- Laboratory for Neurophysiology, Department of Molecular Cell Biology, Leiden University Medical Center, Leiden, Netherlands
In mammals, the suprachiasmatic nucleus (SCN) functions as a circadian clock that drives 24-h rhythms in both physiology and behavior. The SCN is a multicellular oscillator in which individual neurons function as cell-autonomous oscillators. The production of a coherent output rhythm is dependent upon mutual synchronization among single cells and requires both synaptic communication and gap junctions. Changes in phase-synchronization between individual cells have consequences on the amplitude of the SCN’s electrical activity rhythm, and these changes play a major role in the ability to adapt to seasonal changes. Both aging and sleep deprivation negatively affect the circadian amplitude of the SCN, whereas behavioral activity (i.e., exercise) has a positive effect on amplitude. Given that the amplitude of the SCN’s electrical activity rhythm is essential for achieving robust rhythmicity in physiology and behavior, the mechanisms that underlie neuronal synchronization warrant further study. A growing body of evidence suggests that the functional integrity of the SCN contributes to health, well-being, cognitive performance, and alertness; in contrast, deterioration of the 24-h rhythm is a risk factor for neurodegenerative disease, cancer, depression, and sleep disorders.
Introduction
The rotation of the Earth around its central axis causes a daily rhythm in environmental factors, including light intensity, temperature, and food availability. In order to anticipate these 24-h changes in the environment, many species have evolved an internal clock. In mammals, this internal clock resides in the suprachiasmatic nucleus (SCN) in the ventral hypothalamus (1). The SCN is a bilateral structure containing 20,000 neurons that generate circadian rhythms. The SCN synchronizes its circadian rhythm to the external day–night cycle using light information that is projected via the retinohypothalamic tract (RHT). This information is then conveyed to other regions in the central nervous system (2, 3). Based on neuropeptide expression, the SCN is sub-divided into the dorsal (i.e., shell) and ventral (i.e., core) SCN (4–7). The ventral SCN expresses gastrin-releasing peptide (GRP) and vasoactive intestinal polypeptide (VIP) (1, 8), whereas the dorsal SCN contains the hormone vasopressin (9). Moreover, the dorsal SCN receives strong input from the ventral SCN (9), whereas the ventral SCN receives little input from the dorsal SCN (10).
Individual cells in the SCN generate a circadian rhythm via a series of interconnected positive and negative feedback loops; these feedback loops regulate the transcription and activity of clock genes and proteins, respectively (11, 12). One feedback loop is regulated by the transcription factors Circadian Locomotor Output Cycles Kaput (CLOCK) and Brain and Muscle ARNT-like protein 1 (Bmal1). These proteins drive the transcription of specific target genes in the Period (Per1, Per2, and Per3) and Cryptochrome (Cry1 and Cry2) gene families; in turn, Per and Cry proteins inhibit CLOCK/Bmal1-mediated transcription. Another feedback loop consists of the nuclear receptors ROR (α, β, and γ), PPARα, and REV-ERB (α and β). Dissociated SCN cells retain a circadian rhythm in their electrical firing rate, with a relatively wide range of intrinsic periods (ranging from 22 to 28 h) (13–15). This ability of isolated neurons to maintain their intrinsic rhythm indicates that individual SCN neurons function as autonomous single-cell oscillators driven by intrinsic molecular feedback loops (14). The key implication of this finding is that the SCN’s multicellular structure depends upon cooperation among individual neurons in order to function effectively as a coherent pacemaker. In this review, we will discuss the mechanisms that underlie synchronization, and we will discuss the relevance and significance of synchronization for health and disease. Next, we will discuss the consequences of disrupted SCN synchronization on aging, sleep disorders, neurodegenerative diseases, and metabolic disorders. In addition, positive effects of physical exercise on SCN rhythm amplitude will be discussed. Finally, we will compare the organization of the SCN of nocturnal and diurnal species with special focus on potential differences in synchronization mechanisms.
Synchronization of SCN Neurons
Phase Shifts in the SCN are Driven by Synchronized SCN Neurons
Both in vitro and in vivo recordings of SCN firing frequency revealed that the SCN’s electrical activity output has a sinusoidal-like waveform pattern that peaks during the subjective day and is low during the subjective night (16, 17). In nocturnal animals, the trough of the SCN’s electrical activity corresponds with the animal’s behaviorally active phase (18–20). In diurnal animals, this relationship between electrical activity and behavioral activity is reversed; thus, the SCN’s activity peaks in phase with the behaviorally active phase (21). Recordings in the SCN of freely moving mice revealed close correspondence between the SCN’s pattern of electrical activity and the animal’s behavioral activity. Specifically, the behavioral transitions from rest to activity – and vice versa – occur at the mid-point in the declining and increasing slopes in SCN activity, respectively (22). The most intense level of behavioral activity occurs during the trough in electrical activity rhythm, and silencing activity in the SCN by applying tetrodotoxin during the animal’s resting phase induces behavioral activity (23).
A subpopulation of SCN neurons (comprising 32 and 38% of SCN neurons in rats and hamsters, respectively) exhibit light-induced changes in electrical activity (24–29). At low intensities (0.1 lux in rats and 1 lux in hamsters), light can suppress electrical activity, whereas high light intensity increases the rate of neuronal firing in an intensity-dependent manner (25). In response to external light, the clock genes Per1 and Per2 are induced in the SCN (30–34), and the duration of this induction is dependent upon the intensity of the light (35). Glutamate is the primary neurotransmitter used by the RHT to project light information to the SCN (36). The application of glutamate – or agonists of the glutamate receptor – to the SCN causes a phase shift in the SCN’s electrical activity that mimics light-induced phase shifts in behavior (37–39) and alters the levels of Per1 and Per2 mRNA (34). The SCN’s rhythm is synchronized to the daily light–dark cycle by the phase-shifting effects of light on the SCN. Early in the subjective night, light has a phase-delaying effect; in contrast, light in the late subjective night causes a phase advance (40–42). These phase-dependent responses are fundamental to the animal’s ability to entrain to a new light cycle, and are present in all living organisms that exhibit circadian rhythmicity. Whether a light-induced phase delay or phase advance will occur depends upon intercellular signaling cascades and is therefore an intrinsic property of the SCN (43–46).
