- 1Xenobiotics Research Unit, School of Health and Biosciences, The Pontifical Catholic University of Parana, Curitiba, Brazil
- 2Faculty of Dentistry, School of Health and Biosciences, The Pontifical Catholic University of Parana, Curitiba, Brazil
Antifungal drugs belong to few chemical groups and such low diversity limits the therapeutic choices. The urgent need of innovative options has pushed researchers to search new bioactive molecules. Literature regarding the last 15 years reveals that different research groups have used different approaches to achieve such goal. However, the discovery of molecules with different mechanisms of action still demands considerable time and efforts. This review was conceived to present how Pharmaceutical Biotechnology might contribute to the discovery of molecules with antifungal properties by microbial biotransformation procedures. Authors present some aspects of (1) microbial biotransformation of herbal medicines and food; (2) possibility of major and minor molecular amendments in existing molecules by biocatalysis; (3) methodological improvements in processes involving whole cells and immobilized enzymes; (4) potential of endophytic fungi to produce antimicrobials by bioconversions; and (5) in silico research driving to the improvement of molecules. All these issues belong to a new conception of transformation procedures, so-called “green chemistry,” which aims the highest possible efficiency with reduced production of waste and the smallest environmental impact.
Introduction
The search for new molecules has forced the pharmaceutical industry to modernize its synthetic processes. Such modernization has occurred with the adoption of new techniques, such as miniaturization, nanotechnology, microdosing, chemometrics, and high-throughput analysis (Koh et al., 2003). Another aspect of modernization, so-called “Green Chemistry,” requires new synthetic processes with the highest possible efficiency, resulting in reduced production of waste and the smallest environmental impact (Tucker, 2006).
More than 20,000 molecules with antibiotic activity that are produced by microorganisms have been described since the discovery of penicillin by Sir Alexander Fleming; however, only a small fraction of them are clinically useful due to their toxicity. Since the 1980s, a decline in the discovery of new molecules has been observed (Murphy, 2012).
The antifungal agents available on the market act on different targets such as ergosterol synthesis, chitin synthesis, glucan synthesis, nucleic acid synthesis, protein synthesis, microtubule synthesis, or as inhibitors of squalene epoxidase or ergosterol disruptors (Kathiravan et al., 2012). However, although there is substantial variability in the mechanisms of action and despite the technical advances, the development of new antifungal drugs persists considering the co-evolution of resistance mechanisms. Between 2006 and 2010, only one antifungal was approved for use, a natural echinocandin that was chemically changed by a semi-synthetic route (Chen et al., 2011). In the last 30 years, among the 28 new naturally occurring molecules, only three semi-synthetic molecules that underwent chemical changes have been authorized for clinical use (Newman and Cragg, 2012).
It is estimated that 25% of the world's population presents some episode of superficial mycosis and the mortality rate associated with invasive fungal infections frequently exceeds 50%, even with the available antifungal medications. This amount corresponds to approximately 1.5 million deaths annually (Brown et al., 2012). Even under prophylactic use with antifungals, some changes in epidemiological features have been reported in more aggressive mucormicoses in patients using voriconazole, as well as the rampant development of resistance to azole by Aspergillus spp. (Perfect et al., 2014).
Echinocandins and allylamines are more modern drug classes approved, but they date back to the 1970s and 1980s, respectively (Odds, 2003). Echinocandins have been used as the treatment of choice for systemic candidoses and are effective for strains resistant to azoles (Zimbeck et al., 2010; Eschenauer et al., 2014); however, a mutation in the FKS gene increases the resistance of Candida spp. to echinocandins (Zimbeck et al., 2010; Beyda et al., 2012). A multicenter study demonstrated that C. glabrata and C. krusei have lost their susceptibility to caspofungin (24 and 52%, respectively) and that other common Candida species are rapidly losing their susceptibility to echinocandins (Zimbeck et al., 2010).
Even assuming great advances in synthetic chemistry, biotransformation (or biocatalysis) remains the most cost-effective path to discover new pharmaceuticals (Zaks and Dodds, 1997). Special attention should be given to the significant number of drugs produced by microorganisms or by interactions with the host from which they were isolated. Both cases contribute to the idea that biotransformation processes shall expand significantly in the future (Newman and Cragg, 2012). Nevertheless, there are some questions regarding biotransformation that have to be addressed: (1) How can structural changes occur in a way to make the processes more time- and cost-efficient? (2) How can the biological activity of products be enhanced by optimizing the pharmacokinetic/pharmacodynamic (PK/PD) properties and safety? (3) How may a supplier guarantee large-scale drug production under good quality practices? (Bauer and Brönstrup, 2014).
With the appropriation of concepts from White Biotechnology and Green Chemistry, this review aims to assess the technological advances in the development of microbial biotransformation products with antifungal activity.
Microbial Metabolism vs. Microbial Biotransformation
Natural products compose more than 2/3 of antibiotics used in the medical/dental/veterinary practice (Schmitz et al., 2013). Thus, it is not an erroneous statement to assume that active substances from plants or those isolated from microorganisms are the simplest way to search for new molecules. If they have antifungal potential, some criteria must be observed (Barrett, 2002): (1) Do they present novel mechanisms of action or any useful known mechanisms? (2) Is it possible to obtain clinical proof with good biological activity? (3) Is it possible to change the molecule to make it a tolerable drug? A good example of a bioactive molecule obtained from plant extracts is eugenol, which is extracted from Eugenia caryophillis (Indian clove). It is a phenylpropanoid that presents considerable fungicidal activity in vitro against C. albicans, and, unlike fluconazole, is also effective against C. krusei and C. glabrata (Ahmad et al., 2010).
In addition to plants, microorganisms have provided some bioactive molecules with remarkable antimicrobial activity, especially in the last two decades.
For fungal infections, the glycopeptide occidiofungin A is produced by Burkholderia contaminans MS14 and presents great antifungal activity against pathogens of plants and animals (Lu et al., 2009). Its mechanism of action has not been elucidated, but it is assumed that it differs from the known classes and can bypass fungal resistance problems (Tan et al., 2012). Benanomicin A and benanomicin B are fermentatively produced by the cultivation of Actinomadura spadix MH193-16F4 and are broad-spectrum antimicrobials against various fungi including endemic and opportunistic pathogens (Kumagai et al., 2008).
Although there remains a myriad of naturally occurring secondary metabolites to be evaluated and discovered, microbial biotransformation has emerged as an important tool for obtaining novel structural analogs or to improve the pharmacokinetic parameters of other substances (Parshikov et al., 2000; Borges et al., 2008; Baydoun et al., 2014).
It is important to emphasize that metabolism and biotransformation are distinct systems of molecular processing. Microbial metabolism is composed of two major processes; primary metabolism, which is responsible for cellular function, and secondary metabolism, which uses pre-existing metabolic pathways to produce substances from endogenous intermediates to allow better adaptation of the organism to the environment (Keller et al., 2005; Brakhage, 2013). Primary metabolism consists of reactions associated with energy generating, biomass production, and essential cell components. Events such as glycolysis, oxidative phosphorylation, and the Calvin-Benson cycle (in algae and photosynthesizing bacteria) are examples of typical sets of reactions of primary metabolism.
In contrast, secondary metabolism involves important events for adaptation to environmental conditions. In general, it generates low molar mass metabolites that are not essential for growth but that offer some advantages and may sometimes have medical/veterinary/agricultural/industrial importance. They include antibiotics, pigments, anti-tumor agents, etc. They have unusual structures and are normally synthesized during the late phase of cell growth (Ruiz et al., 2010).
Regarding to antibiotics, it is estimated that actinomycetes are responsible for 70–80% of all molecules produced by secondary metabolism. Different species belonging to the genus Streptomyces produces important antibiotics as chloramphenicol, streptomycin, macrolides, and rifampicin, among others (Raja and Prabakarana, 2011). A recent review regarding to this theme presented new perspectives about the optimization in the production of actinomycetes-derived antibiotics (Antoraz et al., 2015).
