Spatiotemporal Features of Retinal Waves Instruct the Wiring of the Visual Circuitry
- 1Department of Molecular and Cell Biology, University of California Berkeley, Berkeley, CA, USA
- 2Helen Wills Neuroscience Institute, University of California Berkeley, Berkeley, CA, USA
Coordinated spontaneous activity is present in different sensory systems during early stages of development. This activity is thought to play a critical role in the development of sensory representations before the maturation of sensory experience. In the visual system, the mechanisms by which spatiotemporal properties of retinal spontaneous activity, called retinal waves, drive developmental events has been well studied. Recent advancements in pharmacological, genetic, and optogenetic manipulations have provided further understanding of the contribution of specific spatiotemporal properties of retinal waves to eye-specific segregation and retinotopic refinement of retinofugal projections. Here we review some of the recent progress in understanding the role of retinal waves in the early stages of visual system development, prior to the maturation of vision.
Introduction
The cellular and molecular mechanisms underlying the spatiotemporal properties ofs retinal waves are well understood (Blankenship and Feller, 2010; Kerschensteiner, 2013). There are three developmental stages of waves mediated by different forms of neurotransmission; stage 1 waves are mediated by a combination of gap junctions and cholinergic circuits (Bansal et al., 2000), stage 2 waves are cholinergic (Feller et al., 1996), and stage 3 waves are glutamatergic (Wong et al., 2000). All three stages generate waves with different spatiotemporal properties (Maccione et al., 2014), as determined in vitro. Waves are characterized by their speed, frequency, covered area (size), within-burst spiking frequency, intra-retina distance-dependent correlations, and inter-eye correlations. More recently it has been established that the spatiotemporal properties of stage 2 retinal waves are preserved in vivo and are synaptically propagated to central visual targets (Colonnese and Khazipov, 2010; Ackman et al., 2012).