Following exposure to a shift in the light–dark cycle (for example, by crossing time zones), the SCN generally takes several cycles to resynchronize (47–52). Recordings of the SCN’s firing rate in the rat revealed that delay-shifting the light–dark cycle by 6 h induces a transient bimodal electrical activity rhythm in the SCN (47). One component of this bimodal activity pattern reflects the activity of a group of neurons that synchronize immediately to the new light–dark cycle, whereas the other component reflects the activity of neurons that remain synchronized to the previous light–dark cycle. Separation of the ventral SCN from the dorsal SCN by surgical incision revealed a unimodal electrical activity pattern in both regions and revealed that the shifted and non-shifted components are generated by the ventral and dorsal SCN, respectively. Surprisingly, following a shift in the light–dark cycle, the electrical activity profile of the ventral SCN (i.e., the shifted component) is considerably more narrow than the electrical activity profile of the dorsal SCN (i.e., the non-shifted component). Curve-fitting analysis revealed that the narrow, shifted component is composed of the electrical output produced by only 20% of the entire SCN’s neuronal population (53). Furthermore, simulations revealed that the ventral SCN’s narrow electrical activity profile is not due to the low number of neurons that contribute to this component, but is actually the result of high synchrony among these neurons (54). The differences in light-induced phase shifts between the ventral and dorsal SCN could be the result of differential innervation by the optic nerve (5, 55, 56). In rats, the ventral SCN receives the majority of light input projected by the retina (3, 6, 7) and has more pronounced light-evoked changes in terms of electrical activity (57, 58) and gene expression (59–64). In contrast, the dorsal SCN is only sparsely innervated by the retina (1).
Robustness of the SCN’s Output is Correlated with Synchrony among SCN Neurons
In vivo recordings of SCN electrical activity revealed that the SCN’s waveform pattern differs between long and short photoperiods (65). The changes in the SCN’s electrical activity waveform in response to a change in photoperiod correspond with observable behavioral adaptations. In both long and short photoperiods, the onset and offset of behavioral activity occur at the mid-point threshold in the declining and rising slopes of electrical activity, respectively (65). Even in continuous darkness, the SCN retains its intrinsic photoperiod-induced waveform for several cycles, suggesting that the SCN has a photoperiodic memory. Furthermore, the photoperiod-induced waveform is preserved even after the SCN has been isolated in vitro. After entraining to a short photoperiod, the SCN’s ensemble discharge rate has a waveform with a short duration of enhanced activity; in contrast, after entraining to a long photoperiod, the SCN’s waveform has a long period of enhanced activity (65, 66). Computational studies showed that changes in phase-synchronization are extremely effective in terms of inducing a change in waveform, whereas changes in single-cell activity patterns have relative weak effects (67, 68). Experimental studies have confirmed that nature indeed functions in this way. Both single-cell and subpopulation recordings revealed that after entraining to a short photoperiod, the activity of individual SCN neurons is more synchronized and clusters around subjective midday. On the other hand, after entraining to a long photoperiod, the activity of SCN neurons is less synchronized (Figure 1).
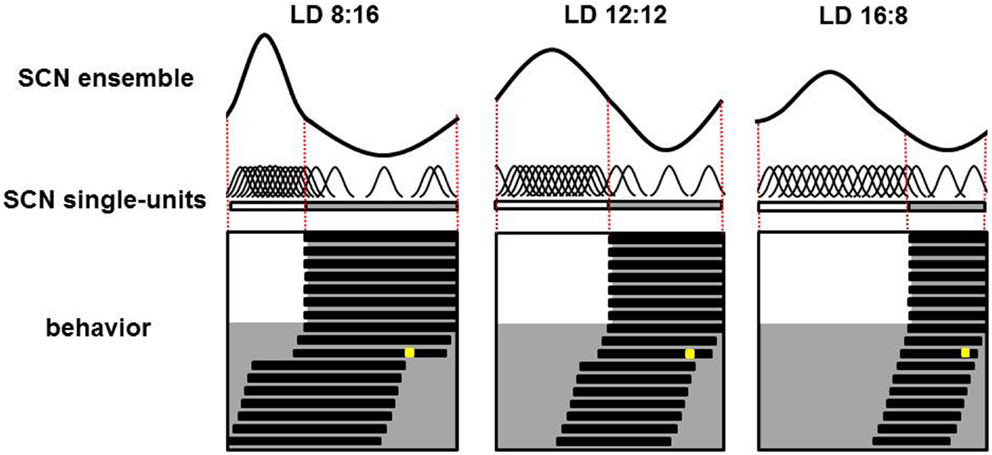
Figure 1. Plasticity of the SCN. Schematic overview of the SCN’s ensemble rhythm (top), single-cell activity in individual SCN neurons (middle), and the animal’s behavioral activity pattern (bottom) in a nocturnal rodent entrained to a short (LD 8:16; left column), medium (LD 12:12; middle column), or long (LD 16:8; right column) photoperiod. In the lower panels, days are depicted on successive lines, and light and dark periods are represented by white and gray backgrounds, respectively. Following a transition to continuous darkness, aftereffects of the photoperiod on behavioral activity can be detected. Following a short photoperiod, the animal’s active phase is longer; in contrast, following a long photoperiod, the active phase is shorter. A light pulse applied late in the subjective night in the third cycle of continuous darkness (the yellow square) results in a large-magnitude phase advance in animals entrained to a short photoperiod, a smaller phase advance in animals entrained to a medium photoperiod, and virtually no phase advance in animals entrained to a long photoperiod (phase advance is measured as the difference in activity onset before and following the light pulse). For each photoperiod, the SCN’s ensemble electrical activity rhythm is depicted in the top row. The relative amplitude of the SCN ensemble rhythm is large in short photoperiods and small in long photoperiods; this difference is due to different levels of synchronization among the electrical activity patterns of the individual neurons. The single-unit activity traces are highly synchronized in animals entrained to a short photoperiod, and less synchronized in animals entrained to a long photoperiod (middle row).
In the dorsal SCN, the electrical activity patterns differ somewhat between short and long photoperiods; however, computational studies revealed that these differences in single-cell activity patterns in the dorsal SCN are not sufficient to explain the waveform changes that occur at the network level (67, 69). However, the narrow phase distribution of subpopulation activity during short photoperiods does explain the narrow peak width in the SCN’s waveform, whereas the broad phase distribution during long photoperiods explains the broad peak width in the SCN’s waveform (65, 66). These findings are supported by molecular studies that show similar single-cell Per1 expression patterns after entrainment to either long or short photoperiods (70). The results from electrophysiological, computational, and molecular studies indicate that synchrony between individual SCN neurons – rather than a change in the activity pattern of those individual neurons – is important for adapting to a change in photoperiod.