Biotransformation, sometimes inaccurately called “xenobiotic metabolism,” is responsible for minor structural modifications in exogenous substances by enzyme systems that lead to the formation of molecules with relatively greater polarity (Asha and Vidyavathi, 2009; Pervaiz et al., 2013). Phenomena such as stereoselective hydroxylation, epoxidation, and oxidation are common reactions attributed to biotransformation processes and have been reported to occur in fungi (Farooq et al., 2002; Choudhary et al., 2005, 2007, 2009, 2010; Al-Aboudi et al., 2009). It is a mechanism that microorganisms developed to adapt to environmental changes and it is useful in a wide range of biotechnological processes (Crešnar and Petric, 2011).
One of the most remarkable features of biotransformation reactions is the maintenance of the original carbon skeleton after obtaining the products. During metabolism, the carbon atoms are transferred to other molecules with different chemical functions (Figure 1).
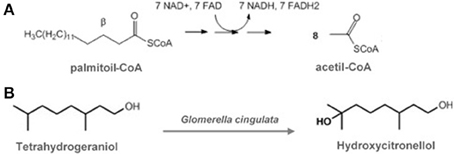
Figure 1. (A) Typical multi-step metabolic reactions, with deep alterations on carbon skeleton (in this case, fatty acid oxidation). (B) Typical one-step biotransformation reaction, with minor punctual alterations on carbon skeleton (Adapted from: Hegazy et al., 2015).
Biotransformation reactions may involve various events such as the formation of stable intermediates, which may be devoid of toxic or pharmacologic activity. Sometimes, short-lived reactants may also be generated. Further, biotransformation reactions can result in chemically stable compounds with desired pharmacological activity (Fura, 2006).
The use of microbial biotransformation is part of a new movement named White Biotechnology, which is an emerging field of modern biotechnology that serves industry. It employs living cells (animals, plants, algae, filamentous fungi, yeast, actinomycetes, and bacteria), as well as enzymes produced by these cells during the generation of products of interest.
Wisely, Venisetty and Ciddi (2003) presented nine practical advantages in the use of microbial systems as models for drug metabolism:
(1) The low-cost and facility to maintain stock cultures of microorganisms;
(2) Procedures with large number of strains are simple repetitive processes;
(3) The concentrations of parental molecules used (generally ranging from 0.2 to 0.5 g/L) are much higher than those employed in other cell or tissue models;
(4) Novel products can be isolated with new or different activities;
(5) There is a possibility to predict the most favored metabolic reactions;
(6) The models can be scaled up easily for the preparation of metabolites for pharmacological and toxicological evaluation;
(7) These models can be utilized in synthetic reactions where tedious steps are involved;
(8) In most cases, relatively mild incubation conditions are used;
(9) The models can be useful in cases where regio- and stereo-specificity are involved, becoming molecular handling more easily achieved by biotransformation than by synthetic chemistry.
With regards to these last two statements, obtaining an antimicrobial may become critically laborious if only chemical procedures are employed, even for semi-synthetic compounds (Wild, 1994; Claes et al., 2013). In this context, microbial biotransformation becomes an attractive resource.
Living cells can be used in their original state (wild strains) or improved to work as “cell factories” to produce enzymes or consumer goods (Carballeira et al., 2009). Despite their potential, the number and diversity of applications is still modest when considering the wide availability of microorganisms, the large number of reactions that they can achieve and the fact that biotransformation reactions are considered economically and ecologically competitive (Borges et al., 2009).
Biotransformation by Whole Cells vs. Immobilized Enzymes
It is insufficient to know the microbial biotransformation pathways to establish whether they are economically useful; rather, it is necessary to define reproducibility at a production scale. This assumption demands some concern regarding raw materials (the molecule to be biotransformed and the microorganism/enzyme responsible for the reaction), equipment (bioreactors), and the necessary technology to be employed in the purification of the products (downstream processing). In addition, it is imperative to take into account the lowest possible production of pollutants and the highest possible enantiomeric purity of the final product.
The criteria above are focuses of Green Chemistry that have allowed the construction of chiral chemical building blocks that lead to the development of enantiopure products obtained under mild reaction conditions at physiological pH and temperature, using water as reaction milieu and environmentally friendly catalysts (enzymes or cells). The obtained products are usually multifunctional molecules that exhibit highly chemo-regio-stereoselective activity (Borges et al., 2009; Muñoz Solano et al., 2012).
Immobilized enzymes are used for the conversion of molecules in many industrial fields, although they are preferably directed to simple catalytic processes. For the production of antimicrobials, the applicability of individualized enzymes in stricto sensu biotransformation processes is still incipient and little explored (Banerjee, 1993a,b; Mujawar, 1999; Takimoto et al., 2004; Hormigo et al., 2010). Possibly, this may result from the functional complexity required, which is readily attainable by living organisms.
An important enzyme feature that has not been properly explored is their promiscuity, which is the ability of enzymes to catalyze different reactions with distinct catalytic mechanisms to create new pathways (Wu et al., 2010). If we obtained a new molecule with remarkable antifungal properties but with inappropriate characteristics for ADMET (administration, distribution, metabolism, excretion, and toxicity), it is possible to “improve” its structure by enzyme promiscuity. For this, in silico technologies favor technical development processes, predicting PK/PD characteristics and increasing the use of immobilized enzymes in the synthesis of pharmaceuticals.
Some complex processes of anti-proliferation production via enzymatic biotransformation are already known. A good example is the biosynthesis of griseofulvin from malonyl-CoA using purified enzymes (Cacho et al., 2013). Several phases including aldol condensation, cyclization, halogenation, and oxidation are enzyme-mediated steps that can be reproduced without the need of a living organism.
Penicillin G acylase (PGA) is one of the most relevant and widely used biocatalysts for the industrial production of β-lactam semisynthetic antibiotics (Srirangan et al., 2013). Such an enzyme may be bulk produced as heterologous PGA in competent strains such as Escherichia coli ATCC® 11105™ (Erarslan et al., 1990) or Bacillus badius PGS10 (Rajendran et al., 2011).
Considering the processes using whole cells, it is assumed that fungi are the organisms most commonly used to obtain natural metabolic products and for biotransformation reactions (Borges et al., 2009), even for the procurement of antifungal drugs; however, microorganisms from other kingdoms may also conduct dedicated biotransformation processes in order to obtain antifungal molecules (Table 1).
The use of whole cells is advantageous once they present all the needed enzymes and cofactors in adequate concentrations and energy status. These favorable conditions may modulate the activity of multienzymatic complexes and contribute to increase conversion rates (Restaino et al., 2014).
The obtaining of biotransformation products may be conducted using co-cultivation of two or more distinct entities. It has been proposed by Wu et al. (2015), who reported the increased obtaining of bioactive molecules during the co-cultivation of Streptomyces coelicolor A3(2)M145 (actinomycete) and Aspergillus niger N402 (fungus). According to the authors, “growth in microbial communities or interactions between different microorganisms is the next logical step in the search for new molecules.”
Another interesting approach involves the possibility of heterologous expression of biotransformation-related cytochrome P450 enzymes from actinomycetes in bacteria to obtain new antimycotic derivates (Kumagai et al., 2008). It becomes useful for biotransformation procedures in large scale producing plants where large bioreactors are employed.
It has been reported that pyrethrosin, a germacrane sesquiterpene lactone commonly found in Chrysanthemum cinerariaefolium Visiani (Asteraceae), can be converted to several new molecules by Cunninghamella elegans NRRL1392 and Rhizopus nigricans NRRL1477 (Galal, 2001). Such filamentous fungi are able to completely deplete pyrethrosin, transforming it into five more polar metabolites that are very active against Cryptococcus neoformans (IC50 = 25–35.0 μg.mL−1) and Candida albicans (IC50 = 10–30 μg.mL−1).
The search for new antifungal molecules via microbial biotransformation involves not only purified precursor molecules, but may encompass complex substrates that can be bioconverted into extracts rich in active substances.
Biotransformation products of cabbage-crude extracts (Brassica oleracea var. capitata) processed by Pseudomonas syringe pv. T1 showed promising inhibitory activity for several Candida spp., with values close to those obtained for amphotericin B (Bajpai et al., 2011). Pectobacterium atrosepticum Pepto-A, a Gram-negative plant pathogen, can also be produced from cabbage extracts to yield anti-Candida compounds (Bajpai et al., 2012a).
