Modality-specific axonal regeneration: toward selective regenerative neural interfaces
- 1 Department of Bioengineering, University of Texas at Arlington, Arlington, TX, USA
- 2 University of Texas Southwestern Medical Center, Dallas, TX, USA
Regenerative peripheral nerve interfaces have been proposed as viable alternatives for the natural control of robotic prosthetic devices. However, sensory and motor axons at the neural interface are of mixed sub-modality types, which difficult the specific recording from motor axons and the eliciting of precise sensory modalities through selective stimulation. Here we evaluated the possibility of using type specific neurotrophins to preferentially entice the regeneration of defined axonal populations from transected peripheral nerves into separate compartments. Segregation of mixed sensory fibers from dorsal root ganglion neurons was evaluated in vitro by compartmentalized diffusion delivery of nerve growth factor (NGF) and neurotrophin-3 (NT-3), to preferentially entice the growth of TrkA+ nociceptive and TrkC+ proprioceptive subsets of sensory neurons, respectively. The average axon length in the NGF channel increased 2.5-fold compared to that in saline or NT-3, whereas the number of branches increased threefold in the NT-3 channels. These results were confirmed using a 3D “Y”-shaped in vitro assay showing that the arm containing NGF was able to entice a fivefold increase in axonal length of unbranched fibers. To address if such segregation can be enticed in vivo, a “Y”-shaped tubing was used to allow regeneration of the transected adult rat sciatic nerve into separate compartments filled with either NFG or NT-3. A significant increase in the number of CGRP+ pain fibers were attracted toward the sural nerve, while N-52+ large-diameter axons were observed in the tibial and NT-3 compartments. This study demonstrates the guided enrichment of sensory axons in specific regenerative chambers, and supports the notion that neurotrophic factors can be used to segregate sensory and perhaps motor axons in separate peripheral interfaces.
Introduction
Advanced robotic prosthetic limbs designed with multiple degrees of freedom bear great promise as substitutes for the human arm/hand in amputees (Lin and Huang, 1997; Miller et al., 2008; Velliste et al., 2008; Matrone et al., 2010; Otr et al., 2010). However, while thousands of touch-sensing receptors in the natural hand provide information about skin deformation and limb position, current hand prostheses lack such sensory feedback systems. Instead, users rely on unnatural vibrotactile and electrotactile sensors for surrogate feedback information, and operate the prosthetic limbs mostly under visual control (Phillips, 1988; Marasco et al., 2009; Micera and Navarro, 2009).
Indwelling multielectrode arrays (MEA) placed in the premotor cortex have been used successfully to record neural activity associated with motor intention in monkeys and humans, and to actuate robotic prosthesis (Hochberg et al., 2006; Simeral et al., 2011). These findings have fueled interest in the possibility of developing a closed-loop cortical interface able to convey tactile and positional information to amputees by direct electrical micro-stimulation of the sensory cortex (Fitzsimmons et al., 2007). However, since the topographic mapping in the somatosensory cortex is quite variable, and is currently unclear which cortical neurons provide information during sensory sub-modality discrimination, this strategy may be limiting in conveying distinct sensations such as pain, touch, thermal, and limb/digit stretch. Furthermore, direct cortical stimulation lacks the benefit of signal integration and modulation from neural networks in the spinal cord and thalamus, known to provide critical context-dependent regulation of information and sensory discrimination (Brown et al., 2004; Lee et al., 2008; Rosenzweig et al., 2010).
Alternatively, tactile and positional information detected from specialized neurons innervating the skin, muscle, and tendons can be interfaced at the dorsal root ganglia (DRG; Weber et al., 2007; Gaunt et al., 2009) or in the transected peripheral nerve (Dhillon et al., 2004; Brill et al., 2009), and used for eliciting sensation in amputees (Dhillon and Horch, 2005). However, modality-specific neurons such as nociceptive and proprioceptive have intermixed perikarya in the DRG, and assorted axons in most peripheral nerves (Castro et al., 2008). Thus, selective electrical stimulation is particularly challenging, and is further complicated by the fact that large myelinated axons (i.e., proprioceptive) are depolarized with smaller currents, while smaller diameter neurons (i.e., nociceptive) require larger stimuli. Thus, when stimulating the small caliber fibers in a nerve with assorted axon types, likely the large-size axons will be non-specifically recruited, eliciting either mixed sensations and/or involuntary motor movements (Grill et al., 2009).
We recently reported the use of an 18-electrode regenerative multielectrode interface (REMI) to record multiunit activity in both acute and chronically damaged peripheral nerves (Garde et al., 2009). Regenerative interfaces such as the REMI are unique since axonal growth can potentially be guided specifically to compartmentalized electrodes using neuron-specific growth factors. Neurotrophic factors such as nerve growth factor (NGF), brain-derived neurotrophic factor (BDNF) and neurotrophin-3 (NT-3), are known to promote cell survival, nerve regeneration and functional recovery not only in the injured peripheral nerve and spinal cord, but in neurodegenerative conditions such as Parkinson and Alzheimer diseases (Fischer et al., 1991; Nagahara et al., 2009; Brock et al., 2010; Rangasamy et al., 2010; Jin et al., 2011). In the peripheral nerve, BDNF and glial cell-derived neurotrophic factors (GDNF), have been shown to promote axonal regeneration of motor neurons (Boyd and Gordon, 2003b; Geremia et al., 2010), whereas NGF and NT-3 are know to stimulate selectively axonal growth of pain, and proprioceptive axons, respectively (Misko et al., 1987; Oakley et al., 1997). Such selectivity relies on the specific binding of NGF to TrkA receptors in nociceptive, and of NT-3 to TrkC receptors on proprioceptive, DRG neurons. Furthermore, the induced expression of NGF or NT-3 effectively guides the regeneration of nociceptive and proprioceptive sensory axons into proper targets in the dorsal or ventral spinal cord (Blits et al., 2000; Romero et al., 2000, 2001), and into skin and motor branches in the periphery (Hu et al., 2010).
Here we hypothesized that mixed axons from an amputated nerve can be segregated into separate regenerative compartments through neuron-type specific neurotrophins. To test this possibility, we investigated the feasibility of using NGF and NT-3 to selectively attract TrkA nociceptive axons and TrkC proprioceptive axons both in vitro and in vivo. Our results are consistent with the notion that specific molecular cues can be used to guide the axonal growth of a mixed axonal population into specific compartments.
Materials and Methods
Dorsal Root Ganglion Explants Culture
Dorsal root ganglia were obtained from neonate (P0–P3) rats. The animals were anesthetized by hypothermia and sacrificed. The spinal cord was harvested into Hank’s buffered salt solution (Gibco, Carlsbad, CA, USA), and cleaned from dorsal and ventral roots using tungsten needles. Individual DRGs were placed in the main well of the choice assay and fixed in place using 50 μl of growth factor reduced ECM (Matrigel; BD Biosciences, San Jose, CA, USA). After ECM polymerization for 5 min at 37°C, the explants were cultured in neurobasal medium supplemented with L-glutamine, B-27, and 1% penicillin/streptomycin (Gibco).