It is widely accepted that retinal waves are critical for the refinement of visual maps in retinofugal targets. Retinal projections to the superior colliculus (SC) and the dorsal lateral geniculate nucleus (dLGN) are retinotopically organized and segregate into eye-specific regions in a manner dependent on retinal activity (Figures 1, 2A; Wong, 1999; Bansal et al., 2000; Huberman et al., 2008). A classic debate in this field has been whether retinal waves are permissive or instructive for driving these developmental processes (Crair, 1999; Chalupa, 2009; Feller, 2009; Maccione et al., 2014). If waves are permissive, it implies that a minimum level of activity is required for some other instructive process to drive segregation, such as gene regulation. If waves are instructive, it implies that information about the target map is contained within the pattern of action potential firing during retinal waves. However, manipulations that alter the pattern of retinal waves also change their overall firing levels, making it difficult to differentiate between instructive and permissive roles. Here we review recent efforts that have used novel manipulations, in both mouse and ferret, to restrict perturbations of specific spatiotemporal properties of waves and thus advance the understanding of their contribution to the development of the visual circuitry.
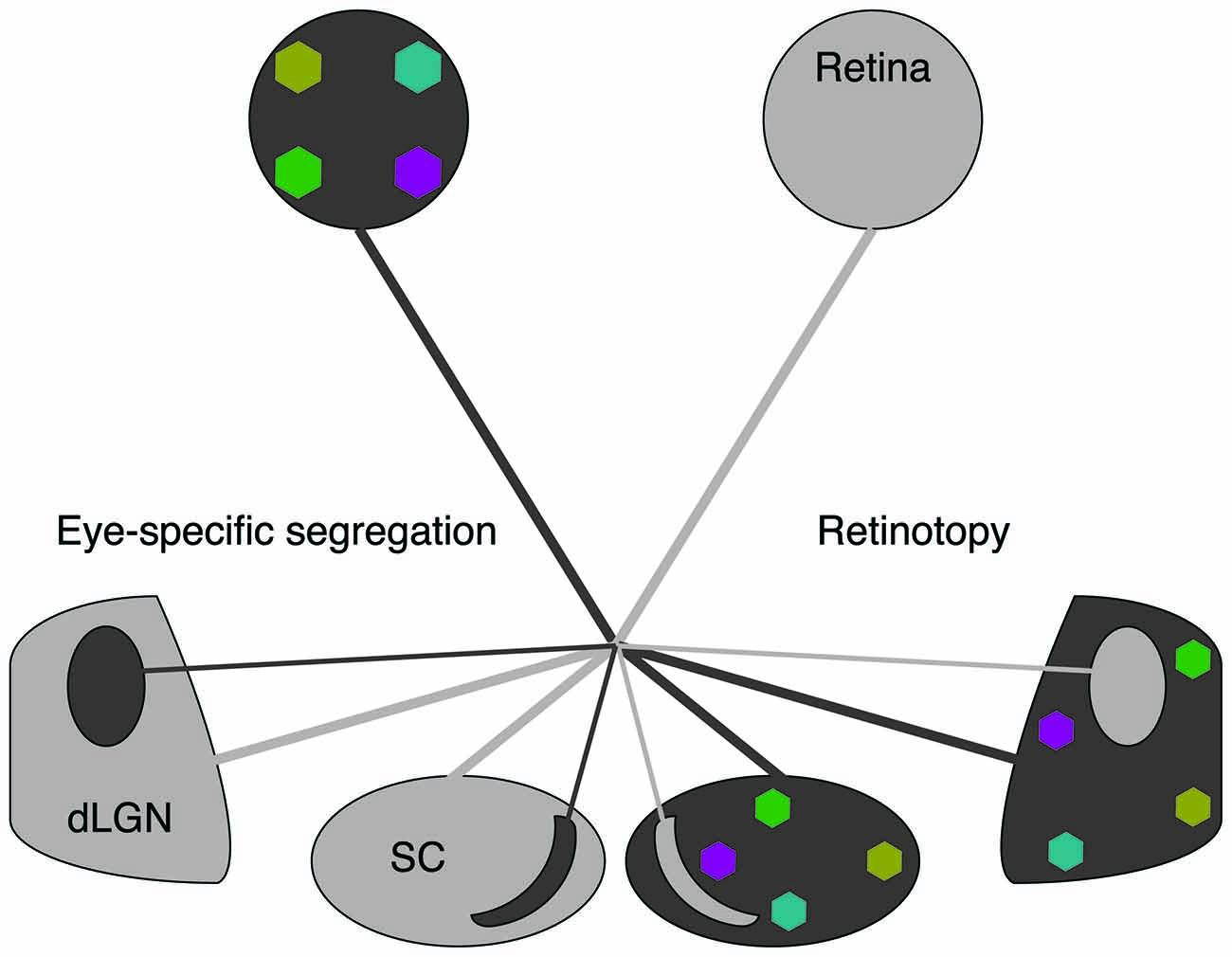
Figure 1. Spontaneous retinal waves mediate eye-specific segregation and retinotopic refinement of retinofugal projections. The axons of retinal ganglion cells (RGCs) target the dorsal lateral geniculate nucleus (dLGN) of the thalamus and the superior colliculus (SC). RGC projections from opposite eyes are segregated into ipsilateral and contralateral regions (black/gray oppositions). RGC projections from each retina are retinotopically organized (colored regions). Note that for simplicity we have depicted retinotopic maps only for one eye. Retinotopic maps and eye-specific segregations form for both eyes.
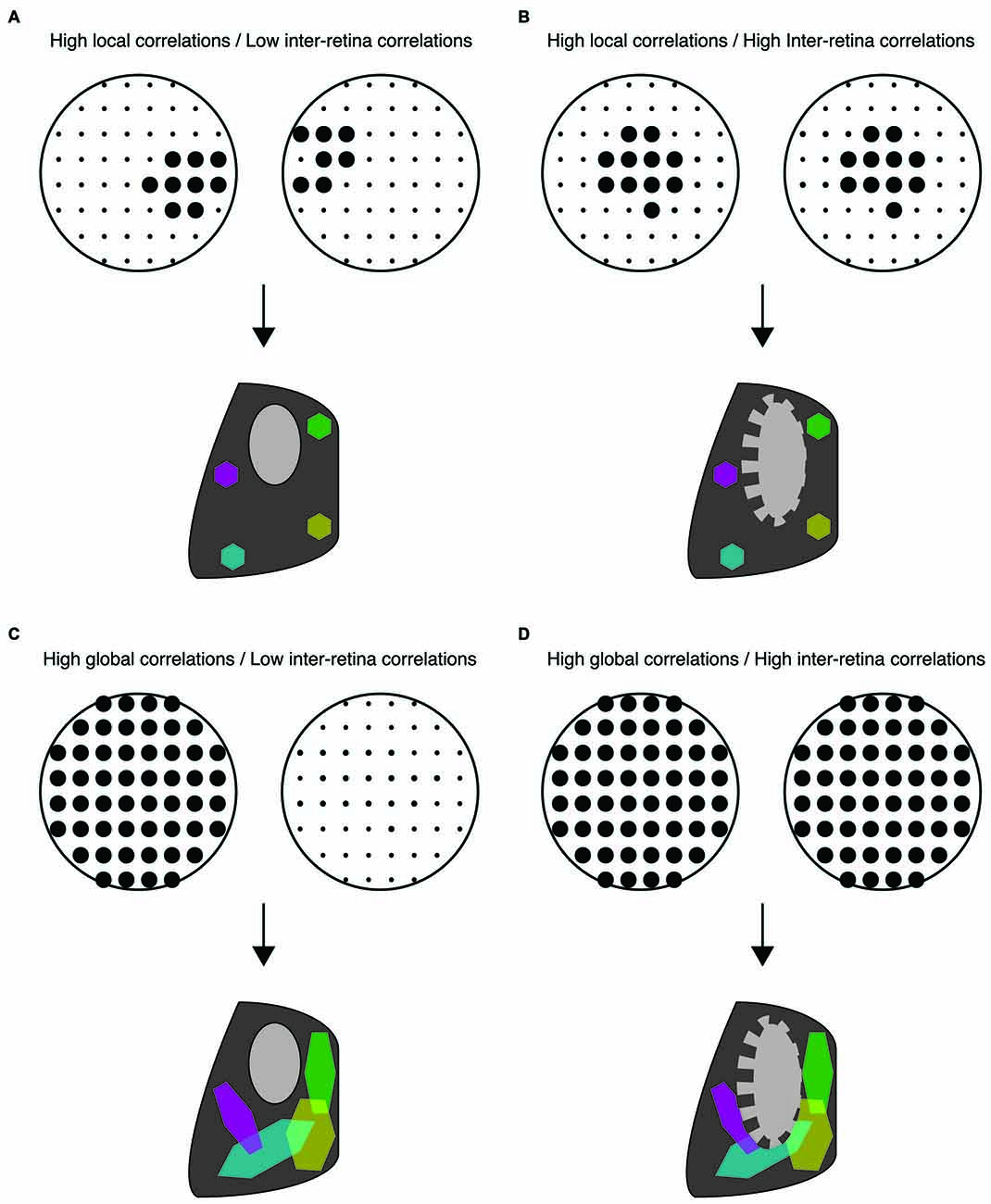