Another Pectobacterium species, P. carotovorum subsp. carotovorum 21, also presented the ability to biotransform compounds found in cabbage (Bajpai et al., 2010a) and in tomatoes (Solanun esculentum) to generate others with remarkable activity against Candida spp. (Bajpai et al., 2012b,c).
Biotransformation has been successfully utilized as a tool to generate pharmaceutical compounds from natural products. Through this process, ethyl p-methoxycinnamate (EPMC) was extracted from Kaempferia galanga (a Malaysian plant) and was transformed using Aspergilus niger to ethyl p-hydroxycinnamate (EPHC) (Omar et al., 2014). Looking at antimicrobial activities, EPHC has a potential inhibitory effect against C. albicans that is better than the effect by EPMC. This highlights the possibility of increasing antifungal value to other known antifungal molecules.
Studies in this area shall be directed toward the diversification of substrates combined with the numerous species of biotransforming microorganisms. This can thus result in the expansion of the antifungal library. In a recent review, a number of molecules with antimicrobial activity derived from monoterpenes have been described after biotransformation processes by various microorganisms (Bhatti et al., 2014).
Endophytic Fungi Produce Antimicrobials by Biotransformation
Despite the substantial worldwide diversity, the discovery of new families of bioactive molecules is surprisingly declining, driving the need to bioprospect new sources (Joseph and Priya, 2011). Following such a thought, a group with promising potential for new discoveries in the biotransformation area is that of endophytic fungi. In recent years, endophytic fungi have garnered great interest.
They are organisms that can grow either intra or extracellularly in the tissues of higher plants in a clear mutualistic relationship without causing any symptoms. Evidence shows that they are a rich source of bioactive natural products. Active metabolites of endophytics show positive actions as antibiotics, immunosuppressives, anti-helminthics, antioxidants, and anticancer drugs (Pimentel et al., 2011).
There is a noticeable increase in the rate of resistance to antimicrobials, which is, at least in part, related to the insufficient number of effective molecules and the small amount of new antimicrobial agents in development, probably due to unfavorable investment returns. In this context, the endophytic fungi present themselves as an attractive alternative to modify the current paradigm (Molina et al., 2012).
However, although a promising field, some problems cannot be ignored in studies with endophytes. Special attention is recommended in face of:
(1) The high nonspecific toxicity of some antimicrobials already obtained;
(2) The fact that fungi tend to not produce toxic substances against themselves, resulting in antimicrobial compounds with moderate antifungal activity without potential as a medicament or pesticide;
(3) The difficulty in large scale production of certain antimicrobial compounds in artificial culture media;
(4) The biosynthesis and regulation of production of antimicrobials from endophytes are partially or totally unknown (Yu et al., 2010).
It is important to mention that many of the products related to endophytics are derived from their secondary metabolism, and do not necessarily have a biotransformation background. In some cases, it is not clear whether the final product is the result of secondary metabolism or biotransformation. In spite of this last finding, it is common sense amongst investigators devoted to biotransformation that these fungi present vast potential (Pimentel et al., 2011).
Table 2 presents some molecules with antifungal activity that are produced exclusively by endophytic fungi via secondary metabolism. As such, organisms that usually live in harsh conditions in the presence of numerous natural compounds are expected to be exceptional biotransforming entities (Shibuya et al., 2003, 2005; Agusta et al., 2005; Fu et al., 2011; Maehara et al., 2011; Huang et al., 2015; Khoyratty et al., 2015). Although endophytic fungi are promising organisms for biotransformation processes, their ability and potential to produce antimicrobial molecules remain unexplored.
Candidate Molecules for Biotransformation
One point of concern when evaluating the feasibility of producing antifungals via microbial biotransformation is the choice of parental molecules to be modified. Such chemicals must not be toxic or inhibitory to the biotransforming organism. However, this can be circumvented if the concentrations during fermentation processes remain inferior to those considered as inhibitory.
Accumulated data has revealed that some classes of molecules seem to be more ready to undergo biotransformation and generate antifungals.
Some unsaturated fatty acids have shown interesting results. Bajpai et al. (2009a, 2010b) have conducted extensive reviews regarding this matter and reported that Pseudomonas aeruginosa NRRL-B-18602 PR3 produced mono-, di-, and tri-hydroxy fatty acid derivatives from unsaturated fatty acids with recognized antifungal properties. This bacterium can convert oleic acid to 7,10-dihydroxy-8(E)-octadecenoic acid, an anticandidal compound (Hou and Forman, 2000).
Other unsaturated fatty acids can also undergo oxidation in the presence of bacteria (Pseudomonas sp. 42A2 or Bacillus megaterium ALA2 NRRL-B-21660) or plants (Colocassia antiquorum) to produce mono-, di-, and tri-hydroxy fatty acids with antifungal potential such as 15,18-dihydroxy-14,17-epoxy-5(Z),8(Z), 11(Z)-eicosatrienoic acid, 17,20-dihydroxy-16,19-epoxy-4(Z),7(Z),10(Z),13(Z)- docosatetraenoic, 9,12,13-trihydroxy-(E)-octadecenoic acid, 12,13,16-trihydroxy- 9(Z)-octadecenoic acid, and 12,13,17-trihydroxy-9(Z)-octadecenoic acid (Masui et al., 1989; De Andrés et al., 1994; Hou, 1996; Hou et al., 1997; Hosokawa et al., 2003a,b).
Based on the mechanism proposed for (Z)-9-heptadecenoic acid (Avis and Bélanger, 2001), the most probable antifungal mechanism of action must involve the disruption or disintegration of the plasma membrane caused by a hydrostatic turgor pressure within the cell resulting in the release of intracellular electrolytes and proteins (Carballeira, 2008).
Another class of molecules with a promising outlook are sterols. This class of substances has been evaluated in relation to their ability to be biotransformed for many years (Mahato and Mukherjee, 1984; Mahato and Garai, 1997; Holland, 1999; Malaviya and Gomes, 2008; Bhatti and Khera, 2012; Donova and Egorova, 2012). Surprisingly, there are few investigations on the production of antifungals, despite the fact that many compounds such as 24-amino-lanosterol, 24-amino-cholesterol, and 24-amino-cholesterol-N-sulfate possess potent antifungal activities against Candida spp., C. neoformans, and Trichophyton mentagrophytes (Chung et al., 1998).
Preliminary studies have shown that steroids and steroidal lactones biotransformed by Cunninghamella spp. produce metabolites with leishmanicidal activity (Choudhary et al., 2006; Baydoun et al., 2014). It is reasonable that such metabolites act on the synthetic pathway of ergosterol in Leishmania spp. We speculate that these putative mechanisms of action may be extrapolated to fungi, which should encourage investigators to drive their efforts toward this problem.
Alkaloids may also be converted into antifungal compounds. As part of an extensive program which aimed the discovery and development of antimicrobials from higher plants, Orabi and colleagues conducted a series of experiments in order to obtain antifungals from sampangine, an alkaloid found in the West African tree Cleistophathis patens (Annonaceae) (Orabi et al., 1999). Their results showed that Beauveria bassiana ATCC®7159™, Doratomyces microsporus ATCC®16225™, and Filobasidiella neoformans ATCC®10226™ produced the 4′-O-methyl-β-glucopyranose conjugate, while Absidia glauca ATCC®22752™, Cunninghamella elegans ATCC®9245™, Cunninghamella sp. NRRL5695, and Rhizopus arrhizus ATCC®11145™ produced the β-glucopyranose conjugate. Both metabolites presented significant in vitro activity against C. neoformans, but were inactive against C. albicans (Orabi et al., 1999).
The same group published interesting results about the biotransformation of the synthetic antifungal alkaloid benzosampangine (Orabi et al., 2000). They showed that Absidia glauca ATCC®22752™, Cunninghamella blakesleeana ATCC®8688a™, Cunninghamella sp. NRRL5695, Fusarium solani f. sp. cucurbitae CSIH#C-5, and Rhizopogon species ATCC®36060™ each produced a β-glucopyranose conjugate of benzosampangine. Such a substance possesses good in vitro antifungal activity against C. albicans, Aspergillus fumigatus (MIC = 0.39 μg.mL−1; amphotericin B: 0.78 μg.mL−1 and 0.39 μg.mL−1, respectively), and C. neoformans (MIC = 1.56 μg.mL−1; amphotercin B, 0.39 μg.mL−1). The authors emphasized that microbial biotransformation is reliable and produces significant quantities of metabolites. In addition, they showed that alkaloids could be converted into conjugate metabolites with increased antifungal activity.