In vitro 2D Y-Shape Assay
A “Y” shaped polydimethylsiloxane (PDMS) template was fabricated containing a circular well for DRG placement in the common arm, and two arms terminating in wells where pieces of Gelfoam (MWI, Meridian, ID, USA) containing neurotrophins were placed. The PDMS was polymerized onto a negative template (1 volume PDMS: 10 volume curing agent mixed at 50°C for 2 h), cleaned, sterilized, and placed on tissue culture chamber slides. Immersing in 70% ethanol and exposing to UV light sterilized the PDMS templates. The DRG explants were then placed in the well with a thin layer of growth factor reduced Matrigel® polymerized on top at 37°C for 30 min. After 2 days in culture, pieces of gelfoam (3 mm × 3 mm) pre-soaked in saline (n = 10; negative control), 7S NGF (n = 10; 100 ng/ml, Sigma, St. Louis, MO, USA), or NT-3 (n = 12; 5 ng/ml, Sigma, St. Louis, MO, USA) were placed in the “target” wells. A piece of glass coverslip was placed over the entire PDMS template with openings at the DRG and gelfoam ends. The coverslip served as a ceiling to the microchannels, prevented floating of the gelfoam and delayed neurotrophin dilution into the media. To demonstrate that the choice assay provides independent gradients of neurotrophins from the separate “target” compartments to the DRG, we placed a piece of gelfoam with Cy2 (897 Da-green) and Cy3 (765 Da-red) dyes in the two separate compartments and determine the fluorescence diffusion into the microfluidic channels at 4, 6, 8, and 12 h. A Zeiss Pascal laser confocal microscope equipped with an environmental chamber was used to evaluate the optical densitometry of the Cy2 and Cy3 fluorescence over time, maintaining the cultures at 37°C and 70% humidity. The diffusion rate was confirmed in separate experiments using Gelfoam loaded with bovine serum albumin (BSA) conjugated alexafluor-594 (66 kDa), as its molecular weight approximates that of the neurotrophins (NGF = 135 kDa and NT-3 = 27 kDa) used in this study.
In vitro 3D Y-Shape Assay
A casting device was designed for 3D cell growth in collagen-filled agarose microchannels similar to that recently described (Dawood et al., 2011). Briefly, a “Y” shaped fiber consisting of a base (OD 0.5, 10 mm long) and two arms (OD 0.25, 11 mm long) were inserted through perforations into separate loading wells (Figure 4A). The hydrogel 1.5% agarose was polymerized over the fibers, collagen IV (Chemicon International, Temecula, CA, USA) was placed in the loading well. Removal of the common fiber not only casted a “Y”-shaped microchannel in the agarose gel, but due to the negative pressure created filled the lumen with collagen. After polymerization of the collagen, we added either NGF (n = 4; 100 ng/ml; Sigma, St. Louis, MO, USA) or NT-3 (n = 4; 500 ng/ml; Sigma, St. Louis, MO, USA) into separate wells to provide separate guidance cues in the arms of the “Y” assay. A DRG explanted from a post-natal day 2 mouse pups was then inserted into the base arm and allowed to grow for 5 days at 37°C and 5% CO2 in a humidified incubator.
In vivo Segregation
A “Y”-shaped tubular conduit was designed consisting of a common arm (OD 0.5, 3 mm long) and two arms (OD 0.25, 7 mm long) using polyurethane tubing (Micro-Renathane®). The animals were anesthetized (Ketamine/medetomidine, 87 mg/kg i.p.) before being subjected to the sciatic nerve transection injury. The sciatic nerve in 32 rats (4 groups; n = 8) was transected and repaired by either a straight tube (normal mixed axonal control) or Y-tube implants filled with collagen in the common arm. The left and right arms were then filled with either collagen, or collagen/NGF or collagen/NT-3. Four experimental groups were evaluated: (1) Simple tube distally attached to the unbranched sciatic nerve (mixed control), (2) “Y” tube distally attached to the tibial and sural nerves (positive control), (3) “Y” tube filled with collagen (negative control), (4) “Y” tube filled with either NGF and/or NT-3; in groups 3 and 4 the distal nerve was not sutured to the tubing. Following a 2-month post-operative survival period, all animals were sacrificed and implants were recovered. The regenerated nerves were sectioned and immunostained to identify large myelinated axons (N-52 clone of neurofilament 200, mouse anti-N-52, 1:3000; Sigma, St. Louis, MO, USA) and pain axons that express calcitonin gene-related peptide (CGRP+). The number of CGRP+ and N-52+ was then quantified from traced digital confocal microscopy photographs in each of the regenerated nerves. This study was performed in accordance to the Institutional Animal Care and Use Committee at UT Southwestern Medical Center.
Immunocytochemical Analysis
The explant cultures were fixed 2 days after the neurotropic treatment using 4% paraformaldehyde (PFA). DRG cultures were rinsed with PBS, permeabilized in 0.25% triton-X for 30 min at room temperature, and blocked by 1% normal donkey serum for another 30 min at room temperature. Regenerated axons from the DRG explants were labeled with β-tubulin (mouse anti- β-tub, 1:500; Sigma, St. Louis, MO, USA) and calcitonin gene-related peptide (CGRP; rabbit anti-CGRP, 1:2000; Chemicon, Temecula, CA, USA). Visualization of axonal growth in the 3D “Y” assay was achieved by labeling the axons with phalloidin green (Molecular Probes, Carlsbad, CA, USA), stack imaged using confocal microscopy and collapsing the 3D image to obtained a 2D rendition of the 3D growth.
Animals were perfused with PBS-1% heparin, followed by 4% PFA. The regenerated nerve was harvested and processed for immunohistochemistry. Double labeling studies were done on longitudinally cut cryosections using the monoclonal N-52 clone of neurofilament 200 (N-52) and CGRP. The tissue was then visualized using Cy2 Goat anti-Rabbit 1:250, Cy3, and Cy2 Goat anti-Mouse 1:500; Cy3 Goat anti-Rat 1:400 (Jackson labs, West Grove, PA, USA). Sections mounted using Vectashield containing the nuclear label DAPI (Molecular Probes, Carlsbad, CA, USA).
Image Analysis and Quantification
For in vitro segregation studies, digital images of 20× magnifications were obtained using a confocal microscope (Zeiss LSM 510 Meta). The length of axons was quantified in each channel to calculate mean axonal length. Only neurite processes longer that 20 μm were counted. For 3D in vitro experiments, the length of four to six axonal fibers per animal was measured to determine the average in each treatment group. For in vivo segregation studies, three stacked images of the A, B, and C arms were captured using confocal microscopy for each subject. Double-level standardized optical density threshold was applied to subtract the background and the saturated intensity values. A circle (fixed area 0.03 mm2) was placed over three randomly selected areas for fiber growth quantification. The area (in μm2) of positively stained N-52+ axons and CGRP+ axons was measured in all three arms of the Y-shaped nerve regenerate.
Statistical Analysis
The data is reported as the mean and the SE of the mean (SEM). An unpaired Student’s t-test was used to determine statistical differences. In multiple group comparisons, one-way ANOVA was used followed by Neuman–Keuls multiple comparison post hoc evaluation (Prism 4, GraphPad). p-Values ≤ 0.05 were considered significant.