Figure 2. Distinct spatiotemporal patterns of retinal waves instruct different features of retinofugal projections in mice. (A–D) Top: schematic of retinal wave firing patterns. Dots are representative of RGCs with larger dots indicating elevated firing rates during a single retinal wave. Bottom: schematic of retinogeniculate wiring using same code as Figure 1—colored hexagons represent retinotopy while gray/black regions represent eye-specific segregation. (A) Under normal conditions, RGCs exhibit high local correlations while activity of the two retinas is minimally correlated. This pattern of activity supports both eye-specific segregation and retinotopic maps in both the dLGN and SC. (B) High correlations between retinal waves of opposite retinas induced by optogenetic stimulation is detrimental to eye-specific segregation while retinotopic maps are unaffected (Zhang et al., 2011). (C) Disruption of local correlations either by an increase in uncorrelated firing or by abnormally elevated correlations between distant RGCs (global correlations), such as that observed in β2KO and Retβ2-cKO mice, is detrimental to retinotopic map formation. Local correlations are sufficient for normal retinotopic maps in Retβ2-cKO, Rxβ2-cKO and β2(TG) (Xu et al., 2011, 2015; Burbridge et al., 2014). (D) High global correlations paired with high inter-retina correlations such as that observed in the β2KO mouse, is detrimental to both eye-specific segregation and retinotopic maps (Xu et al., 2011; Burbridge et al., 2014).
Inter-Eye Competition Instructs Eye-Specific Segregation
Inter-eye competition generated by spontaneous retinal waves was hypothesized to be instructive for eye-specific segregation of retinofugal projections (Butts et al., 2007). During retinal waves, individual retinal ganglion cells (RGCs) fire short bursts of action potentials that propagate to neighboring RGCs within a well defined region and are separated by 1–2 min of silence—hence the firing within retinotopically identified regions of the retina have stronger intra-retinal correlation than inter-retinal correlations. One of the unexpected findings of in vivo calcium imaging used to record activity of RGC terminals projecting to the SC was that activity of the two eyes is more correlated than would be expected by chance (Ackman et al., 2012). However, this correlation is weak and most waves occur independently.