Amplitude of the SCN’s Output Correlates with the SCN’s Phase-Shifting Response
In the 1980s, Pittendrigh and colleagues reported that the photoperiod to which hamsters were entrained affected the resulting amplitude of the phase-response curve. Thus, hamsters that were entrained to a short photoperiod had a larger light-induced phase shift compared to hamsters that were entrained to a long photoperiod (71). More recent studies support these early findings (72–74). The difference in the light-induced behavioral phase shift between short and long photoperiods is not the result of a difference in retinal response; rather, the difference occurs at the level of the SCN. Bath application of the glutamate receptor agonist N-methyl-d-aspartate (NMDA) to an SCN entrained to a short photoperiod causes a significantly larger phase delay compared to an SCN that was entrained to a long photoperiod (74). Moreover, the acute effect of applying a pulse of NMDA is similar between a short photoperiod-entrained SCN and a long photoperiod-entrained SCN, suggesting that the difference in the phase delay in the SCN is not caused by desensitization to glutamate in long photoperiods (74); thus, another mechanism must explain these results.
The amplitude of the SCN’s electrical rhythm is high when the neurons in the SCN are more synchronized (i.e., when entrained to a short photoperiod), whereas the amplitude of the rhythm is low when the neurons are less synchronized (54, 65, 67, 75). Results from both behavioral and in vitro studies revealed that an SCN with high amplitude exhibits a larger shift in response to a given perturbation compared to an SCN with a low rhythmic amplitude (i.e., from a long photoperiod) (74). These results are surprising, as they do not intuitively match predictions that arise from limit cycle oscillator theory, a theory that is often used to model the phase-shifting behavior of oscillators. The limit cycle model predicts that following a perturbation of a given magnitude, oscillators that oscillate with a high amplitude will shift to a lesser degree than oscillators that oscillate with a lower amplitude (71, 76, 77). This prediction holds true for primitive organisms such as Gonyaulax (78) and Neurospora (79, 80). However, the experimental finding that an SCN with a high-amplitude oscillation shifts to a greater extent than an SCN with a low-amplitude oscillation is not consistent with the predictions of limit cycle theory. This apparent discrepancy between theory and practice may be due to the SCN’s functioning at the level of a network. In a population of highly synchronized neurons, each individual neuron will be more in phase, and an external perturbation of the system will cause a similar phase-shifting response in the individual cells, thereby driving a large net shift in the SCN network. In a relatively desynchronized SCN, the individual neurons will be out of phase, and an external perturbation will induce different phase-shifting responses among the individual neurons, thereby causing a relatively small net shift in the SCN network (81). Simulations have confirmed this prediction with surprisingly high accuracy (62). In Afh/Afh mice, the amplitude of the SCN ensemble is reduced by a reduction of the amplitude of single-cell oscillations (82). In accordance to the limit cycle theory, the Afh/Afh mice show high-amplitude resetting to light. To explain light-resetting by the SCN, both the amplitude of single-cell oscillations as well as phase-synchronization among single cells should be taken into consideration.
Under certain conditions, the SCN – as a network – can behave as a limit cycle oscillator. Simulation studies showed that the phase-shifting response of the SCN is opposite to the predictions of a limit cycle oscillator if just a fraction of the network is directly influenced by the perturbation (e.g., if light affects only 20% of the population). On the other hand, if all of the neurons in the SCN network are affected by the perturbation (for example, a change in temperature, which would affect 100% of the neurons in the population), the SCN can exhibit the behavior predicted by limit cycle oscillator theory (83). Thus, although limit cycle theory accurately predicts the behavior of an individual oscillator, the phase-shifting behavior of an entire network of oscillators is more difficult to predict, as such behavior is dependent upon the degree of synchrony among the individual oscillators and the percentage of neurons that will respond to the perturbation (i.e., a 100% response rate to temperature vs. a 20% response rate to external light).
Role of Chemical Coupling in SCN Neuronal Synchronization
Several neurotransmitters play a role in the phase-synchronization of SCN neurons (Figure 2). For example, γ-aminobutyric acid (GABA) is the most prevalent neurotransmitter in the SCN. In the adult SCN, activation of GABAA receptors causes an inhibitory response (84). This inhibitory effect of GABAergic signaling often plays a role in synchronizing neuronal networks within the brain (13, 85–87). Examining synchronization in SCN slices in the presence or absence of a GABAA signaling blocker revealed that GABA plays a role in synchronizing SCN neurons. When slices prepared from a desynchronized SCN were treated with a GABAA signaling blocker, the SCN neurons remained desynchronized; in contrast, in the absence of the blocker, the neurons became synchronized again (88). Although GABA acts predominantly as an inhibitory transmitter in the adult brain, it can play an excitatory role when coupled with the activity of the NKCC1 chloride pump (89, 90). GABAergic transmission is also excitatory in the dorsal SCN (47, 90, 91), and this excitation may play a role in communication between the ventral and dorsal SCN (47). Recently, we reported that GABAergic excitatory transmission is more prevalent in a desynchronized SCN than in a synchronized SCN (40 vs. 28%, respectively) (92), which suggests that the inhibitory/excitatory ratio of GABAergic activity plays a role in the phase-synchronization of individual SCN neurons.