Results
An in vitro “Y” assay was used to determine if separate delivery of NGF and NT-3 can differentially entice axonal regeneration of specific neurons from the mixed population of DRG sensory neurons. The assay consisted in a PDMS mold where early post-natal DRGs were placed at a 1-mm distance from the bifurcation of 5 mm “Y” arms ending in separate compartments. Gelfoam soaked with saline (control), NGF, or NT-3 was presented 48 h after DRG plating and the axonal growth into the separate compartments evaluated (Figure 1A). To verify that the assay provided separated molecular cues we measured the diffusion rate of fluorescently labeled Cy2 (green) or Cy3 (red) antibodies in each compartment. We confirmed that the molecules diffused separately in each arm of the “Y” assay, providing a distinctive and measurable gradient for up to 8–12 h (Figure 1B).
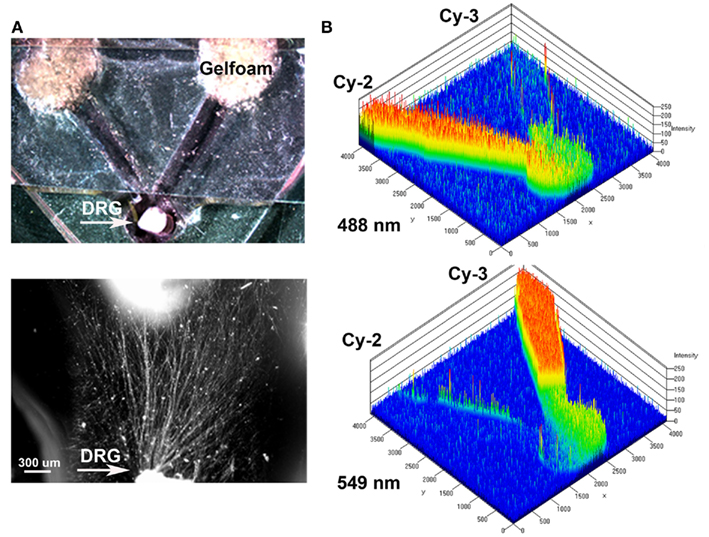
Figure 1. Y-shaped in vitro assay for axonal segregation. (A) Gelfoam-diffusion delivery of neurotrophins into the distal arms was used to differentially entice axonal outgrowth from neonatal DRGs. Bottom: higher magnification shows axonal growth from the DRG (arrow) in the choice area. (B) Diffusion of green (Cy3) and red (Cy3) labeled antibodies, were imaged over time and quantified to demonstrate independent gradient formation.
In absence of guidance cues, DRGs showed axonal growth of mixed axon morphologies (i.e., length or number of branches) into the arms of the Y assay (not shown). In contrast, when NGF was deliver into both arms we observed that axons were long and lacked branches (Figure 2Aa). Conversely, when NT-3 was delivered into the microchannels the axons were shorter and with numerous branch collaterals (Figure 2Ab). Such long unbranched axons, and short-branched axons are morphologically characteristic of the nociceptive and proprioceptive sensory axons in vitro (Romero et al., 2007). We next evaluated whether NGF and NT-3 if presented simultaneously in two separate microchannels would differentially entice the growth of distinct population of neurons. In that assay, axons growing from single DRGs toward the NGF-containing compartment were long and relatively unbranched, while those attracted toward NT-3 were branched and shorter in length. Double immunolabeling for β-tubulin (axonal marker) and CGRP (specific marker for nociceptive sensory axons), confirmed that CGRP+ pain/nociceptive fibers are predominantly attracted toward NGF, while those growing toward NT-3 were CGRP-/N-52+ axons (Figure 2B). Quantitative analysis (Figure 3) showed that axon length and branch number averaged 139.5 ± 17.85 μm, and 4.85 ± 0.82 respectively, in the saline treated groups. In contrast, the average axon length in the NGF channel (352.1 ± 40.24 μm) increase 2.5-fold (p ≤ 0.001) compared to that in saline or NT-3 (115.8 ± 10.30 μm), whereas the number of branches increased threefold in the NT-3 channels (15.75 ± 2.25; n = 12; p ≤ 0.01), compared to saline or NT-3-treated (7 ± 0.95) groups. These results supported the possibility that different sensory axon subtypes in the DRG can be differentially attracted to separate compartments in culture using neuron-specific guidance signals.
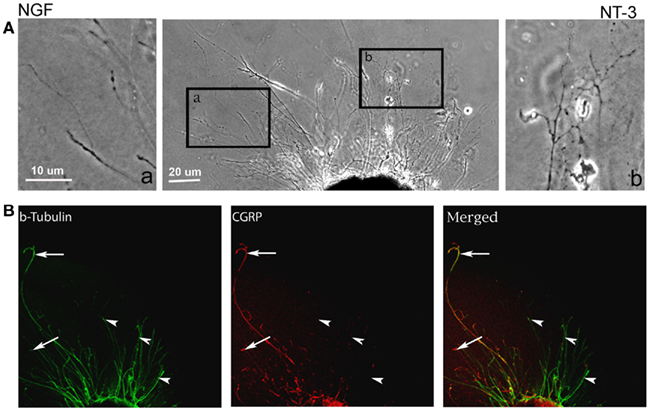
Figure 2. Differential axonal morphology of axons growing toward NGF or NT-3. (A) Mixed axon morphologies were observed in control DRGs. Magnified images of the area in boxes (in right and left) detail the morphological differences in the NGF (n = 10) and NT-3 (n = 12) treated groups. Axons growing toward NGF showed characteristic long and unbranched morphology. In contrast, those growing toward NT-3 were short and highly branched axons. (B) Visualization of β-tubulin (green) and CGRP (red) demonstrated that axons growing toward NGF are CGRP-positive (i.e., nociceptive, arrows), while those growing toward NT-3 are CGRP negative.
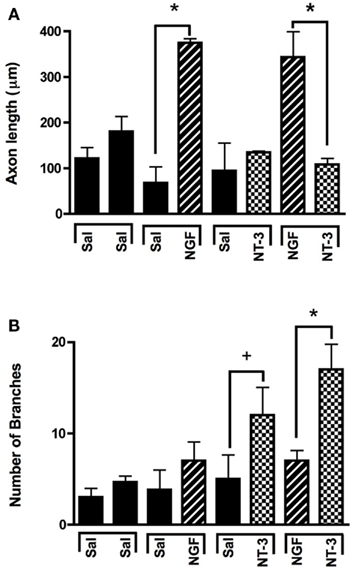
Figure 3. Selective neuron growth of DRG sensory axons by compartmentalized neurotrophin delivery. (A) NGF selectively induced the growth of longer axons compared to control and NT-3-treated groups. (B) NT-3 increased significantly the number of branches per axon compared to control and NGF. *p < 0.001, +p < 0.01. (n = 10–12).