The β2KO mouse is a classic model for studying the role of retinal waves in visual system development. This mouse model lacks the β2 subunit of the nicotinic acetylcholine receptor (β2-nAChR), which is required for cholinergic waves. This mouse has significantly impaired eye-specific segregation and retinotopic refinement of retinal projects to the dLGN of the thalamus and the SC. Unfortunately, there are several conflicting descriptions of β2KO retinal activity in vitro, with different spatiotemporal properties reported based on recording conditions such as temperature and media used (McLaughlin et al., 2003; Sun et al., 2008; Kirkby and Feller, 2013). In contrast, sensitivity to temperature is not observed in waves of wild type (WT) mice (Stafford et al., 2009). Recent in vivo imaging of RGC axon terminals in the SC of β2KO revealed waves to be infrequent but dramatically larger and faster than waves in WT mice, and were described by the authors as “flashes” (Burbridge et al., 2014). Importantly, depolarizations induced by RGCs in β2KO mice were not sufficient to entrain postsynaptic SC neurons, which exhibited an activity pattern different from that of RGC axons. Hence β2KO have strong long-range intra-retinal correlations but weak and infrequent activity. Moreover, when flashes occur, they are likely to be correlated between the two eyes, indicating stronger inter-retinal correlations than those observed in WT (Burbridge et al., 2014).
Though these studies are consistent with the hypothesis that a lack of correlated activity between opposing retinas is important for eye-specific segregation (Figure 2B), they do not rule out the possibility that impaired map refinement in β2KO mice is due to an overall reduction in retinal activity. Recent studies have separated out the contributions of an overall decrease in retinal activity, the increase in inter-retinal correlations, and the disruption of the slowly propagating waves that drive local intra-retinal correlations. First, the frequency of waves is increased in the β2KO via intraocular injection of CP-cAMP (Burbridge et al., 2014), a manipulation known to increase the frequency of retinal waves in vitro (Stellwagen et al., 1999; Stellwagen and Shatz, 2002). Increasing wave frequency and thus overall wave activity improves eye-specific segregation in β2KO. Second, both correlated and anticorrelated inter-retinal activity were induced using optogenetic manipulations (Zhang et al., 2011). In these studies, correlated stimulation of the two retinas induced impaired eye-specific segregation while anticorrelated stimulation permitted eye-specific segregation and even partially rescued it in β2KO mice. These results were complemented by studies based on a transgenic mouse in which β2-nAChR subunits are knocked out exclusively from the retina starting early in development (Rx-β2cKO mice). In these mice local intra-retinal correlation properties of neighboring RGCs were largely maintained, but RGCs fired at low levels. This decrease in activity resulted in impaired eye specific segregation (Xu et al., 2015). Together, these studies strongly implicate that large-scale asynchronous activity between opposite eyes instructs eye-specific segregation while the local intra-retinal correlations induced by slowly propagating activity do not (Figures 2B,C).
Ferrets provide an interesting comparison to mice for assessing whether retinal waves are instructive for eye-specific segregation. Like mice, significant disruption of stage 2 cholinergic retinal waves disrupts eye-specific segregation of retinogeniculate afferents (Huberman et al., 2008). In contrast to mice, ipsilateral and contralateral retinal afferents of ferrets project to distinct cellular laminae in the dLGN, which may indicate differences in the mechanisms underlying visual map refinement between the two species. Using an immunotoxin to ablate starburst amacrine cells, a key component for generating Stage 2 retinal waves, Speer et al. (2014) were able to disrupt both local and global features of retinal waves while maintaining eye-specific segregation, similar to a previous study (Huberman et al., 2003). In contrast to mice, they found that large scale firing and the overall level of activity were not instructive, instead, the amount of uncorrelated firing exhibited by RGCs during the inter-wave periods was detrimental to eye-specific segregation.