In silico Predictive Improvement of Bioprocessable Molecules
In silico is the term used to define experimentation carried out in computers. In turn, in silico pharmacology is a large growing area that helps to develop molecular arrangements using dedicated software to capture, analyze and integrate biological and medical data (Ekins et al., 2007). The use of in silico techniques allows the prediction of the pharmacokinetic aspects of absorption, distribution, biotransformation and excretion of new substances. It can be assumed that in silico projections of new molecules allow the prioritization of chemicals to be tested, identifying hazard and risk assessment (Kulkarni et al., 2005). The computer-assisted simulations based on pharmacological and biological data reduce time and costs during the screening of new substances once they cease to categorize undesirable molecules with improper characteristics during the early stages of discovery.
In addition, it is possible to evaluate the coupling of molecules to their possible targets. Using databases of 3D molecular structures, it is possible to anticipate connections between new molecules and possible binding sites. Numerous substances have been evaluated by this method, and it was possible to propose important features of some of them as the mechanism of action of antifungal piranocoumarins and antibacterial xanthone derivatives (Do et al., 2015).
CaCYP51 is a sterol 14-α demethylase that binds the CYP51 substrates lanosterol and eburicol in C. albicans. In silico techniques based on the molecular structure of CaCYP51 have allowed the development of new azoles by replacing the side chains provided in the simulation. The new azoles showed excellent antifungal activity in vitro with broad spectrum (Che et al., 2009). The development of new molecules in silico largely depends on the molecular recognition of possible couplings between the drug and microorganisms. Therefore, once elucidated, the mechanism of action of an antifungal obtained by biotransformation will allow the simulation of molecules with improved pharmacokinetic characteristics and may accelerate the development of potential drugs (Rask-Andersen et al., 2011).
Perspectives
The prospection of new molecules, principally from endophytic fungi, is a vast field of study with huge potential for growth. However, consistent efforts are necessary to achieve results. Professionals from the fields of biotechnology, pharmacology, computer sciences, engineering and some other related areas have to work closely together to explore possible binding sites for new and existing molecules. The use of in silico analyses anticipate investigations in a timesaving manner and reduce the demands for inputs and even animals used in the initial tests. These features drive future research toward the development of new and feasible technologies within the Green Chemistry guidelines connected to the principle of the 3Rs (replacement, reducing, refinement) for the use of experimental animals (Russell and Burch, 1959).
A reasonable research design for the prospecting of new antifungal drugs under the above stated conditions involves the following:
(1) The in silico analysis of existing molecules for possible new couplings to different targets;
(2) The in silico identification of necessary changes in the precursor molecule for effective coupling on targets;
(3) In the absence of new discoveries, the biotransformation of various candidate substrates for obtaining new molecules;
(4) The recognition of which organisms or isolated enzymes may modify structural precursor molecules to yield enantiomerically pure antifungal molecules based on the theory of enzyme promiscuity;
(5) The in silico evaluation of toxicity for newly developed antifungal molecules;
(6) The process of scaling up with environmentally friendly inputs to test the economic viability.
Once the goals outlined above are achieved, the investigator is ready to conduct clinical trials to confirm the PK/PD properties, security, and all other steps necessary for commercialization of the drug.
It is important to notice that with the advent of in silico technologies, laboratory tests tend to reduce their margin of error, and therefore, save both environmental and financial resources. However, they primarily accelerate new discoveries to minimize the impact of developed resistance to existing drugs.
Therefore, it is necessary to sequence and deposit the genomes of microorganisms with biotransformation capacity in databases. In addition, further study on the molecular structure of possible targets in pathogenic fungi is mandatory. This will provide subsidies to find coupling regions for the developed molecules.
Currently, we have high cost and low return as factors related to the decline in the development of new antimicrobial drugs. There is no other way to achieve such a market if the costs of research are not reduced and production is enabled in a sustainable way.
Funding
This review was supported entirely by the Pontifical Catholic University of Parana, to whom the authors are grateful.
Conflict of Interest Statement
The authors declare that the research was conducted in the absence of any commercial or financial relationships that could be construed as a potential conflict of interest.
References
Agusta, A., Maehara, S., Ohashi, K., Simanjuntak, P., and Shibuya, H. (2005). Stereoselective oxidation at C-4 of flavans by the endophytic fungus Diaporthe sp. isolated from a tea plant. Chem. Pharm. Bull. 53, 1565–1569. doi: 10.1248/cpb.53.1565
Ahmad, A., Khan, A., Manzoor, N., and Khan, L. A. (2010). Evolution of ergosterol biosynthesis inhibitors as fungicidal against Candida. Microb. Pathog. 48, 35–41. doi: 10.1016/j.micpath.2009.10.001
Al-Aboudi, A., Mohammad, M. Y., Haddad, S., Al-Far, R., Choudhary, M. I., and Atta-Ur-Rahman. (2009). Biotransformation of methyl cholate by Aspergillus niger. Steroids 74, 483–486. doi: 10.1016/j.steroids.2009.01.002
Antoraz, S., Santamaría, R. I., Díaz, M., Sanz, D., and Rodríguez, H. (2015). Toward a new focus in antibiotic and drug discovery from the Streptomyces arsenal. Front. Microbiol. 6:461. doi: 10.3389/fmicb.2015.00461
Asha, S., and Vidyavathi, M. (2009). Cunninghamella - a microbial model for drug metabolism studies-a review. Biotechnol. Adv. 27, 16–29. doi: 10.1016/j.biotechadv.2008.07.005
Avis, T. J., and Bélanger, R. R. (2001). Specificity and mode of action of the antifungal fatty acid cis-9-heptadecenoic acid produced by Pseudozyma flocculosa. Appl. Environ. Microbiol. 67, 956–960. doi: 10.1128/AEM.67.2.956-960.2001
Bajpai, V. K., and Kang, S. C. (2007). Antifungal potential of bioconverted product of eicosapentaenoic acid. KSBB 10, 67–68.
Bajpai, V. K., Kang, S. C., and Baek, K. H. (2012a). Microbial fermentation of cabbage by a bacterial strain of Pectobacterium atrosepticum for the production of bioactive material against Candida species. J. Mycol. Med. 22, 21–29. doi: 10.1016/j.mycmed.2011.11.004
Bajpai, V. K., Kang, S. C., Heus, S., Shukla, S., Lee, S., and Baek, K. H. (2010a). Microbial conversion and anticandidal effects of bioconverted product of cabbage (Brassica oleracea) by Pectobacterium carotovorum pv. carotovorum 21. Food. Chem. Toxicol. 48, 2719–2724. doi: 10.1016/j.fct.2010.06.046
Bajpai, V. K., Kang, S. C., Kim, H. R., and Hou, C. T. (2010b). “Potential approach of microbial conversion to develop new antifungal products of omega-3 fatty acids,” in Biocatalysis and Biomolecular Engineering, eds C. T. Hou and J. F. Shaw (Hoboken, NJ: John Wiley & Sons), 459–471.
Bajpai, V. K., Kang, S. C., Lee, S. G., and Baek, K. H. (2012c). Microbial conversion of tomato by a plant pathogenic bacterium Pectobacterium atrosepticum: a plant-microbial approach to control pathogenic Candida species. Nat. Prod. Commun. 7, 65–69.
Bajpai, V. K., Kang, S. C., Park, E., Jeon, W. T., and Baek, K. H. (2011). Diverse role of microbially bioconverted product of cabbage (Brassica oleracea) by Pseudomonas syringe pv. T1 on inhibiting Candida species. Food Chem. Toxicol. 49, 403–407. doi: 10.1016/j.fct.2010.11.015
Bajpai, V. K., Kan, S. C., Kim, S., Hasan, M. M., Dhakal, R., and Park, E. (2012b). Microbial conversion of tomato by Pectobacterium carotovorum subsp. carotovorum 21: a biotechnological approach to control pathogenic Candida species. Roman. Biotechnol. Lett. 17, 6989–6998.