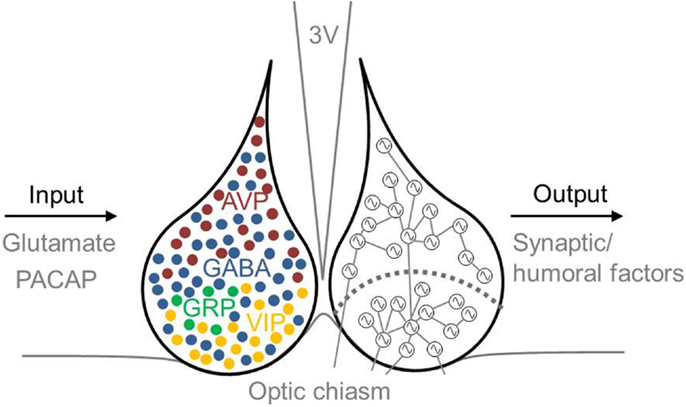
Figure 2. Schematic illustration of the mammalian SCN, including inputs and outputs. Input to the SCN is mediated by the neurotransmitters glutamate and PACAP, and output from the SCN is mediated by synaptic and humoral factors. The approximate locations of specific neurotransmitter-expressing neurons are indicated in the left nucleus. GABA (blue) is co-expressed in other neuronal cell types, including VIP- (yellow), AVP- (red), and GRP- (green) expressing neurons. AVP-expressing cells are located primarily in the dorsal SCN, and VIP-expressing cells are located primarily in the ventral SCN. The SCN depicted on the right schematically shows the ventral and dorsal SCN subdivision (the curved dotted line), and coupling between rhythmic SCN neurons is indicated by lines connecting the cells. Many neurons in the ventral SCN are directly innervated by afferent fibers arising from the optic chiasm, whereas far fewer neurons in the dorsal SCN receive direct light information input. The ventral SCN has direct input to the dorsal SCN, while the dorsal SCN is only sparsely innervated by the ventral aspect. Each neuron is depicted schematically as a cell-autonomous single-cell oscillator. 3V, third ventricle; AVP, vasopressin; GABA, γ-aminobutyric acid; GRP, gastrin-releasing peptide; PACAP, pituitary adenylate cyclase-activating polypeptide; SCN, suprachiasmatic nucleus; VIP, vasoactive intestinal peptide.
The primary neurotransmitter in the ventral SCN is VIP. Intrinsically photoreceptive retinal ganglion cells (ipRGCs) contain the photopigment melanopsin and convey light information to the SCN via the RHT (93–95). VIP-containing neurons process light information received from the RHT and then transfer this information to the dorsal SCN (45, 96). The RHT contains both glutamate and pituitary adenylate cyclase-activating polypeptide (PACAP). The application of glutamate has an excitatory effect on SCN neurons, whereas glutamate receptor antagonists inhibit light-induced responses both in vivo and in vitro (97). Eliminating glutamate from ipRGCs impairs photo-entrainment of behavioral rhythm in mice (98); similarly, eliminating PACAP or its receptor also impairs photo-entrainment (99, 100). Although eliminating VIP reduces the light-induced upregulation of the clock gene Per1, photic induction of Per1 is unimpaired in PACAP-deficient mice (99). Thus, VIP-expressing cells are critically important for relaying externally received light information to the SCN network. Eliminating VIP or the VIP receptor (VIP2R, also known as VPAC2) reduces SCN electrical activity (101), molecular rhythms (102, 103), and behavioral rhythms (104–107). In contrast, application of VIP mimics light-induced responses in the SCN (108, 109), drives long-lasting increased electrical activity in dorsal SCN neurons (110), and restores synchrony among SCN neurons in VIP-knockout mice (102–104). Finally, the SCN in VIP-knockout mice does not exhibit photoperiod adaptation. Taken together, these compelling findings indicate that VIP is important both for SCN neuronal synchronization and for the ability of the SCN to encode photoperiod-related information (111).
A subpopulation of neurons in the ventral SCN express GRP, and these neurons are important for conveying information regarding external light throughout the SCN (5, 8, 102, 103). In Syrian hamsters, in vivo microinjections of GRP into the third ventricle induces the expression of c-fos, Per1, and Per2 in the dorsal SCN (8). GRP receptor-knockout mice have reduced light-induced phase shifts and reduced induction of Per and c-fos-expression in the dorsal SCN (112). The in vitro application of GRP to SCN slices induces a light-like phase shift in the SCN (113); moreover, applying GRP to SCN slices from VIP receptor-knockout mice increases synchrony among SCN neurons (5, 114).
The majority of neurons in the dorsal SCN express the neuropeptide vasopressin (AVP). This expression is rhythmic and is driven by the intrinsic molecular feedback loop in the core clock machinery. The in vitro application of AVP to SCN neurons isolated from VIP-deficient mice restores the rhythmicity and synchrony of the neurons (103). Furthermore, the expression pattern of AVP is different after entraining to a long photoperiod (i.e., in a desynchronized network) than after entraining to a short photoperiod (i.e., in a more synchronized network) (115, 116).
Connectivity within the SCN network is surprisingly plastic. In addition to seasonal plasticity, the SCN also exhibits a daily rhythm of synaptic connectivity. Measuring the firing rates of individual SCN neurons in the presence or absence of GABAA receptor antagonists revealed that SCN connectivity is dependent upon GABAergic communication, and the strength of this connectivity can change in a matter of days or even hours (117). Recently, confocal microscopy studies revealed that synaptic changes occur in VIP-expressing neurons in the SCN’s retinorecipient region, but not in AVP-expressing neurons in the non-retinorecipient region (118). The authors hypothesized that remodeling of the synaptic connectivity in VIP-expressing neurons over a 24-h cycle might contribute to the ability of these neurons to adapt to light, thereby increasing the efficacy of photic transmission (118). In addition, during the subjective night, the neuron–glia network in the SCN undergoes morphological rearrangements (119), and during the day, axon terminal coverage of VIP-expressing neurons increases (119). These findings indicate that the SCN is a remarkably plastic structure that can efficiently adapt its network structure in response to changes in functional needs.
Role of Electrical Coupling in Synchronization of SCN Neurons
The SCN network contains a large number of gap junctions that mediate electrical synchronization among SCN neurons (19, 120–123). Gap junction-mediated coupling improves the connectivity of neuronal networks (124, 125) and plays a role in the synchronization of several brain areas (121, 126–131). In the absence of chemical synaptic transmission, 24–26% of SCN neurons in rodents exhibit synchronous electrical activity (28, 121, 132); moreover, tracer-coupling experiments showed that 30% of SCN neurons are coupled via gap junctions (28, 120). In mice, gap junctions couple neurons in both the ventral and dorsal SCN, and clusters of coupled cells are restricted to each subdivision (120). In the mammalian CNS, the protein connexin-36 (Cx36) is a key component of gap junctions (131), and Cx36-knockout mice have significantly reduced electrical coupling in the SCN (121). Moreover, Cx36-knockout mice that were housed in continuous darkness had significantly reduced wheel-running activity compared to wild-type mice housed under the same conditions, suggesting that gap junctions play a role in the circadian organization of locomotor activity rhythms (121). Although immunofluorescence microscopy and immunogold labeling experiments confirmed that Cx36 is expressed abundantly in the postnatal SCN, tracer coupling and electrical coupling (i.e., coupled spiking) is relatively weak between SCN neurons in adult animals (122) compared to young animals (28, 120, 121). Interestingly, recent findings suggest that VIP increases gap junction-mediated coupling, as the application of VIP increased coupling efficiency between SCN neurons in which chemical coupling was blocked (123). This recent result supports the notion that the balance between chemical and electrical communication in the SCN is important for modulating synchronous rhythms, and disruption of only one form of communication can disrupt the circadian rhythmicity of the SCN and the periphery.