A new insight into how waves influence eye-specific segregation in ferrets was achieved using an enucleated ferret model (Failor et al., 2015). Monocular enucleation results in expanded ipsilateral projections in the dLGN. Pharmacologically blocking waves in the remaining eye reduces this expansion and causes abnormal fragmentation of the ipsilateral laminae. To a lesser degree, blocking waves also induces fragmentation of contralateral projections in monocularly enucleated animals. This study suggests that intra-retinal activity itself plays a role in the development of eye-specific segregation that is independent of inter-eye competition. Furthermore, single-unit spike recordings from dLGN cells showed that blocking waves in monocularly enucleated animals causes abnormal enlargement and misalignment of receptive fields, thereby matching functional connectivity of the retino-geniculate projections with the observed anatomical phenotypes.
Together, these studies indicate that in ferrets intra-retinal correlations may be more critical for targeting axons to the correct layers and may also influence eye-specific segregation, in contrast with the observations in mice (Figures 2B,D).
Local Intra-Retinal Correlations Instruct Retinotopy
In contrast to eye-specific segregation, local intra-retinal correlations induced by propagating activity are thought to be the primary feature instructing retinotopic refinement. During locally propagating activity, nearby RGCs are more correlated than distant RGCs and therefore information about retinotopy is contained within the retinal wave firing pattern (Eglen et al., 2003). The process of retinotopic refinement has been studied primarily in retinocollicular projections, though recent progress in understanding the role of waves in retinotopic refinement of retinogeniculate projections has also been made.
Several of the mouse models described above have also identified key features of retinal waves that instruct retinotopic refinement. For example, restoring the overall level of retinal activity in β2KO mice using optogenetics or intraocular injections of CP-cAMP rescued impairments in eye-specific segregation, but not retinotopy (Zhang et al., 2011; Burbridge et al., 2014; Figure 2D), suggesting that local intra-retinal correlations rather than overall retinal activity instruct retinotopy. One of the most striking mouse models lacks β2-nAChR in the nasal and temporal retina but expression is maintained along the central dorso-ventral strip (Retβ2-cKO), which serves as an internal control (Burbridge et al., 2014). In vivo calcium imaging of retinocollicular axon terminals confirmed that these mice exhibit locally propagating waves in the central retina while waves are absent in the nasal and temporal portions of the retina. Retinocollicular projections emerging from temporal or nasal retina were diffuse, indicating a lack of retinotopic refinement, while projections emerging from central retina were normal. This finding indicates that local intra-retinal activity patterns play a central role in establishing retinotopy (Figure 2C).
The Rx-β2cKO retina discussed earlier exhibits lower overall activity, smaller waves with high local correlations, more variable inter-wave intervals, and lower wave amplitude as measured with calcium imaging. Rx-β2cKO mice have disrupted eye-specific segregation and normal retinotopy in the monocular zones, indicating that the restricted local propagation of waves is sufficient for retinotopic refinement. Interestingly, retinotopy of the binocular zones was largely impaired, indicating interdependence between eye-specific segregation and retinotopy in the binocular zone (Xu et al., 2015). A careful comparison of waves spatiotemporal patterns in various knockout models is discussed in this study.
The role of waves in the retinotopic refinement of retinogeniculate projections in ferrets has also seen recent advancements. Intraocular blockade of inhibition to enhance glutamatergic stage 3 retinal waves led to abnormally early retinotopic refinement of retinogeniculate projections, as measured anatomically and functionally (Davis et al., 2015). Based on in vitro experiments, blockade of inhibition increases the frequency of glutamatergic waves while maintaining local correlations (Kerschensteiner and Wong, 2008; Firl et al., 2013). These findings are rather surprising since it suggests that increased spontaneous activity prior to visual experience could potentially compensate for lack of visual experience.
It is important to note that retinal waves work in concert with molecular cues for establishing retinotopic maps (Pfeiffenberger et al., 2006; Cang et al., 2008; Ackman and Crair, 2014; Assali et al., 2014). Though recent progress in this field is too vast to review here, we want to make note of a recent study that implicates a role for retinal waves in establishing heterogeneity in visual map organization not only across animals but also across the two colliculi of a single animal. The study makes a strong case that spontaneous activity and molecular cues interact stochastically to form well-defined yet variable retinotopic maps (Owens et al., 2015).
In summary, the evidence suggests that in mice, overall activity levels and inter-eye competition are determinant for eye-specific segregation while local intra-retinal correlations determine retinotopy, with some interdependence of the two in the binocular zones of central retinal targets. In ferrets, there is additional evidence that local intra-retinal correlations also influence eye-specific segregation.