Bajpai, V. K., Kim, H. R., Hou, C. T., and Kang, S. C. (2009a). Bioconverted products of essential fatty acids as potential antimicrobial agents. N. Biotechnol. 26, 122–130. doi: 10.1016/j.nbt.2009.07.007
Bajpai, V. K., Kim, H. R., Hou, C. T., and Kang, S. C. (2009b). Microbial conversion and in vitro and in vivo antifungal assessment of bioconverted docosahexaenoic acid (bDHA) used against agricultural plant pathogenic fungi. J. Ind. Microbiol. Biotechnol. 36, 695–704. doi: 10.1007/s10295-009-0539-6
Bajpai, V. K., Kim, H. R., and Kang, S. C. (2006). In vitro anti-fungal activity of various hydroxylated fatty acids bioconverted by Pseudomonas aeruginosa PR3. Agric. Chem. Biotechnol. 49, 131–134.
Bajpai, V. K., Shin, S. Y., Kim, H. R., and Kang, S. C. (2008). Anti-fungal action of bioconverted eicosapentaenoic acid (bEPA) against plant pathogens. Ind. Crop. Prod. 27, 136–141. doi: 10.1016/j.indcrop.2007.07.014
Banerjee, U. C. (1993a). Characterization of rifamycin oxidase immobilized on alginate gel. Biomater. Artif. Cells Immobilization Biotechnol. 21, 675–683. doi: 10.3109/10731199309117392
Banerjee, U. C. (1993b). Studies on rifamycin oxidase immobilized on kappa-carrageenan gel. Biomater. Artif. Cells Immobilization Biotechnol. 21, 665–674.
Barrett, D. (2002). From natural products to clinically useful antifungals. Biochim. Biophys. Acta 1587, 224–233. doi: 10.1016/S0925-4439(02)00085-6
Bauer, A., and Brönstrup, M. (2014). Industrial natural product chemistry for drug discovery and development. Nat. Prod. Rep. 31, 35. doi: 10.1039/C3NP70058E
Baydoun, E., Karam, M., Atia-tul-Wahab Khan Ahmad, M. S., Samreen, M. S., et al. (2014). Microbial transformation of nandrolone with Cunninghamella echinulata and Cunninghamella blakesleeana and evaluation of leishmaniacidal activity of transformed products. Steroids. 88, 95–100. doi: 10.1016/j.steroids.2014.06.020
Beyda, N. D., Lewis, R. E., and Garey, K. W. (2012). Echinocandin resistence in Candida species: mechanisms of reduced susceptibility and therapeutic approaches. Ann. Pharmacother. 46, 1086–1096. doi: 10.1345/aph.1R020
Bhatti, H. N., Khan, S. S., Khan, A., Rani, M., Ahmad, V. U., and Choudhary, M. I. (2014). Biotransformation of monoterpenoids and their antimicrobial activities. Phytomedicine 21, 1597–1626. doi: 10.1016/j.phymed.2014.05.011
Bhatti, H. N., and Khera, R. A. (2012). Biological transformations of steroidal compounds: a review. Steroids 77, 1267–1290. doi: 10.1016/j.steroids.2012.07.018
Boonphong, S., Kittakoop, P., Isaka, M., Pittayakhajonwut, D., Tanticharoen, M., and Thebtaranonth, Y. (2001). Multiploides A and B, new antifungal 10-membered lactones from Xylaria multiplex. J. Nat. Prod. 64, 965–967. doi: 10.1021/np000291p
Borges, K. B., Borges, W. D. S., Pupo, M. T., and Bonato, P. S. (2008). Stereoselective analysis of thioridazine-2-sulfoxide and thioridazine-5-sulfoxide: an investigation of rac-thioridazine biotransformation by some endophytic fungi. J. Pharm. Biomed. Anal. 46, 945–952. doi: 10.1016/j.jpba.2007.05.018
Borges, K. B., Borges, W. S., Durán-Patrón, R., Pupo, M. T., Bonato, P. S., and Collado, I. G. (2009). Stereoselective biotransformations using fungi as biocatalysts. Tetrahedron: Asymmetry 20, 385–397. doi: 10.1016/j.tetasy.2009.02.009
Brakhage, A. A. (2013). Regulation of fungal secondary metabolism. Nat. Rev. Microbiol. 1, 21–32. doi: 10.1038/nrmicro2916
Brown, G. D., Denning, D. W., Gow, N. A., Levitz, S. M., Netea, M. G., and White, T. C. (2012). Hidden killers: human fungal infections. Sci. Transl. Med. 4:165rv13. doi: 10.1126/scitranslmed.3004404
Cacho, R. A., Chooi, Y.-H, Zou, H., and Tang, Y. (2013). Complexity generation in fungal polyketide biosynthesis: a spirocycle-forming P450 in the concise pathway to the antifungal drug griseofulvin. ACS Chem. Biol. 8, 2322–2330. doi: 10.1021/cb400541z
Cafêu, M. C., Silva, G. H., Teles, H. L., Helder, L., Bolzani, V. S., Araujo, A. R., et al. (2005). Substâncias antifúngicas de Xylaria sp., um fungo endofíticos isolado de Palicourea macgravii (Rubiaceae). Quim Nova 28, 991–995. doi: 10.1590/S0100-40422005000600011
Carballeira, J. D., Quezada, M. A., Hoyos, P., Simeó, Y., Hernaiz, M. J., Alcantara, A. R., et al. (2009). Microbial cells as catalysts for stereoseletive red-ox reactions. Biotechnol. Adv. 27, 685–714. doi: 10.1016/j.biotechadv.2009.05.001
Carballeira, N. M. (2008). New advances in fatty acids as antimalarial, antimycobacterial and antifungal agents. Prog. Lipid Res. 47, 50–61. doi: 10.1016/j.plipres.2007.10.002
Che, X., Sheng, C., Wang, W., Cao, Y., Xu, Y., Ji, H., et al. (2009). New azoles with potent antifungal activity: design, synthesis and molecular docking. Eur. J. Med. Chem. 44, 4218–4226. doi: 10.1016/j.ejmech.2009.05.018
Chen, S. C., Slavin, M. A., and Sorrel, T. C. (2011). Echinocandin antifungal drugs in fungal infection. Drugs 71, 11–41. doi: 10.2165/11585270-000000000-00000
Choudhary, M. I., Khan, N. T., Musharraf, S. G., Anjum, S., and Atta-ur-Rahman. (2007). Biotransformation of adrenosterone by filamentous fungus Cunninghamella elegans. Steroids 72, 923–929. doi: 10.1016/j.steroids.2007.08.002
Choudhary, M. I., Mohammad, M. Y., Musharraf, S. G., Parvez, M., Al-Aboudi, A., and Atta-ur-Rahman. (2009). New oxandrolone derivatives by biotransformation using Rhizopus stolonifera. Steroids 74, 1040–1044. doi: 10.1016/j.steroids.2009.08.003
Choudhary, M. I., Shah, S. A. A., Atta-ur-Rahman, Khan, S. N., and Khan, M. T. H. (2010). Alpha-glucosidase and tyrosinase inhibitors from fungal hydroxylation of tibolone and hydroxytibolones. Steroids 75, 956–966. doi: 10.1016/j.steroids.2010.05.017
Choudhary, M. I., Sultan, S., Jalil, S., Anjum, S., Rahman, A. A., Fun, H. K., et al. (2005). Microbial transformation of mesterolone. Chem. Biodivers. 2, 392–400. doi: 10.1002/cbdv.200590019
Choudhary, M. I., Yousuf, S., Samreen, Shah, S. A., Ahmed, S., and Atta-ur-Rahman (2006). Biotransformation of physalin H and leishmanicidal activity of its transformed products. Chem. Pharm. Bull. 54, 927–930. doi: 10.1248/cpb.54.927
Chung, S. K., Ryoo, C. H., Yang, H. W., Shim, J. Y., Kang, M. G., Lee, K. W., et al. (1998). Synthesis and bioactivities of steroid derivatives as antifungal agents. Tetrahedron 54, 15899–15914. doi: 10.1016/S0040-4020(98)01000-X
Claes, P., Cappoen, D., Mbala, B. M., Jacobs, J., Mertens, B., Mathys, V., et al. (2013). Synthesis and antimycobacterial activity of analogues of the bioactive natural products sampangine and cleistopholine. Eur. J. Med. Chem. 67, 98–110. doi: 10.1016/j.ejmech.2013.06.010
Crešnar, B., and Petrič, Š. (2011). Cytochrome P450 enzymes in the fungal kingdom. Biochim. Biophys. Acta 1814, 29–35. doi: 10.1016/j.bbapap.2010.06.020
De Andrés, C., Mercadé, E., Guinea, J., and Manresa, A. (1994). 7,10-Dihydroxy-8(E)-octadecenoic acid produced by Pseudomonas 42A2: evaluation of different cultural parameters of the fermentation. World J. Microbiol. Biotechnol. 10, 106–109.