We then evaluated the feasibility of delivering neurotrophins into a luminar collagen matrix of a 3D Y-shaped transparent hydrogel assay. Using a custom device (Figures 4A,C), we confirmed the formation of gradients in separate arms of the 3D-assay by adding Trypan blue dye into a single loading well (Figures 4B,B′). Quantification of the absorbance from 1 mm segments taken at top middle and bottom of the “Y” arm after 7 days revealed a 2.14 dilution at the bifurcation point (Figure 4D). When NGF and NT-3 were delivered into separate arms of the “Y” assay and a DRG placed at the bottom of the base channel, we observed the long unbranched axons in the arm with luminal NGF and short-branched axons in the NT-3 arm (Figures 4E,F). In this assay both neurotrophins elicited longer axonal growth compared to no-treatment controls, but that containing NGF was able to entice a fivefold increase in axonal length of unbranched fibers (Figure 4G).
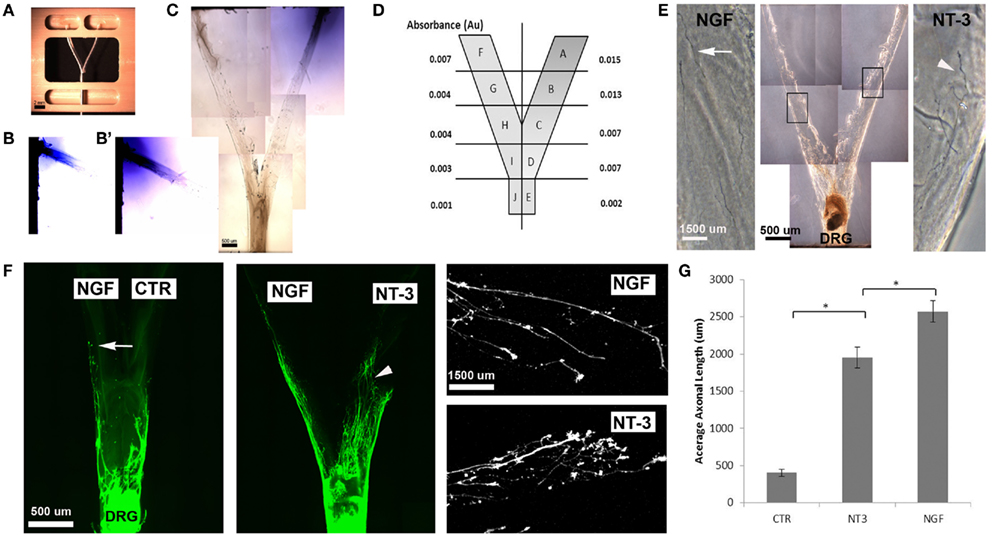
Figure 4. Three-dimensional Y-shaped microchannels. (A) Casting device with dual cell-seeding wells and containing a Y-shaped fiber. Subsequent to the addition of agarose in the central well and collagen in both the cell-seeding wells, the brush is extracted thus, forming a collagen-filled Y-shaped channel. (B) Diffusion of blue dye overtime in collagen at day 3 and day 7 (B’). (C) Photograph of the Y-channel homogenously filled with collagen. (D) Schematic of the Y-shaped assay with quantified absorbance in each segment measured at 7 days of continuous dye delivery into the right well. (E) Axonal growth from DRG into channels containing either NGF (n = 4) or NT-3 (n = 4) confirmed differences in axonal morphology in the separate compartments. (F) Compared to untreated channels, those with NGF showed longer and unbranched axons (arrows), while those in the NT-3 compartment were relatively shorter and highly arborized (arrowheads). (G) Quantification of mean axonal length confirmed the growth promoting effect of the neurotrophins. *p < 0.001.
Compartmentalized NT-3 In vivo Enriches N-52-Positive Fibers
To test if mixed axons in the transected peripheral nerve could be segregated into modality-specific compartments in vivo, we compared the segregation effect of natural distal targets such as the sural (primarily sensory) and tibial (mixed sensorimotor) nerves; distally connected to each arm of a Y-shaped tube, to that enticed by NGF and NT-3. The sciatic nerve in 32 rats was completely transected and repaired with either straight or Y-tubes. The two arms of the Y-tubes were filled with collagen and distally sutured to the tibial or sural nerves, or filled with NGF- or NT-3-containing collagen. Following a 2-month survival the regenerated tissue was longitudinally sectioned and stained for specific markers. All implanted groups demonstrated axonal outgrowth in the common arm of the implants (Figure 5). Compared to simple tubularization nerve gap repair that mediate the regeneration of a single nerve cable (Figure 5A), Y-tubes containing collagen connected distally to sural and tibial nerves showed robust Y-shaped nerve regeneration (Figure 5B). Growth in those with collagen-only was minimal in absence of distal targets (Figure 5C), but was qualitatively enhanced if NGF and NT-3 were added in the Y-arms (Figure 5D).
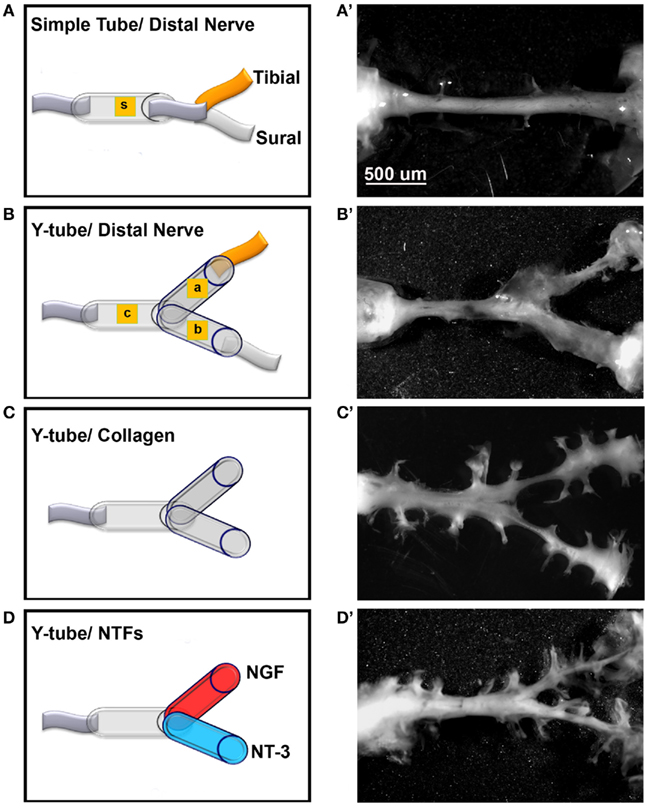
Figure 5. Guided peripheral nerve regeneration. (A–D) Schematic representation of the experimental groups (n = 8) tested in vivo. s = single tube, c = common arm, a and b = left and right arms of the Y-shaped tube. (A’–D’) Photographs of regenerated nerves 60 days post-tubularization. A single nerve cable was observed in nerves repaired with straight tubes (A’), A Y-shaped nerve regenerate formed in the other groups. The regenerated tissue was thicker with the sural and tibial nerves attached distally (B’), dramatically reduced in absence of distal treatment (C’), and increased with neurotrophin delivery (D’).