SCN Neuronal Synchrony is Disrupted in Aging and Disease
Aging and the SCN
With aging, many species – including humans – experience changes in circadian timing. These changes are manifested as a reduction in the behavioral activity rhythm and in disruptions of the sleep–wake cycle (133–136). Given that the output of the SCN drives rhythms in behavior and physiology, these age-related disturbances in circadian rhythmicity could be caused by age-related deficits in the SCN. In support of this notion, transplanting fetal SCN tissue into the anterior hypothalamus of aged animals improves circadian rhythmicity in both hamsters (137, 138) and rats (139). In vivo recordings of electrical activity revealed reduced circadian amplitude in the SCN of middle-aged mice compared to young mice. Moreover, the amplitude of electrical activity rhythm in the subparaventricular zone – which receives input from the SCN – is substantially reduced in aged animals (140). Specifically, the amplitude of the SCN’s electrical activity rhythm in aged animals is approximately half of the amplitude in young animals (136) (Figure 3). This reduced amplitude cannot be explained simply by a loss of SCN neurons in aged mice (141) or rats (142), and experimental data suggest that age-related deficits in coupling between SCN neurons underlies the decrease in amplitude (136, 143).
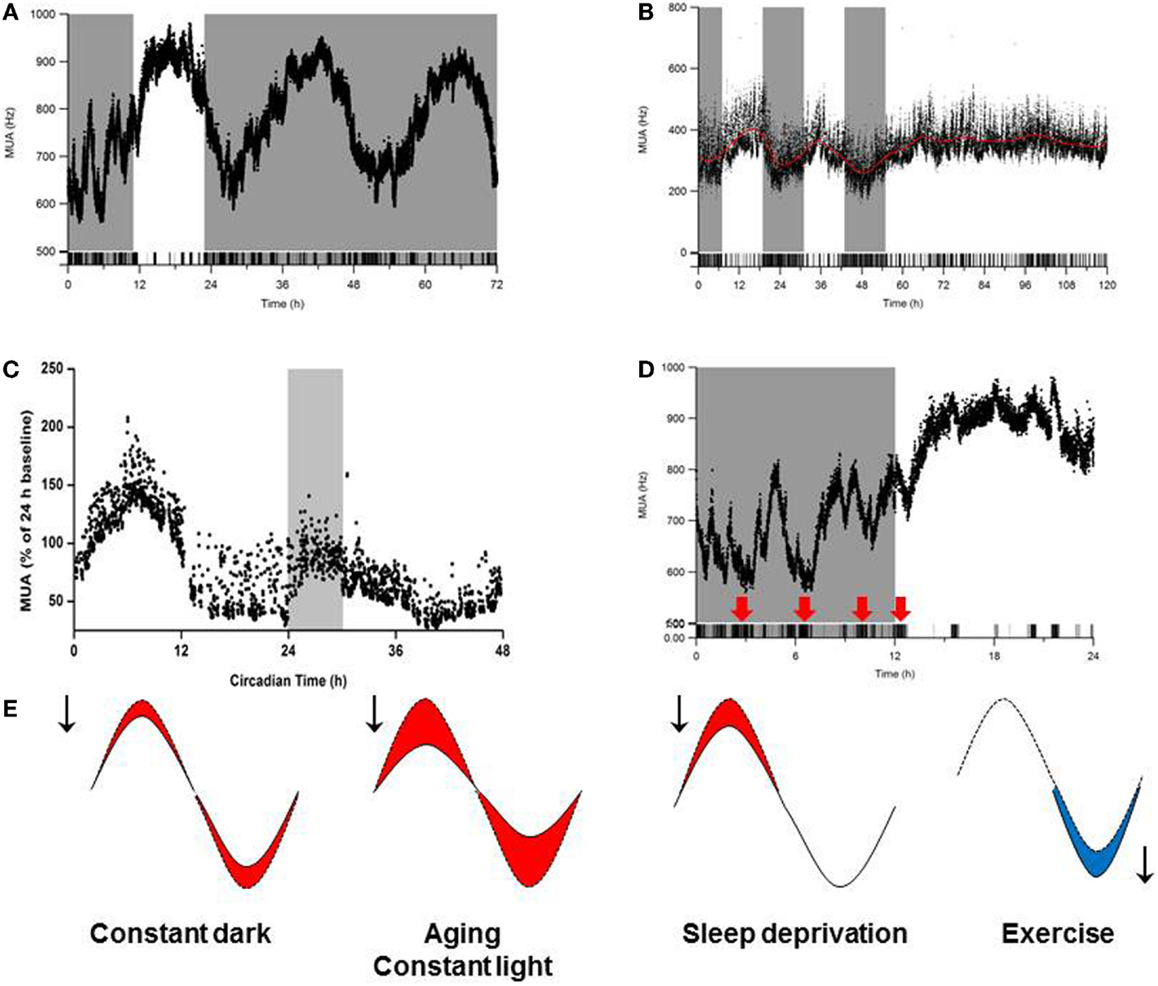
Figure 3. Influence of environmental conditions on SCN amplitude. Effects of (A) continuous darkness (212), (B) continuous light (212), (C) sleep deprivation (170), and (D) behavioral activity/physical exercise (254) on SCN neuronal activity measured using long-term in vivo recording of the SCN in freely moving mice. In (A–D), the x-axis indicates (circadian) time (in hours), and the y-axis shows the electrical activity of the SCN ensemble (MUA, or multiunit activity) recording. (E) Schematic summary of the effects of continuous darkness, continuous light, sleep deprivation, and behavioral activity (exercise) on SCN neuronal activity. The red and blue areas under the curves represent a decrease or increase, respectively, in the SCN’s rhythm amplitude.
