the Oxford Volcano Seismology Workshop held in August 2014. Elements of the discussion also arose in the MeMoVolc workshop held in Geneva5, Switzerland in January 2014 (Lindsay et al., 2010). Alison Rust provided useful comments on the manuscript. The authors would like to thank all participants for their contributions.
Footnotes
1. ^http://www.volcano.si.edu/index.cfm
2. ^http://globalvolcanomodel.org/
3. ^http://www.bgs.ac.uk/vogripa/index.cfm
5. ^http://www.unige.ch/sciences/terre/mineral/CERG/MeMoVolc-Workshop/ConsDoc-MemoVolc-2014.pdf
References
Acocella, V. (2014). Great challenges in volcanology: how does the volcano factory work? Front. Earth Sci. 2:4. doi: 10.3389/feart.2014.00004
Bebbington, M. S. (2013). Assessing probabilistic forecasts of volcanic eruption onsets. Bull. Volcanol. 75, 1–13. doi: 10.1007/s00445-013-0783-5
Benton, M. J., and Storrs, G. W. (1994). Testing the quality of the fossil record: paleontological knowledge is improving. Geology 22, 111–114.
Biggs, J., Ebmeier, S. K., Aspinall, W. P., Lu, Z., Pritchard, M. E., Sparks, R. S. J., et al. (2014). Global link between deformation and volcanic eruption quantified by satellite imagery. Nat. Commun. 5:3471. doi: 10.1038/ncomms4471
Pubmed Abstract | Pubmed Full Text | CrossRef Full Text | Google Scholar
Cashman, K., and Blundy, J. (2000). Degassing and crystallization of ascending andesite and dacite. Philos. Trans. R. Soc. Lond. Ser. A 358, 1487–1513. doi: 10.1098/rsta.2000.0600
Ewert, J. W., Guffanti, M., and Murray, T. L. (2005). An Assessment of Volcanic Threat and Monitoring Capabilities in the United States: Framework for a National Volcano Early Warning System. USGS Open-File Report 2005–1164.
Fiske, R. S., Rose, T. R., Swanson, D. A., Champion, D. E., and McGeehin, J. P. (2009). Kulanaokuaiki Tephra (ca. A.D. 400–1000): newly recognized evidence for highly explosive eruptions at Kīlauea volcano, Hawai‘i. Geol. Soc. Am. Bull. 121, 712–728. doi: 10.1130/B26327.1
Hone, D. W. E., Mahoney, S. H., Sparks, R. S. J., and Martin, K. T. (2007). Cladistic analysis applied to the classification of volcanoes. Bull. Volcanol. 70, 203–220. doi: 10.1007/s00445-007-0132-7
Lindsay, J., Marzocchi, W., Jolly, G., Constantinescu, R., Selva, J., and Sandri, L. (2010). Towards real-time eruption forecasting in the Auckland Volcanic Field: application of BET_EF during the New Zealand National Disaster Exercise “Ruaumoko.” Bull. Volcanol. 72, 185–204. doi: 10.1007/s00445-009-0311-9
Lourdes, N. M., Roberto, T. C. A., Diego, G. M. M., Patricia, C. J. G., Héctor, C. V., and John, S. (1997). “Tornillo”-type seismic signals at Galeras volcano, Colombia, 1992–1993. J. Volcanol. Geother. Res. 77, 159–171.
Marzocchi, W., and Bebbington, M. S. (2012). Probabilistic eruption forecasting at short and long time scales. Bull. Volcanol. 74, 1777–1805. doi: 10.1007/s00445-012-0633-x
Miller, C. A., and Jolly, A. D. (2014). A model for developing best practice volcano monitoring: a combined threat assessment, consultation and network effectiveness approach. Nat. Hazards 71, 493–522. doi: 10.1007/s11069-013-0928-z
Newhall, C. G., and Self, S. (1982). The volcanic explosivity index (VEI) an estimate of explosive magnitude for historical volcanism. J. Geophys. Res. 87, 1231–1238. doi: 10.1029/JC087iC02p01231
Oppenheimer, C. (2003). Climatic, environmental and human consequences of the largest known historic eruption: Tambora volcano (Indonesia) 1815. Prog. Phys. Geogr. 27, 230–259. doi: 10.1191/0309133303pp379ra
Phillipson, G., Sobradelo, R., and Gottsmann, J. (2013). Global volcanic unrest in the 21st century: an analysis of the first decade. J. Volcanol. Geother. Res. 264, 183–196. doi: 10.1016/j.jvolgeores.2013.08.004
Schmidt, A., Ostro, B., Carslaw, K. S., Wilson, M., Thordarson, T., Mann, G. W., et al. (2011). Excess mortality in Europe following a future Laki-style Icelandic eruption. Proc. Natl. Acad. Sci. U.S.A. 108, 15710–15715. doi: 10.1073/pnas.1108569108
Pubmed Abstract | Pubmed Full Text | CrossRef Full Text | Google Scholar
Simkin, T., and Fiske, R. S. (1983). Krakatau, 1883–the Volcanic Eruption and its Effects. Washington, DC: Smithsonian Institution Press.
Sparks, R. S. J. (1997). Causes and consequences of pressurisation in lava dome eruptions. Earth Planet. Sci. Lett. 150, 177–189. doi: 10.1016/S0012-821X(97)00109-X
Stohl, A., Prata, A. J., Eckhardt, S., Clarisse, L., Durant, A., Henne, S., et al. (2011). Determination of time-and height-resolved volcanic ash emissions and their use for quantitative ash dispersion modeling: the 2010 Eyjafjallajökull eruption. Atmos. Chem. Phys. 11, 4333–4351. doi: 10.5194/acp-11-4333-2011
Keywords: comparative volcanology, global database, eruption style, type volcanoes, challenge in volcanology
Citation: Cashman K and Biggs J (2014) Common processes at unique volcanoes—a volcanological conundrum. Front. Earth Sci. 2:28. doi: 10.3389/feart.2014.00028
Received: 15 August 2014; Paper pending published: 02 September 2014;
Accepted: 05 October 2014; Published online: 03 November 2014.
Edited by:
Nicolas Fournier, GNS Science, New ZealandReviewed by:
Micol Todesco, Istituto Nazionale di Geofisica e Vulcanologia, ItalyBen Matthew Kennedy, University of Canterbury, New Zealand
Copyright © 2014 Cashman and Biggs. This is an open-access article distributed under the terms of the Creative Commons Attribution License (CC BY). The use, distribution or reproduction in other forums is permitted, provided the original author(s) or licensor are credited and that the original publication in this journal is cited, in accordance with accepted academic practice. No use, distribution or reproduction is permitted which does not comply with these terms.
*Correspondence: Katharine Cashman, School of Earth Sciences, University of Bristol, Queens Road, Bristol BS8 1RJ, UK e-mail: glkvc@bristol.ac.uk