Emerging Roles of Retinal Waves in the Development of the Visual System
Most of the research on how retinal waves influence visual system development has been focused on retinofugal projections. However, there is also an interest in understanding whether retinal waves drive other aspects of visual system development. Recent studies have elucidated emerging roles for retinal waves in the development of visual circuits. In one study it was found that disrupting retinal waves increased cortical neurogenesis in the primary visual cortex while enhancing waves decreased neurogenesis (Bonetti and Surace, 2010). Another study found that eliminating the input of retinal waves to the SC resulted in migration and connectivity deficits in inhibitory interneurons of the dLGN (Golding et al., 2014). It has also been shown that elimination of retinal inputs to central targets accelerates the cortical innervation of the dLGN, yet synapse maturation was unaffected (Seabrook et al., 2013). In addition, computational models have implicated slow features of retinal waves in the establishment of complex cells in primary visual cortex (Dähne et al., 2014), and in the establishment of initial biases in orientation maps in primary visual cortex (Hagihara et al., 2015). These discoveries suggest a broad involvement of developmental spontaneous activity in cell signaling processes that mediate development, differentiation, integration, and connectivity of neural circuits.
The refinement of strategies to precisely control neural activity in vivo, as well as a deeper understanding of the molecular mechanisms linking retinal waves to various developmental processes will continue to provide insights onto the various roles of spontaneous activity patterns in circuit development.
Author Contributions
Both authors contributed to the writing of the manuscript. All authors listed, have made substantial, direct and intellectual contribution to the work, and approved it for publication.
Funding
This work was supported by the National Institutes of Health Grants R01EY013528 to MBF and Grant F31EY024842 to DAA.
Conflict of Interest Statement
The authors declare that the research was conducted in the absence of any commercial or financial relationships that could be construed as a potential conflict of interest.
References
Ackman, J. B., Burbridge, T. J., and Crair, M. C. (2012). Retinal waves coordinate patterned activity throughout the developing visual system. Nature 490, 219–225. doi: 10.1038/nature11529
Ackman, J. B., and Crair, M. C. (2014). Role of emergent neural activity in visual map development. Curr. Opin. Neurobiol. 24, 166–175. doi: 10.1016/j.conb.2013.11.011
Assali, A., Gaspar, P., and Rebsam, A. (2014). Activity dependent mechanisms of visual map formation–from retinal waves to molecular regulators. Semin. Cell Dev. Biol. 35, 136–146. doi: 10.1016/j.semcdb.2014.08.008
Bansal, A., Singer, J. H., Hwang, B. J., Xu, W., Beaudet, A., and Feller, M. B. (2000). Mice lacking specific nicotinic acetylcholine receptor subunits exhibit dramatically altered spontaneous activity patterns and reveal a limited role for retinal waves in forming ON and OFF circuits in the inner retina. J. Neurosci. 20, 7672–7681.
Blankenship, A. G., and Feller, M. B. (2010). Mechanisms underlying spontaneous patterned activity in developing neural circuits. Nat. Rev. Neurosci. 11, 18–29. doi: 10.1038/nrn2759
Bonetti, C., and Surace, E. M. (2010). Mouse embryonic retina delivers information controlling cortical neurogenesis. PLoS One 5:e15211. doi: 10.1371/journal.pone.0015211
Burbridge, T. J., Xu, H.-P., Ackman, J. B., Ge, X., Zhang, Y., Ye, M.-J., et al. (2014). Visual circuit development requires patterned activity mediated by retinal acetylcholine receptors. Neuron 84, 1049–1064. doi: 10.1016/j.neuron.2014.10.051
Butts, D. A., Kanold, P. O., and Shatz, C. J. (2007). A burst-based “Hebbian” learning rule at retinogeniculate synapses links retinal waves to activity-dependent refinement. PLoS Biol. 5:e61. doi: 10.1371/journal.pbio.0050061