Do, Q. T., Medina-Franco, J. L., Scior, T., and Bernard, P. (2015). How to valorize biodiversity? Let's go hashing, extracting, filtering, mining, fishing. Planta Med. 81, 436–449. doi: 10.1055/s-0034-1396314
Donova, M. V., and Egorova, O. V. (2012). Microbial steroid transformations: current state and prospects. Appl. Microbiol. Biotechnol. 94, 1423–1447. doi: 10.1007/s00253-012-4078-0
Ekins, S., Mestres, J., and Testa, B. (2007). In silico pharmacology for drug discovery: methods for virtual ligand screening and profiling. Br. J. Pharmacol. 152, 9–20. doi: 10.1038/sj.bjp.0707305
Erarslan, A., Terzi, I., Guray, A., and Bermek, E. (1990). Purification and kinetics of penicillin G acylase from a mutant strain of Escherichia coli ATCC 11105. J. Chem. Technol. Biotechnol. 51, 27–40. doi: 10.1002/jctb.280510103
Eschenauer, G. A., Nguyen, M. H., Shoham, S., Vazquez, J. A., Morris, A. J., Pasculle, W. A., et al. (2014). Real World Experience with echinocandin MICs against Candida species in a multicenter study of hospitals that routinely perform susceptibility testing of bloodstream isolates. Antimicrob. Agents Chemother. 58, 1897–1906. doi: 10.1128/AAC.02163-13
Farooq, A., Choudhary, M. I., Atta-ur-Rahman, Tahara, S., Başer, K. H., and Demirci, F. (2002). Detoxification of terpinolene by plant pathogenic fungus Botrytis cinerea. Naturforsch. 57, 303–306. doi: 10.1515/znc-2002-9-1018
Fu, S. B., Yang, J. S., Cui, J. L., Feng, X., and Sun, D. A. (2011). Biotransformation of ursolic acid by an endophytic fungus from medicinal plant Huperzia serrata. Chem. Pharm. Bull. 59, 1180–1182. doi: 10.1248/cpb.59.1180
Fura, A. (2006). Role of pharmacologically active metabolites in drug discovery and development. Drug Discover. Today 11, 133–142. doi: 10.1016/S1359-6446(05)03681-0
Galal, A. M. (2001). Microbial transformation of pyrethrosin. J. Nat. Prod. 64, 1098–1099. doi: 10.1021/np0100082
Gopkumar, P., and Mugeraya, G. (2010). Microbial transformation of bioactive natural products. Res. J. Pharm. Biol. Chem. Sci. 1, 826–831. doi: 10.1016/j.jare.2014.11.009
Guo, B., Wang, Y., Sun, X., and Tang, K. (2008). Bioactive natural products from endophytes: a review. Prikl. Biokhim. Mikrobiol. 44, 153–158. doi: 10.1134/s0003683808020026
Hegazy, M. E., Mohamed, T. A., ElShamy, A. I., Mohamed, A. E., Mahalel, U. A., Reda, E. H., et al. (2015). Microbial biotransformation as a tool for drug development based on natural products from mevalonic acid pathway: a review. Adv Res. 6, 17–33. doi: 10.1016/j.jare.2014.11.009
Holland, H. L. (1999). Recent advances in applied and mechanistic aspects of the enzymatic hydroxylation of steroids by whole-cell biocatalysts. Steroids 64, 178–186. doi: 10.1016/S0039-128X(98)00085-3
Hormigo, D., de la Mata, I., Acebal, C., and Arroyo, M. (2010). Immobilized aculeacin A acylase from Actinoplanes utahensis: characterization of a novel biocatalyst. Bioresour. Technol. 101, 4261–4268. doi: 10.1016/j.biortech.2010.01.117
Hosokawa, M., Hou, C. T., and Weisleder, D. (2003a). Bioconversion of n-3 and n-6 PUFA by Clavibacter sp. ALA2. J. Am. Oil Chem. Soc. 80, 1085–1091. doi: 10.1007/s11746-003-0824-8
Hosokawa, M., Hou, C. T., and Weisleder, D. (2003b). Production of novel tetrahydroxyfuranyl fatty acids from alpha-linolenic acid by Clavibacter sp. strain ALA2. Appl. Environ. Microbiol. 69, 3868–3873. doi: 10.1128/AEM.69.7.3868-3873.2003
Hou, C. T. (1996). A novel compound, 12,13,17-trihydroxy-9(Z)-octadecenoic acid, from linoleic acid by a new microbial isolate Clavibacter sp. ALA2. J. Am. Oil Chem. Soc. 73, 1359–1362. doi: 10.1007/BF02523497
Hou, C. T., Brown, W., Labeda, D. P., Abbott, T. P., and Weisleder, D. (1997). Microbial production of a novel trihydroxy unsaturated fatty acid from linoleic acid. J. Ind. Microbiol. Biotechnol. 19, 34–38. doi: 10.1038/sj.jim.2900414
Hou, C. T., and Forman, R. J. III. (2000). Growth inhibition of plant pathogenic fungi by hydroxy fatty acids. J. Ind. Microbiol. Biotechnol. 24, 275–276. doi: 10.1038/sj.jim.2900816
Huang, Q., An, H., Song, H., Mao, H., Shen, W., and Dong, J. (2015). Diversity and biotransformative potential of endophytic fungi associated with the medicinal plant Kadsura angustifolia. Res. Microbiol. 166, 45–55. doi: 10.1016/j.resmic.2014.12.004
Huang, Z., Cai, X., Shao, C., She, Z., Xia, X., Chen, Y., et al. (2008). Chemistry and weak antimicrobial activities of phomopsins produced by mangrove endophytic fungus Phomopsis sp. ZSU-H76. Phytochem. 69, 1604–1608. doi: 10.1016/j.phytochem.2008.02.002
İşcan, G., Kirimer, N., Demirci, F., Noma, Y., and Başer, K. H. (2012). Biotransformation of (-)-(R)-α-phellandrene: antimicrobial activity of its major metabolite. Chem. Biodivers. 9, 1525–1532. doi: 10.1002/cbdv.201100283
Joseph, B., and Priya, R. M. (2011). Bioactive compounds from endophytes and their potential in pharmaceutical effect. Am. J. Biochem. Mol. Biol. 1, 291–309. doi: 10.3923/ajbmb.2011.291.309
Kathiravan, M. K., Salake, A. B., Chothe, A. S., Dudhe, P. B., Watode, R. P., Mukta, M. S., et al. (2012). The biology and chemistry of antifungal agents: a review. Bioorg. Med. Chem. 20, 5678–5698. doi: 10.1016/j.bmc.2012.04.045
Keller, N. P., Turner, G., and Bennett, J. W. (2005). Fungal secondary metabolism – from biochemistry to genomics. Nat. Rev. Microbiol. 3, 937–947. doi: 10.1038/nrmicro1286
Khoyratty, S., Dupont, J., Lacoste, S., Palama, T. L., Choi, Y. H., Kim, H. K., et al. (2015). Fungal endophytes of Vanilla planifolia across Réunion Island: isolation, distribution and biotransformation. BMC Plant Biol. 15:142. doi: 10.1186/s12870-015-0522-5
Koh, H.-L., Yau, W.-P., Ong, P.-S., and Heqde, A. (2003). Current trends in modern pharmaceutical analysis for drug discovery. Drug Discover. Today 8, 889–897. doi: 10.1016/S1359-6446(03)02846-0