To determine the specific modality of the neurons that grew into the different “Y” chambers, we tried to retrograde label those axons using Flourogold as recently reported (Tansey et al., 2011). However, the limited amount of tissue distal to the Y-arms caused cross-contamination during labeling. Alternatively, we resourced to markers specific for nociceptive fibers (CGRP+) and for large-diameter axons (N-52+). In the DRG, sciatic nerve (Figure A1 in Appendix) and spinal cord (not shown), CGRP labeled exclusively the TrkA pain fibers while N-52 stained large TrkC neurons and large-diameter axons. The staining did not overlap, thus labeling these two distinctive sub-populations of neurons in the experimental groups confirmed that the CGRP+ fibers were qualitatively more abundant in the arms of the Y-tube sutured distal to the sural nerve (Figure 6A), and N-52+ axons appeared to be more dense in the arm attached to the tibial nerve (Figure 6B). In those with NGF/NT-3, no differences were apparent with CGRP labeling, but seemed more abundant when labeled with N-52 in the arm with NT-3 (Figure 6C).
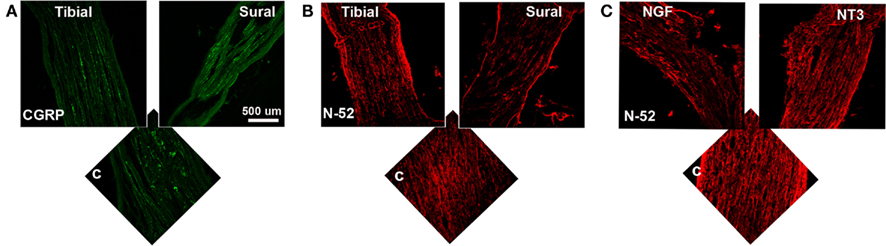
Figure 6. (A) In the “Y” shaped nerve regenerate, both axon types are present in the common arm (c), whereas those attached to the tibial nerve showed apparently less CGRP+ axons compared to those growing into the sural nerve compartment. (B) Conversely, N-52+ axons appear denser in the tibial compared to the sural compartments. (C) In the NT-3 and NGF groups, N-52+ axons were more prevalent in the NT-3 arm.
Quantitative analysis using optical densitometry in single tube regenerated nerve, or nerve regenerated through the common (c) arm of the Y-tube, showed no significant differences between the treatment groups (Figure 7). In sharp contrast, the density of CGRP+ axons (Figure 7A) was significantly increased in the compartment attached to the sural nerve (p < 0.01; 7528 ± 604.7 OD units), but not in that supplemented with NGF (1884 ± 504.8 OD units). Conversely, N-52+ immunoreactivity was significantly increased (p < 0.01), both the tibial (8718 ± 769.2 OD units) and NT-3 (9120 ± 1080 OD units) compartments (Figure 7B). We also noted significant increases (p < 0.05) in CGRP in the tibial (4894 ± 739.4 OD units) and of N-52 in the sural (9689 ± 676.3 OD units), compartments.
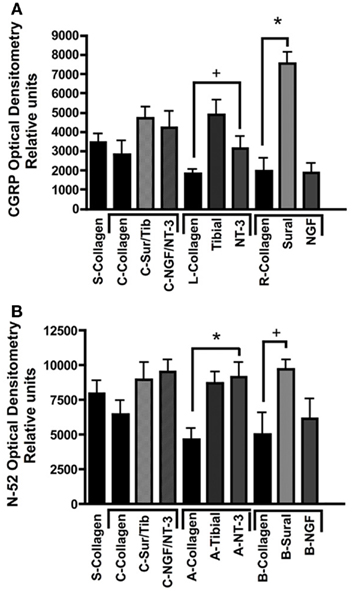
Figure 7. Optical densitometry of CGRP and N-52 axons. (A) Pain fibers (CGRP+) axons are grown in a significantly larger numbers in arms filled with NGF and tibial nerve compared to collagen or NT-3. (B) Large-diameter axons were attracted toward the tibial and NT-3 channels, but also to the sural nerve compared to collagen controls. *p < 0.01, +p < 0.05.
Together, the data indicate that specific growth factor combinations can be used to guide the axonal growth of a mixed neuron population in an amputated nerve and coerce specific types of regenerative fibers to grow into separate compartments.
Discussion
The transected peripheral nerve provides an optimal site for the neural interfacing as movement commands can be recorded from motor axons, and electrical stimulation of sensory fibers can convey natural sensation to the user of advanced robotic prosthetic devices. Stimulation of sensory axons conveys sensory feedback with natural integration and modulation at the spinal cord, brain stem, and thalamus prior to reaching the sensory cortex. Furthermore, neuron-specific stimulation will be able to convey precise sub-modality sensory information such as pain, temperature, and limb stretching.
Peripheral nerve interfacing has been accomplished either through extraneural (i.e., cuff electrodes; Leventhal and Durand, 2004 #2143), or intraneural electrodes. Indwelling interfaces like the longitudinally implanted intrafascicular electrodes (LIFEs; Lefurge et al., 1991) have been used successfully to record motor signals from the peripheral nerve, and through stimulation, used to convey sensation in long-term amputee human volunteers (Dhillon et al., 2004; Benvenuto et al., 2010). However, indwelling electrodes like LIFE provide limited long-term efficacy due to the progressive reduction in the number of active sites over time, poor bio–abio interface, tissue damage, and loss of recording activity due to electrode insulation as a result of tissue scar formation (Biran et al., 2005, 2007; Williams et al., 2007; Leung et al., 2008).
Regenerative sieve electrodes were proposed more than three decades ago as a viable alternative to interface motor and sensory nerve axons (Mannard et al., 1974; Edell et al., 1982; Dario et al., 1998). Sieve electrodes have been shown to obtain neural recordings after long-term (i.e., 2–6 months) implantation (Klinge et al., 2001; Lago et al., 2007; Panetsos et al., 2008), and we recently obtained long-lasting single and multiunit recordings using a non-obstructive REMI placed between the transected ends of an end-to-end repaired nerve, and after interfacing the nerve 5–6 months after injury despite absent connections to their normal target muscles (Garde et al., 2009). Here we tested the possibility to use neurotrophins to guide the regenerative process of the transected peripheral nerve and segregate the growing axons into modality-specific compartments.
Previous reports have shown that incorporation of growth factors into neural interface electrodes can increase the sensitivity of the neural interfaces by attracting axons to the recording sites. Indeed, several growth factors and adhesion molecules have been incorporated to conductive substrates. NGF has been attached to polypyrrole (Gomez and Schmidt, 2007), or combined with laminin and applied to polymer polyethylene dioxythiophene (Green et al., 2010) and brain-derived neurotrophic factor (BDNF) and NGF have been entrapped in hydrogels polymerized over the electrodes or in nanopore membranes (Lopez et al., 2006; Winter et al., 2007; Jun et al., 2008).