Cang, J., Wang, L., Stryker, M. P., and Feldheim, D. A. (2008). Roles of ephrin-as and structured activity in the development of functional maps in the superior colliculus. J. Neurosci. 28, 11015–11023. doi: 10.1523/JNEUROSCI.2478-08.2008
Chalupa, L. M. (2009). Retinal waves are unlikely to instruct the formation of eye-specific retinogeniculate projections. Neural Dev. 4:25. doi: 10.1186/1749-8104-4-25
Colonnese, M. T., and Khazipov, R. (2010). “Slow activity transients” in infant rat visual cortex: a spreading synchronous oscillation patterned by retinal waves. J. Neurosci. 30, 4325–4337. doi: 10.1523/JNEUROSCI.4995-09.2010
Crair, M. C. (1999). Neuronal activity during development: permissive or instructive? Curr. Opin. Neurobiol. 9, 88–93. doi: 10.1016/s0959-4388(99)80011-7
Dähne, S., Wilbert, N., and Wiskott, L. (2014). Slow feature analysis on retinal waves leads to V1 complex cells. PLoS Comput. Biol. 10:e1003564. doi: 10.1371/journal.pcbi.1003564
Davis, Z. W., Chapman, B., and Cheng, H.-J. (2015). Increasing spontaneous retinal activity before eye opening accelerates the development of geniculate receptive fields. J. Neurosci. 35, 14612–14623. doi: 10.1523/JNEUROSCI.1365-15.2015
Eglen, S. J., Demas, J., and Wong, R. O. L. (2003). Mapping by waves. Patterned spontaneous activity regulates retinotopic map refinement. Neuron 40, 1053–1055. doi: 10.1016/S0896-6273(03)00808-0
Failor, S., Chapman, B., and Cheng, H.-J. (2015). Retinal waves regulate afferent terminal targeting in the early visual pathway. Proc. Natl. Acad. Sci. U S A 112, E2957–E2966. doi: 10.1073/pnas.1506458112
Feller, M. B. (2009). Retinal waves are likely to instruct the formation of eye-specific retinogeniculate projections. Neural Dev. 4:24. doi: 10.1186/1749-8104-4-24
Feller, M. B. M., Wellis, D. P. D., Stellwagen, D. D., Werblin, F. S. F., and Shatz, C. J. C. (1996). Requirement for cholinergic synaptic transmission in the propagation of spontaneous retinal waves. Science 272, 1182–1187. doi: 10.1126/science.272.5265.1182
Firl, A., Sack, G. S., Newman, Z. L., Tani, H., and Feller, M. B. (2013). Extrasynaptic glutamate and inhibitory neurotransmission modulate ganglion cell participation during glutamatergic retinal waves. J. Neurophysiol. 109, 1969–1978. doi: 10.1152/jn.00039.2013
Golding, B., Pouchelon, G., Bellone, C., Murthy, S., Di Nardo, A. A., Govindan, S., et al. (2014). Retinal input directs the recruitment of inhibitory interneurons into thalamic visual circuits. Neuron 81, 1057–1069. doi: 10.1016/j.neuron.2014.01.032
Hagihara, K. M., Murakami, T., Yoshida, T., Tagawa, Y., and Ohki, K. (2015). Neuronal activity is not required for the initial formation and maturation of visual selectivity. Nat. Neurosci. 18, 1780–1788. doi: 10.1038/nn.4155
Huberman, A. D., Feller, M. B., and Chapman, B. (2008). Mechanisms underlying development of visual maps and receptive fields. Annu. Rev. Neurosci. 31, 479–509. doi: 10.1146/annurev.neuro.31.060407.125533
Huberman, A. D., Wang, G.-Y., Liets, L. C., Collins, O. A., Chapman, B., and Chalupa, L. M. (2003). Eye-specific retinogeniculate segregation independent of normal neuronal activity. Science 300, 994–998. doi: 10.1126/science.1080694
Kerschensteiner, D. (2013). Spontaneous network activity and synaptic development. Neuroscientist 20, 272–290. doi: 10.1177/1073858413510044
Kerschensteiner, D., and Wong, R. O. L. (2008). A precisely timed asynchronous pattern of ON and OFF retinal ganglion cell activity during propagation of retinal waves. Neuron 58, 851–858. doi: 10.1016/j.neuron.2008.04.025
Kirkby, L. A., and Feller, M. B. (2013). Intrinsically photosensitive ganglion cells contribute to plasticity in retinal wave circuits. Proc. Natl. Acad. Sci. U S A 110, 12090–12095. doi: 10.1073/pnas.1222150110
Maccione, A., Hennig, M. H., Gandolfo, M., Muthmann, O., van Coppenhagen, J., Eglen, S. J., et al. (2014). Following the ontogeny of retinal waves: pan-retinal recordings of population dynamics in the neonatal mouse. J. Physiol. 592, 1545–1563. doi: 10.1113/jphysiol.2013.262840