Experiments have also revealed that SCN neurons in aged animals have an altered pattern of electrophysiological activity (136, 143–147). For example, the activity patterns of individual cells are less synchronized in the SCN of aged mice compared to young mice, and SCN neurons in aged mice even have anti-phasic activity (136). Computational studies found that decreased coupling in the aged SCN can lead to reduced synchrony among SCN neurons, thereby reducing the amplitude of the SCN’s network output (148). Physiologically, aging causes a clear change in the expression of neurotransmitters in the SCN. For example, the number of VIP- and AVP-expressing neurons is decreased in the SCN of aged rats (142, 149). In addition, the amplitudes of the circadian expression levels of VIP mRNA (150) and VPAC2 mRNA (151) decline with aging. Functionally, in vitro patch-clamp recordings of SCN neurons from aged mice revealed that postsynaptic GABAergic currents are lower in both frequency (143) and amplitude (136) compared to recordings from young SCN neurons. Furthermore, the number of GABAergic synaptic terminals in the SCN of aged mice is reduced by 26% compared to young animals (152). These findings are relevant to humans as well, as neurotransmission decreases with aging. In elderly people, the number of VIP-expressing SCN neurons is reduced (153), and vasopressin levels are reduced in the SCN of elderly people from the age of 80 years (154). This age-associated decline in neurotransmission in the SCN could affect both the quality and degree of synchronization among SCN neurons.
Theoretically, a decline in the amplitude in the SCN’s rhythm can result from a decrease in synchronization and/or from a decrease in the amplitude of individual neurons (148). In mice, an age-related decrease in the SCN ensemble rhythms of Per2 (155), Clock, and Bmal1 expression (156–158) have been reported. SIRT1 is an NAD-dependent protein deacetylase that activates transcription of the Clock and Bmal1 genes, thereby regulating circadian rhythms in tissues. In mice, an age-related decrease in SIRT1 levels in the brain corresponds with decreased levels of BMAL1 and PER2 and is associated with a longer intrinsic period, disrupted behavioral activity patterns, and impaired light entrainment (158). Several studies reported robust rhythms in Per1 and Per2 expression in the SCN of aged animals (159), and an increased amplitude of Per2 expression has been reported in the SCN of aged mice (160).
Sleep Deprivation and the SCN
The sleep–wake cycle is regulated by both the circadian clock and a homeostatic mechanism that regulates sleep homeostasis (161). Homeostatic sleep pressure is reflected on an electroencephalogram (EEG) as slow-wave activity (SWA) during non-rapid eye movement (NREM) sleep. This homeostatic process can be manipulated by sleep deprivation; for example, sleep deprivation causes an increase in SWA in rats, hamsters, birds, and humans (162–168). During NREM sleep, SWA is negatively correlated with electrical activity in the SCN (169). Moreover, spontaneous transitions between NREM and REM sleep occur simultaneously with changes in SCN electrical activity (169), suggesting that sleep deprivation can directly affect the amplitude of the SCN’s electrical activity rhythm. This hypothesis was tested by performing simultaneous in vivo measurements of EEG and SCN firing rate in sleep-deprived rats. After 6 h of sleep deprivation, both SWA NREM sleep and REM sleep were significantly increased, and the SCN’s electrical activity was significantly decreased (170). These effects of sleep deprivation on SCN activity were long-lasting and could be measured at least 6 h after sleep deprivation (170) (Figure 3). In humans, disruptions in the quality and/or timing of sleep are common among the elderly and among individuals with neurodegenerative disorders (see below).
Neurodegenerative Disorders and the SCN
Patients with neurodegenerative disorders such as Alzheimer’s disease (AD), Huntington’s disease (HD), and Parkinson’s disease (PD) often exhibit perturbations in their 24-h activity patterns and in their sleep–wake cycles. Moreover, the expression patterns of clock genes are altered in the brains of patients with these neurodegenerative disorders compared to healthy subjects (171). In patients with AD, the loss of AVP is generally correlated with the severity of symptoms, suggesting a causal relationship (172). The 3xTg-AD mouse model of AD has disrupted circadian behavior and a decrease in VIP- and AVP-expressing neurons in the SCN (173, 174). Another model of AD, the APPxPS1 mouse, has disrupted sleep–wake rhythms compared to wild-type mice, but only a modest change in Per2 expression in the SCN (175). R6/2 mice, a model of HD, develop progressively severe disruptions in behavior rhythms as the disease progresses (176), despite normal electrical output from the SCN (177). Two mouse models of HD and PD (the BACHD and ASO mouse lines, respectively) have disrupted circadian rhythms in locomotor activity, heart rate, and body temperature (178, 179). These two mouse lines also have reduced single-cell electrical activity in the SCN, although Per2 expression is unaffected (178, 179).
Fragile X syndrome is the most common form of inherited mental retardation. Fragile X syndrome is caused by silencing of the FMR1 gene and the subsequent loss of fragile X mental retardation protein (FMRP, also known as FXR1P) (180). Patients with fragile X syndrome suffer from sleep disorders (181, 182), and mice that lack either FMRP or FXR2P show a complete lack of behavioral rhythmicity (183). Interestingly, the SCN in Fmr1/Fxr2 double-knockout mice have normal expression rhythms of the Per1, Per2, Bmal1, and Cry1 genes, and the SCN has high-amplitude electrical activity output (183). Molecular and electrophysiological data show that the SCN in the various mouse models of AD, HD, PD, and fragile X syndrome is functional, suggesting that the cause of the disease-associated perturbations in behavioral rhythmicity lie downstream of the SCN.
Metabolic Disorders and the SCN
Exposure to light during the subjective night alters the circadian rhythm of melatonin and cortisol levels (184, 185) and can alter sleep patterns (186, 187). Shift workers often develop severe circadian disruptions due to irregular timing of light exposure, which can increase the risk of developing type 2 diabetes or other conditions (188–191). Animal studies revealed that disrupting the circadian system by exposing animals to a shifted light–dark cycle or continuous light causes a range of symptoms that are similar to the effects of aging, including sleep disorders (192, 193), cardiovascular disorders (194–196), cognitive difficulties (197), and metabolic deficits (198–200). CLOCK-knockout mice have a dampened feeding rhythm and develop obesity and a host of other symptoms that are associated with metabolic disorder (199). The SCN plays a role in the regulation of energy homeostasis in both mice (201) and rats (202), and controls rhythms in energy metabolism by modulating rhythms in plasma glucose levels (203, 204), lipogenesis and lipolysis (205–207), and plasma leptin levels (208). Thermal ablation of the SCN in wild-type mice disrupts the circadian rhythmicity of energy intake, activity, and energy expenditure (209), as well as a loss of rhythm in plasma leptin levels (208). Lesioning the SCN induces mild obesity compared to sham-operated mice; however, hepatic insulin sensitivity decreases considerably, and basal glucose levels increase (209).