This study shows that in addition to the general chemoattractive nature of the neurotrophins, neuron-specific molecular guidance cues can be used to separate the regeneration of specific types of neurons. DRG neurons have been broadly classified based on cell body size, axonal diameter, conduction velocity, and the expression of either NGF, BDNF, or NT-3 Trk receptors (Harper and Lawson, 1985; Misko et al., 1987; Oakley et al., 1997), and further differentiated based on the expression of N-52 and CGRP, which are preferentially expressed in large and small-diameter nerve fibers, respectively (Goldstein et al., 1991; Zhang et al., 1995; Ho and O’Leary, 2011). We and others have shown that exogenous expression of NGF can be used successfully to entice and direct axonal regeneration of CRGP-positive pain axons in the brain (Curinga and Smith, 2008), spinal cord (Romero et al., 2000, 2001), and peripheral nerves (Hu et al., 2010). This study shows that compartmentalized diffusion of NGF and NT-3 can differentially entice and direct the regeneration CGRP+ nociceptive and N-52+ large-diameter fibers, such as proprioceptive and mechanoreceptive axons, into separate chambers. This notion is further supported by the observed morphological dimorphism observed in DRG axons growing into the NGF and NT-3 compartments, in which, similarly to that previously reported in vitro, NGF-dependent nociceptive neurons grow mostly long and unbranched axons, while NT-3 dependent proprioceptive neurons show increased axonal branching (Romero et al., 2007). Furthermore, Y-nerve regeneration assays showed that NT-3 can mimic the specific enticement of N-52+ axons observed in chambers in which the tibial mixed sensory-motor nerve was sutured distally. Conversely, the sural sensory nerve was able to attract a larger population of CGRP-positive axons toward that compartment. However, we were unable to replicate such effect when NGF release was compartmentalized. Since we used a larger concentration of NGF (100 ng/ml) compared to that of NT-3 (5 ng/ml), it is possible that a lower NGF concentration is needed for an optimal axonal enticement, and future studies will be required to optimize the concentration and nature of the signaling cues needed to achieve maximal and more selective segregation.
This study demonstrates the guided enrichment of nociceptive axons in this particular regenerative chamber, and supports the notion that neurotrophic factors can be used directly as a means to enrich sensory and perhaps motor axons into an electrode interface (see model in Figure 8). However, since no electrical recording was done in this study, additional research is needed to demonstrate the selective recording/stimulation of growth factor-enticed REMI. Future experiments are also needed to test weather growth factors such as glial-derived neurotrophin factor and BNDF, known to stimulate the regeneration of motor neurons (Boyd and Gordon, 2003a; Jubran and Widenfalk, 2003), can be use to segregate motor from sensory fibers. Achieving a greater concentration of axons from a particular neural subtype is expected to provide a more sophisticated and selective peripheral neuro-interface. The selective regeneration of subtypes of neurons into specific target chambers would be better suited to achieve selective stimulation of a neuron subtype, compared to the penetration electrodes that are in contact with mixed axons from different neuron types, as this would minimize the possibility of unintentional neural activation. Ultimately, such an arrangement would reduce the burden of data extraction from mixed signals from electrodes embedded in a mixed neuron population, and achieve selective recording and stimulation of the regenerative peripheral neurointerfaces, which in turn can be valuable in order to achieve more precise control of the robotic prosthetic hand.
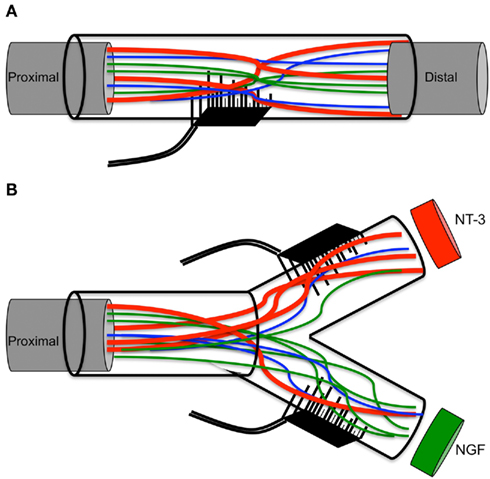
Figure 8. Schematic of multielectrode compartments. (A) Mixed nature of regenerative nerve in the absence of any molecular cues. (B) Specific growth factors attract a subtype of neurons to the modality-specific compartment.
Conflict of Interest Statement
The authors declare that the research was conducted in the absence of any commercial or financial relationships that could be construed as a potential conflict of interest.
Acknowledgments
This work was supported by the Defense Advanced Research Projects Agency and the Crowely-Carter Foundation (Mario I. Romero-Ortega). We thank Russ Daniel for administrative assistance and Myresa Hurst for expert technical support.
References
Benvenuto, A., Raspopovic, S., Hoffmann, K. P., Carpaneto, J., Cavallo, G., Di Pino, G., Guglielmelli, E., Rossini, L., Rossini, P. M., Tombini, M., and Micera, S. (2010). Intrafascicular thin-film multichannel electrodes for sensory feedback: evidences on a human amputee. Conf. Proc. IEEE Eng. Med. Biol. Soc. 2010, 1800–1803.
Biran, R., Martin, D. C., and Tresco, P. A. (2005). Neuronal cell loss accompanies the brain tissue response to chronically implanted silicon microelectrode arrays. Exp. Neurol. 195, 115–126.
Biran, R., Martin, D. C., and Tresco, P. A. (2007). The brain tissue response to implanted silicon microelectrode arrays is increased when the device is tethered to the skull. J. Biomed. Mater. Res. A 82, 169–178.
Blits, B., Dijkhuizen, P. A., Boer, G. J., and Verhaagen, J. (2000). Intercostal nerve implants transduced with an adenoviral vector encoding neurotrophin-3 promote regrowth of injured rat corticospinal tract fibers and improve hindlimb function. Exp. Neurol. 164, 25–37.
Boyd, J. G., and Gordon, T. (2003a). Glial cell line-derived neurotrophic factor and brain-derived neurotrophic factor sustain the axonal regeneration of chronically axotomized motoneurons in vivo. Exp. Neurol. 183, 610–619.
Boyd, J. G., and Gordon, T. (2003b). Neurotrophic factors and their receptors in axonal regeneration and functional recovery after peripheral nerve injury. Mol. Neurobiol. 27, 277–324.
Brill, N., Polasek, K., Oby, E., Ethier, C., Miller, L., and Tyler, D. (2009). Nerve cuff stimulation and the effect of fascicular organization for hand grasp in nonhuman primates. Conf. Proc. IEEE Eng. Med. Biol. Soc. 2009, 1557–1560.
Brock, J. H., Rosenzweig, E. S., Blesch, A., Moseanko, R., Havton, L. A., Edgerton, V. R., and Tuszynski, M. H. (2010). Local and remote growth factor effects after primate spinal cord injury. J. Neurosci. 30, 9728–9737.
Brown, P. B., Koerber, H. R., and Millecchia, R. (2004). From innervation density to tactile acuity: 1. Spatial representation. Brain Res. 1011, 14–32.
Castro, J., Negredo, P., and Avendano, C. (2008). Fiber composition of the rat sciatic nerve and its modification during regeneration through a sieve electrode. Brain Res. 1190, 65–77.
Curinga, G., and Smith, G. M. (2008). Molecular/genetic manipulation of extrinsic axon guidance factors for CNS repair and regeneration. Exp. Neurol. 209, 333–342.