Kulkarni, S. A., Zhu, J., and Blechinger, S. (2005). In silico techniques for the study and prediction of xenobiotic metabolism: a review. Xenobiotica 35, 955–973. doi: 10.1080/00498250500354402
Kumagai, H., Umekita, M., Sawa, R., Takahashi, Y., Arisawa, A., Isshiki, K., et al. (2008). Generation of new benanomicin analogues by biotransformation using Escherichia coli expressing actinomycete cytochrome P450. J. Antibiot. 61, 394–399. doi: 10.1038/ja.2008.55
Kusari, S., Lamshöft, M., Zühlke, S., and Spiteller, M. (2008). An endophytic fungus from Hypericum perforatum that produces hyperecin. J. Nat. Prod. 71, 159–162. doi: 10.1021/np070669k
Kusari, S., and Spiteller, M. (2011). Are we ready for industrial production of bioactive plant secondary metabolites utilizing endophytes? Nat. Prod. Rep. 28, 1203–1207. doi: 10.1039/c1np00030f
Kusari, S., Zühlke, S., Kosuth, J., Cellárová, E., and Spiteller, M. (2009). Light-independent metabolomics of endophytic Thielavia subthermophila provides insight into microbial hypericin biosynthesis. J. Nat. Prod. 72, 1825–1835. doi: 10.1021/np9002977
Li, E., Jiang, L., Guo, L., Zhang, H., and Che, Y. (2008). Pestalachlorides A-C, antifungal metabolites from the plant endophytic fungus Pestalotiopsis adusta. Bioorg. Med. Chem. 16, 7894–7899. doi: 10.1016/j.bmc.2008.07.075
Liu, J. Y., Song, Y. C., Zhang, Z., Wang, L., Guo, Z. J., Zou, W. X., et al. (2004). Aspergillus fumigatus CY018, an endophytic fungus in Cynodon dactylon as a versatile producer of new and bioactive metabolites. J. Biotechnol. 114, 279–287. doi: 10.1016/j.jbiotec.2004.07.008
Liu, X., Dong, M., Chen, X., Jiang, M., Lv, X., and Zhou, J. (2008). Antimicrobial activity of an endophytic Xylaria sp. YX-28 and indentification of its antimicrobial compound 7-amino-4-meticoumarin. Appl. Microbiol. Biotechnol. 78, 241–247. doi: 10.1007/s00253-007-1305-1
Lu, S. E., Novak, J., Austin, F. W., Gu, G., Ellis, D., Kirk, M., et al. (2009). Occidiofungin, a unique antifungal glycopeptide produced by a strain of Burkholderia contaminans. Biochemistry 48, 8312–8321. doi: 10.1021/bi900814c
Maehara, S., Ikeda, M., Haraguchi, H., Kitamura, C., Nagoe, T., Ohashi, K., et al. (2011). Microbial conversion of curcumin into colorless hydroderivatives by the endophytic fungus Diaporthe sp. associated with Curcuma longa. Chem. Pharm. Bull. 59, 1042–1044. doi: 10.1248/cpb.59.1042
Mahato, S. B., and Garai, S. (1997). Advances in microbial steroid biotransformation. Steroids 62, 332–345. doi: 10.1016/S0039-128X(96)00251-6
Mahato, S. B., and Mukherjee, A. (1984). Steroid transformations by microorgamisms. Phytochemistry 23, 2131–2154. doi: 10.1016/S0031-9422(00)80509-9
Malaviya, A., and Gomes, J. (2008). Androstenedione production by biotransformation of phytosterols. Bioresour. Technol. 99, 6725–6737. doi: 10.1016/j.biortech.2008.01.039
Martin-Arjol, I., Bassas-Galia, M., Bermudo, E., Garcia, F., and Manresa, A. (2010). Identification of oxylipins with antifungal activity by LC-MS/MS from the supernatant of Pseudomonas 42A2. Chem. Phys. Lipids 163, 341–346. doi: 10.1016/j.chemphyslip.2010.02.003
Masui, H., Kondo, T., and Kojima, M. (1989). An antifungal compound, 9,12,13-trihydroxy-(E)-octadecenoic acid, from Colocasia antiquorum inoculated with Ceratocytis fimbriata. Phytochemistry 28, 2613–2615. doi: 10.1016/S0031-9422(00)98051-8
Molina, G., Pimentel, M. R., Bertucci, T. C. P., and Pastore, G. M. (2012). Application of fungal endophytes in biotechnological processes. Chem. Eng. Tran. 27, 289–294.
Mujawar, S. K. (1999). D-amino acid oxidase: its potential in the production of 7-aminocephalosporanic acid. Hindustan. Antibiot. Bull. 41, 1–14.
Muñoz Solano, D., Hoyos, P., Hernáiz, M. J., Alcántara, A. R., and Sánchez-Montero, J. M. (2012). Industrial biotransformation in the synthesis of building blocks leading to enantiopure drugs. Bioresource Technol. 115, 196–207. doi: 10.1016/j.biortech.2011.11.131
Murphy, C. D. (2012). The microbial cell factory. Org. Biomol. Chem. 10, 1949–1957. doi: 10.1039/c2ob06903b
Newman, D. J., and Cragg, G. M. (2012). Natural products as sources of new drugs over the 30 years from 1981 to 2010. J. Nat. Prod. 75, 311–335. doi: 10.1021/np200906s
Odds, F. C. (2003). Antifungal agents: their diversity and increasing sophistication. Mycologist 17, 51–55. doi: 10.1017/S0269915X03002064
Omar, M. N., Hasali, N. H. M., Alfarra, H. Y., Yarmo, M. A., and Zuberdi, A. M. (2014). Antimicrobial activity and microbial transformation of ethyl p-methoxycinnamate extracted from Kaempferia galanga. Orient. J. Chem. 30, 1037–1043. doi: 10.13005/ojc/300315
Orabi, K. Y., Clark, A. M., and Hufford, C. D. (2000). Microbial transformation of benzosampangine. J. Nat. Prod. 63, 396–398. doi: 10.1021/np990493p
Orabi, K. Y., Li, E., Clark, A. M., and Hufford, C. D. (1999). Microbial transformation of sampangine. J. Nat. Prod. 62, 988–992. doi: 10.1021/np980457a
Park, J. H., Choi, G. J., Lee, H. B., Kim, K. M., Jung, H. S., Lee, S. W., et al. (2005). Griseofulvin from Xylaria sp. strain F0010, and endophytic fungus of Abies holophylla and its antifungal activity against plant pathogenic fungi. J. Microbiol. Biotechnol. 15, 112–117.
Park, J. W., Park, S. R., Han, A. R., Ban, Y. H., Yoo, Y. J., Kim, E. J., et al. (2011). Microbial transformation of trichostatin A to 2,3-dihydrotrichostatin A. J. Nat. Prod. 74, 1272–1274. doi: 10.1021/np1006718
Parshikov, I. A., Freeman, J. P., Lay, J. O. Jr. Beger, R. D., Williams, A. J., and Sutherland, J. B. (2000). Microbiological transformation of enrofloxacin by the fungus Mucor ramannianus. Appl. Environ. Microbiol. 66, 2664–2667.