Repeated exposure to light during the subjective night causes increased body weight in mice (210, 211), and continuous exposure to light results in the complete loss of circadian rhythmicity in both energy metabolism and insulin sensitivity (212). Continuous exposure to light also reduces the amplitude of the SCN’s rhythm via desynchronization among the SCN neurons (213, 214). In vivo recordings of electrical activity in the SCN of freely moving mice that were continuously exposed to light revealed a 50% reduction in amplitude (212) (Figure 3). Remarkably, mice that are exposed continuously to light and fed a regular diet gain more weight than mice that are exposed to a standard light–dark cycle (12 h light:12 h dark) and fed a high-fat diet (212). A mixed-model analysis revealed that the reduction in the amplitude of the SCN’s output has a more severe effect on body weight than consuming a high-fat diet, thus underscoring the importance of robust SCN output for maintaining health. Disruptions in circadian rhythm and obesity are interrelated, and each disorder exacerbates the other, as a high-fat diet has a severely negative effect on the SCN’s ability to synchronize in response to light (215). Mice that genetically lack leptin (ob/ob mice) develop obesity and have altered phase-delaying capacity compared to heterozygous (ob/+) mice (216). Injecting ob/ob mice with leptin normalizes the photic response of the SCN, suggesting that leptin modifies light-induced responses in the SCN via an indirect pathway (216).
Behavioral Activity and the SCN
In addition to external light, several other stimuli provide input to the clock, and these inputs are known as non-photic stimuli. Examples include wheel-running activity (217), social interactions (218), dark pulses (219), and sleep deprivation (220). In general, non-photic stimuli induce behavioral activity, suggesting either that behavioral activity actually induces phase-shifting responses in the SCN (221) or that behavioral activity activates the same pathways. Interestingly, substances that are known to induce behavioral activity and/or influence neurotransmitter pathways involved in non-photic resetting – such as neuropeptide Y (NPY) (222), serotonin agonists (223), opioids (224), and short-acting benzodiazepines (225, 226) – induce a phase shift in the SCN’s rhythm.
The SCN receives its major inputs from two afferent pathways; the serotonergic tract provides input from the raphe nuclei, and the geniculohypothalamic tract provides input from the intergeniculate leaflet (IGL). Ablation of the serotonergic afferent SCN pathways attenuates the phase-shifting effects of several non-photic cues (227–230); moreover, increased levels of behavioral activity increase the levels of serotonin in both the SCN and the IGL (231, 232). In addition to behavioral activity, a phase shift can also be induced by arousal, and serotonin appears to play an important role in arousal-induced phase-shifting (230, 233). The geniculohypothalamic tract provides input to the SCN via the neurotransmitters NPY, GABA, enkephalin, and neurotensin (234–237). In rodents, wheel-running activity can induce the release of NPY in the SCN (238). The delivery of anti-NPY antibodies to the SCN attenuates the phase shift induced by novel wheel-running activity (239) and increases light-induced phase shifts (240). This finding suggests that NPY plays a role in both photic and non-photic resetting in the SCN (240).
Some non-photic stimuli suppress expression of Per1 and/or Per2 during the subjective day (241–245), and this suppression may underlie the SCN’s phase-shifting response to non-photic stimuli at the molecular level. Applying NPY (246–249) or the serotonin receptor agonist 8-OH-DPAT (250, 251) to SCN neurons in vitro decreases the neurons’ firing rate. At the in vivo level, recordings in the SCN of freely moving hamsters (252), rats (253), and mice (254) revealed that behavioral activity induces an immediate suppression of the SCN’s firing rate.
In mice, behaviorally induced suppression of SCN electrical activity is superimposed on the SCN’s circadian rhythm and occurs at all phases of the circadian cycle (254). The magnitude and duration of the suppression depend upon the intensity and duration of the behavioral activity, respectively. Even an ultra-short (i.e., briefer than 1 min) bout of behavioral activity can suppress the SCN’s firing rate to a rate similar to the rate achieved with longer bouts of activity. Less intense behaviors such as grooming, eating, drinking, and rearing can suppress electrical activity in the SCN by ~30%, and more intense activity such as locomotor activity can decrease electrical activity by up to 60% (254). The suppression in firing rate remains stable throughout the entire bout of behavioral activity, and switching between types of behavior (for example, from less intense activity to more intense activity) causes a change in the level of suppression. Because mice are nocturnal animals, their behavioral activity increases during the night; consequently, behaviorally induced suppressed firing decreases the trough of the SCN rhythm even further. On the other hand, during the day, mice are relatively inactive; consequently, the firing rate of the SCN is only barely suppressed during the peak of the rhythm. When a nocturnal animal is active during its resting phase, the peak in the SCN’s rhythm is lower, thereby resulting in a lower amplitude SCN rhythm. These studies suggest that scheduled behavioral activity can increase the SCN’s rhythmic amplitude, thereby improving peripheral rhythmicity. In support of this notion, voluntary exercise in aged mice increases the amplitude of the SCN’s firing rate in vitro and improves resynchronization of the SCN and peripheral systems to the light–dark cycle (255). Furthermore, mice lacking VIP or the VIP receptor show improved behavioral activity in response to scheduled locomotor activity (256, 257), and voluntary exercise improves circadian behavioral rhythmicity in a mouse model of HD (258). In humans, physical exercise accelerates the synchronization of sleep–wake rhythms to the external light–dark cycle (259) and improves health, mood, and performance (260–266).