McLaughlin, T., Torborg, C. L., Feller, M. B., and O’Leary, D. D. M. (2003). Retinotopic map refinement requires spontaneous retinal waves during a brief critical period of development. Neuron 40, 1147–1160. doi: 10.1016/s0896-6273(03)00790-6
Owens, M. T., Feldheim, D. A., Stryker, M. P., and Triplett, J. W. (2015). Stochastic interaction between neural activity and molecular cues in the formation of topographic maps. Neuron 87, 1261–1273. doi: 10.1016/j.neuron.2015.08.030
Pfeiffenberger, C., Yamada, J., and Feldheim, D. A. (2006). Ephrin-As and patterned retinal activity act together in the development of topographic maps in the primary visual system. J. Neurosci. 26, 12873–12884. doi: 10.1523/JNEUROSCI.3595-06.2006
Seabrook, T. A., El-Danaf, R. N., Krahe, T. E., Fox, M. A., and Guido, W. (2013). Retinal input regulates the timing of corticogeniculate innervation. J. Neurosci. 33, 10085–10097. doi: 10.1523/JNEUROSCI.5271-12.2013
Speer, C. M., Sun, C., Liets, L. C., Stafford, B. K., Chapman, B., and Cheng, H.-J. (2014). Eye-specific retinogeniculate segregation proceeds normally following disruption of patterned spontaneous retinal activity. Neural Dev. 9:25. doi: 10.1186/1749-8104-9-25
Stafford, B. K., Sher, A., Litke, A. M., and Feldheim, D. A. (2009). Spatial-temporal patterns of retinal waves underlying activity-dependent refinement of retinofugal projections. Neuron 64, 200–212. doi: 10.1016/j.neuron.2009.09.021
Stellwagen, D., and Shatz, C. J. (2002). An instructive role for retinal waves in the development of retinogeniculate connectivity. Neuron 33, 357–367. doi: 10.1016/s0896-6273(02)00577-9
Stellwagen, D., Shatz, C. J., and Feller, M. B. (1999). Dynamics of retinal waves are controlled by cyclic AMP. Neuron 24, 673–685. doi: 10.1016/s0896-6273(00)81121-6
Sun, C., Warland, D. K., Ballesteros, J. M., van der List, D., and Chalupa, L. M. (2008). Retinal waves in mice lacking the β2 subunit of the nicotinic acetylcholine receptor. Proc. Natl. Acad. Sci. U S A 105, 13638–13643. doi: 10.1073/pnas.0807178105
Wong, R. O. L. (1999). Retinal waves and visual system development. Annu. Rev. Neurosci. 22, 29–47. doi: 10.1146/annurev.neuro.22.1.29
Wong, W. T., Myhr, K. L., Miller, E. D., and Wong, R. O. (2000). Developmental changes in the neurotransmitter regulation of correlated spontaneous retinal activity. J. Neurosci. 20, 351–360.
Xu, H.-P., Burbridge, T. J., Chen, M.-G., Ge, X., Zhang, Y., Zhou, Z. J., et al. (2015). Spatial pattern of spontaneous retinal waves instructs retinotopic map refinement more than activity frequency. Dev. Neurobiol. 75, 621–640. doi: 10.1002/dneu.22288
Xu, H.-P., Furman, M., Mineur, Y. S., Chen, H., King, S. L., Zenisek, D., et al. (2011). An instructive role for patterned spontaneous retinal activity in mouse visual map development. Neuron 70, 1115–1127. doi: 10.1016/j.neuron.2011.04.028
Keywords: activity-dependent development, ferret, mouse, retinotopy, retinal waves, eye-specific segregation
Citation: Arroyo DA and Feller MB (2016) Spatiotemporal Features of Retinal Waves Instruct the Wiring of the Visual Circuitry. Front. Neural Circuits 10:54. doi: 10.3389/fncir.2016.00054
Received: 30 May 2016; Accepted: 12 July 2016;
Published: 26 July 2016.
Edited by:
Edward S. Ruthazer, McGill University, CanadaReviewed by:
Sarah L. Pallas, Georgia State University, USAJames Ackman, University of California, Santa Cruz, USA
Copyright © 2016 Arroyo and Feller. This is an open-access article distributed under the terms of the Creative Commons Attribution License (CC BY). The use, distribution and reproduction in other forums is permitted, provided the original author(s) or licensor are credited and that the original publication in this journal is cited, in accordance with accepted academic practice. No use, distribution or reproduction is permitted which does not comply with these terms.
*Correspondence: Marla B. Feller, mfeller@berkeley.edu