Perfect, J. R., Hachem, R., and Wingard, J. R. (2014). Update on epidemiology of and preventive strategies for invasive fungal infections in cancer patients. Clin. Infect. Dis. 59, 352–355. doi: 10.1093/cid/ciu639
Pervaiz, I., Ahmad, S., Madni, M. A., Ahmad, H., and Khaliq, F. H. (2013). Microbial biotransformation: a tool for drug designing. Appl. Biochem. Micro. 49, 437–450. doi: 10.1134/S0003683813050098
Pimentel, M. R., Molina, G., Dionísio, A. P., Maróstica, M. R. Jr., and Pastore, G. M. (2011). The use of endophytes to obtain bioactive compounds and their application in biotransformation process. Biotechnol. Res. Int. 2011:576286. doi: 10.4061/2011/576286
Pongcharoen, W., Rukachaisirikul, V., Phongpaichit, S., Kühn, T., Pelzing, M., Sakayaroj, J., et al. (2008). Metabolites from the endophytic fungus Xylaria sp. PSU-D14. Phytochemistry 69, 1900–1902. doi: 10.1016/j.phytochem.2008.04.003
Qin, J. C., Zhang, Y. M., Gao, J. M., Bai, M. S., Yang, S. X., Laatsch, H., et al. (2009). Bioactive metabolites produced by Chaetomium globosum, an endophytic fungus isolated from Ginkgo biloba. Bioorg. Med. Chem. Lett. 19, 1572–1574. doi: 10.1016/j.bmcl.2009.02.025
Raja, A., and Prabakarana, P. (2011). Actinomycetes and drug – an overview. Am. J. Drug Disc. Dev. 1, 75–84. doi: 10.3923/ajdd.2011.75.84
Rajendran, K., Mahadevan, S., Sekar, S., Paramasamy, G., and Mandal, A. B. (2011). Biocalorimetric and respirometric studies on production of Penicillin G acylase from Bacillus badius pac in E. coli DH5 alpha. Biochem. Eng. J. 55, 223–229. doi: 10.1016/j.bej.2011.05.003
Rask-Andersen, M., Almén, M. S., and Schiöth, H. B. (2011). Trends in the exploitation of novel drug targets. Nat. Rev. Drug. Discovery 10, 579–590. doi: 10.1038/nrd3478
Restaino, O. F., Marseglia, M., De Castro, C., Diana, P., Forni, P., Parrilli, M., et al. (2014). Biotechnological transformation of hydrocortisone to 16α-hydroxy hydrocortisone by Streptomyces roseochromogenes. Appl. Microbiol. Biotechnol. 98, 1291–1299. doi: 10.1007/s00253-013-5384-x
Ruiz, B., Chávez, A., Forero, A., García-Huante, Y., Romero, A., Sánchez, M., et al. (2010). Production of microbial secondary metabolites: regulation by the carbon source. Crit. Rev. Microbiol. 36, 146–167. doi: 10.3109/10408410903489576
Russell, W. M. S., and Burch, R. L. (1959). The Principles of Humane Experimental Technique. London: Methuen.
Schmitz, A., Felder, S., Höver, T., Kehraus, S., Neu, E., Lohr, F., et al. (2013). Antibiotics from gliding bacteria. Phytochem. Rev. 12, 507–516. doi: 10.1007/s11101-012-9224-x
Schulz, B., Sucker, J., Aust, H. J., Krohn, K., Ludewig, K., Jones, P. G., et al. (1995). Biologically active secondary metabolites of endophytic Pezicula species. Mycol. Res. 99, 1007–1015. doi: 10.1016/S0953-7562(09)80766-1
Shafiee, A., Harris, G. H., Zink, D. L., Sigmund, J. M., Rosenbach, M. J., and Mandala, S. M. (1999). Microbial Transformation Products with Antifungal Properties. US Patent No 5, 972, 994. Washington, DC: Merck & Co., Inc.
Shibuya, H., Agusta, A., Ohashi, K., Maehara, S., and Simanjuntak, P. (2005). Biooxidation of (+)-catechin and (-)-epicatechin into 3,4-dihydroxyflavan derivatives by the endophytic fungus Diaporthe sp. isolated from a tea plant. Chem. Pharm. Bull. 53, 866–867. doi: 10.1248/cpb.53.866
Shibuya, H., Kitamura, C., Maehara, S., Nagahata, M., Winarno, H., Simanjuntak, P., et al. (2003). Transformation of Cinchona alkaloids into 1-N-oxide derivatives by endophytic Xylaria sp isolated from Cinchona pubescens. Chem. Pharm. Bull. 51, 71–74. doi: 10.1248/cpb.51.71
Silva, G. H., Teles, H. L., Trevisan, H. C., Bolzani, V. S., Young, M. C. M., Pfenning, L. H., et al. (2005). New bioactive metabolites produced by Phomopsis cassia, an endophytic fungus in Cassia spectabilis. J. Braz. Chem. Soc. 16, 1463–1466. doi: 10.1590/S0103-50532005000800029
Srirangan, K., Orr, V., Akawi, L., Westbrook, A., Moo-Young, M., and Chou, C. P. (2013). Biotechnological advances on penicillin G acylase: pharmaceutical implications, unique expression mechanism and production strategies. Biotechnol. Adv. 31, 1319–1332. doi: 10.1016/j.biotechadv.2013.05.006
Takimoto, A., Takakura, T., Tani, H., Yagi, S., and Mitsushima, K. (2004). Batch production of deacetyl 7-aminocephalosporanic acid by immobilized cephalosporin-C deacetylase. Appl. Microbiol. Biotechnol. 65, 263–267. doi: 10.1007/s00253-004-1595-5
Tan, W., Cooley, J., Austin, F., Lu, S. E., Pruett, S. B., and Smith, L. (2012). Nonclinical toxicological evaluation of occidiofungin, a unique glycolipopeptide antifungal. Int. J. Toxicol. 31, 326–336. doi: 10.1177/1091581812445185
Tucker, J. L. (2006). Green chemistry, a pharmaceutical perspective. Org. Process. Res. Dev. 10, 315–319. doi: 10.1021/op050227k
Venisetty, R. K., and Ciddi, V. (2003). Application of microbial biotransformation for the new drug discovery using natural drugs as substrates. Curr. Pharm. Biotechnol. 4, 153–167. doi: 10.2174/1389201033489847
Wang, F. W., Jiao, R. H., Cheng, A. B., Tan, S. H., and Song, Y. C. (2007). Antimicrobial potentials of endophytic fungi residing in Quercus variabilitis and brefeldin A obtained from Cladosporium sp. World J. Microbiol. Biotechnol. 23, 79–83. doi: 10.1007/s11274-006-9195-4
Wild, H. (1994). Enantioselective total synthesis of the antifungal natural products chlorotetaine, bacilysin, and anticapsin and of related compounds: revision of the relative configuration. J. Org. Chem. 59, 2748–2761. doi: 10.1021/jo00089a019
Wu, C., Zacchetti, B., Ram, A. F., van Wezel, G. P., Claessen, D., and Hae Choi, Y. (2015). Expanding the chemical space for natural products by Aspergillus-Streptomyces co-cultivation and biotransformation. Sci. Rep. 5:10868. doi: 10.1038/srep10868
Wu, Q., Liu, B. K., and Lin, X. F. (2010). Enzymatic promiscuity for organic synthesis and organic cascade process. Curr. Org. Chem. 14, 1966–1988. doi: 10.2174/138527210792927591
You, F., Han, T., Wu, J. Z., Huang, B. K., and Qin, L. P. (2009). Antifungal secondary metabolites from endophytic Verticillium sp. Biochem. Syst. Ecol. 37, 162–165. doi: 10.1016/j.bse.2009.03.008
Yu, H., Zhang, L., Li, L., Zheng, C., Guo, L., Li, W., et al. (2010). Recent developments and future prospects of antimicrobial metabolites produced by endophytes. Microbiol. Res. 165, 437–449. doi: 10.1016/j.micres.2009.11.009
Zaks, A., and Dodds, D. R. (1997). Application of biocatalysis and biotransformations to the synthesis of pharmaceuticals. Drug Discover. Today 2, 513–531. doi: 10.1016/S1359-6446(97)01078-7
Keywords: microbial biotransformation, biocatalysis, bioconversion, metabolism, antifungals
Citation: Bianchini LF, Arruda MFC, Vieira SR, Campelo PMS, Grégio AMT and Rosa EAR (2015) Microbial Biotransformation to Obtain New Antifungals. Front. Microbiol. 6:1433. doi: 10.3389/fmicb.2015.01433
Received: 31 August 2015; Accepted: 01 December 2015;
Published: 24 December 2015.
Edited by:
Helen Zgurskaya, University of Oklahoma, USAReviewed by:
Jon Y. Takemoto, Utah State University, USAPolpass Arul Jose, Central Salt and Marine Chemicals Research Institute, India
Copyright © 2015 Bianchini, Arruda, Vieira, Campelo, Grégio and Rosa. This is an open-access article distributed under the terms of the Creative Commons Attribution License (CC BY). The use, distribution or reproduction in other forums is permitted, provided the original author(s) or licensor are credited and that the original publication in this journal is cited, in accordance with accepted academic practice. No use, distribution or reproduction is permitted which does not comply with these terms.
*Correspondence: Edvaldo A. R. Rosa, edvaldo.rosa@pucpr.br