Dario, P., Garzella, P., Toro, M., Micera, S., Alavi, M., Meyer, U., Valderrama, E., Sebatiani, L., Ghelarducci, B., Mazzoni, C., and Pastacaldi, P. (1998). Neural interfaces for regenerated nerve stimulation and recording. IEEE Trans. Rehabil. Eng. 6, 353–363.
Dawood, A., Lotfi, P., Dash, S. N., Kona, S., Nguyen, K. T., and Romero-Ortega, M. I. (2011). VEGF release in multiluminal hydrogels directs angiogenesis from adult vasculature in vitro. Cardiovasc. Eng. Technol. 2, 173–185.
Dhillon, G. S., and Horch, K. W. (2005). Direct neural sensory feedback and control of a prosthetic arm. IEEE Trans. Neural Syst. Rehabil. Eng. 13, 468–472.
Dhillon, G. S., Lawrence, S. M., Hutchinson, D. T., and Horch, K. W. (2004). Residual function in peripheral nerve stumps of amputees: implications for neural control of artificial limbs. J. Hand Surg. Am. 29, 605–615; discussion 16–18.
Edell, D. J., Churchill, J. N., and Gourley, M. (1982). Biocompatibility of a silicon based peripheral nerve electrode. Biomater. Med. Devices Artif. Organs 10, 103–122.
Fischer, W., Bjorklund, A., Chen, K., and Gage, F. H. (1991). NGF improves spatial memory in aged rodent as a function of age. J. Neurosci. 11, 1889–1906.
Fitzsimmons, N. A., Drake, W., Hanson, T. L., Lebedev, M. A., and Nicolelis, M. A. (2007). Primate reaching cued by multichannel spatiotemporal cortical microstimulation. J. Neurosci. 27, 5593–5602.
Garde, K., Keefer, E., Botterman, B., Galvan, P., and Romero, M. I. (2009). Early interfaced neural activity from chronic amputated nerves. Front. Neuroeng. 2:5.
Gaunt, R. A., Hokanson, J. A., and Weber, D. J. (2009). Microstimulation of primary afferent neurons in the L7 dorsal root ganglia using multielectrode arrays in anesthetized cats: thresholds and recruitment properties. J. Neural Eng. 6, 55009.
Geremia, N. M., Pettersson, L. M., Hasmatali, J. C., Hryciw, T., Danielsen, N., Schreyer, D. J., and Verge, V. M. (2010). Endogenous BDNF regulates induction of intrinsic neuronal growth programs in injured sensory neurons. Exp. Neurol. 223, 128–142.
Goldstein, M. E., House, S. B., and Gainer, H. (1991). NF-L and peripherin immunoreactivities define distinct classes of rat sensory ganglion cells. J. Neurosci. Res. 30, 92–104.
Gomez, N., and Schmidt, C. E. (2007). Nerve growth factor-immobilized polypyrrole: bioactive electrically conducting polymer for enhanced neurite extension. J. Biomed. Mater. Res. 81, 135–149.
Green, R. A., Lovell, N. H., and Poole-Warren, L. A. (2010). Impact of co-incorporating laminin peptide dopants and neurotrophic growth factors on conducting polymer properties. Acta Biomater. 6, 63–71.
Grill, W. M., Norman, S. E., and Bellamkonda, R. V. (2009). Implanted neural interfaces: biochallenges and engineered solutions. Annu. Rev. Biomed. Eng. 11, 1–24.
Harper, A. A., and Lawson, S. N. (1985). Conduction velocity is related to morphological cell type in rat dorsal root ganglion neurones. J. Physiol. (Lond.) 359, 31–46.
Ho, C., and O’Leary, M. E. (2011). Single-cell analysis of sodium channel expression in dorsal root ganglion neurons. Mol. Cell. Neurosci. 46, 159–166.
Hochberg, L. R., Serruya, M. D., Friehs, G. M., Mukand, J. A., Saleh, M., Caplan, A. H., Branner, A., Chen, D., Penn, R. D., and Donoghue, J. P. (2006). Neuronal ensemble control of prosthetic devices by a human with tetraplegia. Nature 442, 164–171.
Hu, X., Cai, J., Yang, J., and Smith, G. M. (2010). Sensory axon targeting is increased by NGF gene therapy within the lesioned adult femoral nerve. Exp. Neurol. 223, 153–165.
Jin, Y., Zhang, C., Ziemba, K. S., Goldsteain, G. A., Sullivan, P. G., and Smith, G. M. (2011). Directing dopaminergic fiber growth aling a performed molecular pathway from embryonic ventral mesencephalon transplants in the rat brain. J. Neurosci. Res. 89, 619–627.
Jubran, M., and Widenfalk, J. (2003). Repair of peripheral nerve transections with fibrin sealant containing neurotrophic factors. Exp. Neurol. 181, 204–212.
Jun, S. B., Hynd, M. R., Dowell-Mesfin, N. M., Al-Kofahi, Y., Roysam, B., Shain, W., and Kim, S. J. (2008). Modulation of cultured neural networks using neurotrophin release from hydrogel-coated microelectrode arrays. J. Neural Eng. 5, 203–213.
Klinge, P. M., Vafa, M. A., Brinker, T., Brandis, A., Walter, G. F., Stieglitz, T., Samii, M., and Wewetzer, K. (2001). Immunohistochemical characterization of axonal sprouting and reactive tissue changes after long-term implantation of a polyimide sieve electrode to the transected adult rat sciatic nerve. Biomaterials 22, 2333–2343.
Lago, N., Udina, E., Ramachandran, A., and Navarro, X. (2007). Neurobiological assessment of regenerative electrodes for bidirectional interfacing injured peripheral nerves. IEEE Trans. Biomed. Eng. 54, 1129–1137.
Lee, S., Carvell, G. E., and Simons, D. J. (2008). Motor modulation of afferent somatosensory circuits. Nat. Neurosci. 11, 1430–1438.
Lefurge, T., Goodall, E., Horch, K., Stensaas, L., and Schoenberg, A. (1991). Chronically implanted intrafasicular recording electrodes. Ann. Biomed. Eng. 19, 197–207.
Leung, B. K., Biran, R., Underwood, C. J., and Tresco, P. A. (2008). Characterization of microglial attachment and cytokine release on biomaterials of differing surface chemistry. Biomaterials 29, 3289–3297.
Leventhal, D. K., and Durand, D. M. (2004). Chronic measurment of the stimulation sensitivity of the flat interface nerve electrode. IEEE Trans. Biomed. Eng. 51, 1649–1658.
Lin, L. R., and Huang, H. P. (1997). Mechanism and computer simulation of a new robot hand for potential use as an artificial hand. Artif. Organs 21, 59–69.
Lopez, C. A., Fleischman, A. J., Roy, S., and Desai, T. A. (2006). Evaluation of silicon nanoporous membranes and ECM-based microenvironments on neurosecretory cells. Biomaterials 27, 3075–3083.
Mannard, A., Stein, R. B., and Charles, D. (1974). Regenerative electrode units: implants for recording from single peripheral nerve fibers in freely moving animals. Science 183, 547–549.
Marasco, P. D., Schultz, A. E., and Kuiken, T. A. (2009). Sensory capacity of reinnervated skin after redirection of amputated upper limb nerves to the chest. Brain 132, 1441–1448.
Matrone, G. C., Cipriani, C., Secco, E. L., Magenes, G., and Carrozza, M. C. (2010). Principal components analysis based control of a multi-DoF underactuated prosthetic hand. J. Neuroeng. Rehabil. 7, 16.
Micera, S., and Navarro, X. (2009). Bidirectional interfaces with the peripheral nervous system. Int. Rev. Neurobiol. 86, 23–38.
Miller, L. A., Lipschutz, R. D., Stubblefield, K. A., Lock, B. A., Huang, H., Williams, T. W. III, Weir, R. F., and Kuiken, T. A. (2008). Control of a six degree of freedom prosthetic arm after targeted muscle reinnervation surgery. Arch. Phys. Med. Rehabil. 89, 2057–2065.
Misko, T. P., Radeke, M. J., and Shooter, E. M. (1987). Nerve growth factor in neuronal development and maintenance. J. Exp. Biol. 132, 177–190.
Nagahara, A. H., Merrill, D. A., Coppola, G., Tsukada, S., Schroeder, B. E., Shaked, G. M., Wang, L., Blesch, A., Kim, A., Conner, J. M., Rockenstein, E., Chao, M. V., Koo, E. H., Geschwind, D., Masliah, E., Chiba, A. A., and Tuszynski, M. H. (2009). Neurotrophic effects of brain-derived neurotrophic factor in rodent and primate models of Alzheimer’s disease. Nat. Med. 15, 331–337.
Oakley, R. A., Lefcort, F. B., Clary, D. O., Reichardt, L. F., Prevette, D., Oppenheim, R. W., and Frank, E. (1997). Neurotrophin-3 promotes the differentiation of muscle spindle afferents in the absence of peripheral targets. J. Neurosci. 17, 4262–4274.
Otr, O. V., Reinders-Messelink, H. A., Bongers, R. M., Bouwsema, H., and Van Der Sluis, C. K. (2010). The i-LIMB hand and the DMC plus hand compared: a case report. Prosthet. Orthot. Int. 34, 216–220.
Panetsos, F., Avendano, C., Negredo, P., Castro, J., and Bonacasa, V. (2008). Neural prostheses: electrophysiological and histological evaluation of central nervous system alterations due to long-term implants of sieve electrodes to peripheral nerves in cats. IEEE Trans. Neural Syst. Rehabil. Eng. 16, 223–232.
Phillips, C. A. (1988). Sensory feedback control of upper- and lower-extremity motor prostheses. Crit. Rev. Biomed. Eng. 16, 105–140.
Rangasamy, S. B., Soderstrom, K., Bakay, R. A., and Kordower, J. H. (2010). Neurotrophic factor therapy for Parkinson’s disease. Prog. Brain Res. 184, 237–264.
Romero, M. I., Lin, L., Lush, M. E., Lei, L., Parada, L. F., and Zhu, Y. (2007). Deletion of Nf1 in neurons induces increased axon collateral branching after dorsal root injury. J. Neurosci. 27, 2124–2134.
Romero, M. I., Rangappa, N., Garry, M. G., and Smith, G. M. (2001). Functional regeneration of chronically injured sensory afferents into adult spinal cord after neurotrophin gene therapy. J. Neurosci. 21, 8408–8416.
Romero, M. I., Rangappa, N., Li, L., Lightfoot, E., Garry, M. G., and Smith, G. M. (2000). Extensive sprouting of sensory afferents and hyperalgesia induced by conditional expression of nerve growth factor in the adult spinal cord. J. Neurosci. 20, 4435–4445.
Rosenzweig, E. S., Courtine, G., jindrich, D. L., Brock, J. H., Ferguson, A. R., Strand, S. C., Nout, Y. S., Roy, R. R., Miller, D. M., Beattie, M. S., Havton, L. A., Bresnahan, J. C., Edgerton, V. R., and Tuszynski, M. H. (2010). Extensive spontaneous plasticity of corticospinal projections after primate spinal cord injury. Nat. Neurosci. 13, 1505–1510.
Simeral, J. D., Kim, S. P., Black, M. J., Donoghue, J. P., and Hochberg, L. R. (2011). Neural control of cursor trajectory and click by a human with tetraplegia 1000 days after implant of an intracortical microelectrode array. J. Neural Eng. 8, 025027.
Tansey, K. E., Seifert, J. L., Botterman, B., Delgado, M. R., and Romero, M. I. (2011). Peripheral nerve repair through multi-luminal biosynthetic implants. Ann. Biomed. Eng. 39, 815–1828.
Velliste, M., Perel, S., Spalding, M. C., Whitford, A. S., and Schwartz, A. B. (2008). Cortical control of a prosthetic arm for self-feeding. Nature 453, 1098–1101.
Weber, D. J., Stein, R. B., Everaert, D. G., and Prochazka, A. (2007). Limb-state feedback from ensembles of simultaneously recorded dorsal root ganglion neurons. J. Neural Eng. 4, S168–S180.
Williams, J. C., Hippensteel, J. A., Dilgen, J., Shain, W., and Kipke, D. R. (2007). Complex impedence spectroscopy for monitoring tissue response to inserted neural implants. J. Neural. Eng. 4, 410–423.
Winter, J. O., Cogan, S. F., and Rizzo, J. F. III. (2007). Neurotrophin-eluting hydrogel coatings for neural stimulating electrodes. J. Biomed. Mater. Res. Part B Appl. Biomater. 81, 551–563.
Zhang, X., Aman, K., and Hokfelt, T. (1995). Secretory pathways of neuropeptides in rat lumbar dorsal root ganglion neurons and effects of peripheral axotomy. J. Comp. Neurol. 352, 481–500.
Appendix
Keywords: peripheral nerve, nerve regeneration, multielectrode array, sensory feedback, bionics, NGF, NT-3, neurotrophins
Citation: Lotfi P, Garde K, Chouhan AK, Bengali E and Romero-Ortega MI (2011) Modality-specific axonal regeneration: toward selective regenerative neural interfaces. Front. Neuroeng. 4:11. doi: 10.3389/fneng.2011.00011
Received: 18 July 2011;
Paper pending published: 15 August 2011;
Accepted: 26 September 2011;
Published online: 12 October 2011.
Edited by:
Gabriel A. Silva, University of California San Diego, USAReviewed by:
Anja Kunze, École Polytechnique Fédérale de Lausanne, SwitzerlandHari S. Sharma, Uppsala University, Sweden
Copyright: © 2011 Lotfi, Garde, Chouhan, Bengali and Romero-Ortega. This is an open-access article subject to a non-exclusive license between the authors and Frontiers Media SA, which permits use, distribution and reproduction in other forums, provided the original authors and source are credited and other Frontiers conditions are complied with.
*Correspondence: Mario I. Romero-Ortega, University of Texas at Arlington, 500 UTA Blvd., ERB 242, Box 19138, Arlington, TX 76019, USA. e-mail: mromero@uta.edu