Comparison of the SCN between Nocturnal and Diurnal Animals
A long-standing – and inadequately addressed – question is whether the circadian clock is organized similarly or differently between nocturnal and diurnal animals. A comparison of the neuronal networks in the SCN of diurnal (Acomys russatus) and nocturnal (Acomys cahirinus) spiny mice revealed no differences with respect to neurotransmitter localization, SCN subdivisions, input fibers, or output fibers (267). Similar to nocturnal species, diurnal species have circadian rhythmicity in clock gene expression and electrical activity profiles in the SCN (268–278). On the other hand, diurnal and nocturnal species differ with respect to photic responses and photoperiod encoding. In the SCN of nocturnal species, ~25% of neurons are excited by light, whereas a smaller percentage of neurons are inhibited or silenced completely (29, 57, 279). In the SCN of diurnal species such as ground squirrels (280) and degus (281), the percentage of light-responsive cells is lower (~10%), and within the group of light-responsive cells, the proportion of light-suppressed cells is higher. Under LD 12:12, the overall rhythm in Fos-expression is similar between the nocturnal rat and the diurnal grass rat, however the spatial distribution of the Fos-expressing neurons differs. Whereas Fos-expression occurs in 40% of GRP containing SCN neurons in nocturnal rats (282), less than 1% of the GRP neurons in diurnal grass rats show Fos-expression (283). On the other hand, in grass rats, ~30% of AVP containing SCN neurons express Fos, while Fos-expression in AVP neurons in nocturnal rats is uncommon (284–286). Photoperiod studies suggest that light has a synchronizing effect on clock gene profiles and neuronal electrical activity in the SCN of mice, rats, and hamsters (65, 66, 287–291). However, the expression profiles of Per1 and Per2 in the SCN of diurnal grass rats do not adapt to short photoperiods (292). The SCN of diurnal and nocturnal species show a differential phase window for serotonin-mediated phase resetting (293). In nocturnal species, injections of serotonin receptor agonists 8-OH-DPAT or (+)8-OH-DPAT cause large phase advances of behavioral activity only during subjective midday (241, 294, 295) while in the diurnal Arvicanthis, injections of (+)8-OH-DPAT induce small phase advances during the subjective night (293). Importantly, in diurnal Arvicanthis, serotonin agonists strengthen, instead of oppose, the effects of light on the circadian system, which could be clinically relevant (293).
The SCN of nocturnal mice and diurnal grass rats respond differently to GABA. Activating GABAA receptors during the subjective day causes a phase advance in the SCN of mice, whereas the SCN of grass rats exhibits a phase delay (296). In the SCN of mice, the rhythms in GRP and VIP are strengthened following continuous exposure to darkness. In contrast, in the SCN of diurnal grass rats, these rhythms do not become stronger; rather, they show a shift in their peak time of expression (297). Moreover, in the SCN of three-striped South Indian squirrels (Funambulus palmarum, a diurnal species), the phase relation of daily VIP and AVP rhythms differ from those found in nocturnal species (298). Recently, a study of the SCN in capuchin monkeys confirmed the presence of circadian oscillations in PER2 in the SCN of primates (299). Moreover, VIP-containing cells are present in the ventral SCN of capuchin monkeys (299), similar to VIP expression in nocturnal rodents (1, 3, 300, 301). In addition, AVP is present in both the ventral and dorsal SCN in capuchin monkeys (299), which is strikingly different than the expression patterns in the SCN of most nocturnal species studied to date (1, 3, 301–303).
Thus, the molecular clock is an evolutionarily preserved structure, and the SCN has both structural and functional similarities between nocturnal and diurnal species. On the other hand, the SCN of nocturnal and diurnal species differ with respect to their responsiveness to light, photoperiod, and neurotransmitters. These differences suggest fundamental differences between nocturnal and diurnal animals with respect to neuronal coupling in the SCN and synchronization mechanisms. These key differences should be considered carefully when making recommendations to improve the well-being of humans based largely on results obtained from studying nocturnal species.
Summary
The functioning of the SCN depends on its intrinsic molecular machinery, as well as on its organization at the network level. Communication and synchronization among SCN neurons is essential for generating a robust rhythm at the SCN’s tissue level which is transmitted to other brain structures, thereby impacting many of our bodily functions. The SCN is influenced by environmental factors, the most important of which is light. In a short photoperiod, the SCN’s electrical activity rhythm is robust due to highly synchronized single-cell activity patterns, while in a long photoperiod, the SCN’s electrical output is dampened by reduced synchrony among individual cells. Photoperiod-induced changes in the expression of clock genes generally coincide with photoperiod-induced changes in the SCN’s electrical rhythm. Despite this similarity, spatial differences exist with respect to SCN clock gene expression, while the electrical activity rhythm has only small spatial differences among various regions within the SCN. With aging, the amplitudes of both single-cell rhythms and ensemble rhythms decline; however, reports of the amplitude of clock gene expression patterns in the aged SCN have been inconclusive. These points lead to the fundamental question of how the molecular feedback loop is correlated with the SCN’s electrical output.
The SCN clock is part of a larger brain network that includes areas involved in the sleep–wake cycle, energy metabolism, and behavioral activity. A dampened SCN rhythm is associated with reduced amplitude of the behavioral activity rhythm and can lead to metabolic disorders; vice versa, a disrupted behavioral rhythm (for example, induced by shift work or by food intake during the resting phase) can adversely affect the amplitude of the SCN’s rhythm. Increased levels of behavioral activity and voluntary exercise have been identified as potential strategies to boost the amplitude of the SCN’s rhythm, presumably by increasing cellular synchronization at the ensemble level. To be therapeutically effective for humans, it is important to investigate the effects of exercise on the SCN in diurnal species, particularly given that some differences have been found between nocturnal and diurnal animals. Whether – and how – the peripheral systems involved in behavioral activity and food intake influence the SCN in diurnal mammals remains largely unknown. Increasing our knowledge of the interplay between the SCN and the periphery in diurnal animals will increase our understanding of circadian-related disorders in humans and might lead to novel, effective recommendations for improving lifestyle patterns.
Conflict of Interest Statement
The authors declare that the research was conducted in the absence of any commercial or financial relationships that could be construed as a potential conflict of interest.
Acknowledgments
We thank Hester van Diepen and Dr. Tom de Boer for providing figures for this review and Dr. Erno Vreugdenhil for his useful comments. This work is supported by the Netherlands Organization for Scientific Research, complexity grant (no. 645.000.010).
References
1. Abrahamson EE, Moore RY. Suprachiasmatic nucleus in the mouse: retinal innervation, intrinsic organization and efferent projections. Brain Res (2001) 916:172–91. doi:10.1016/S0006-8993(01)02890-6
2. Kalsbeek A, Palm IF, La Fleur SE, Scheer FA, Perreau-Lenz S, Ruiter M, et al. SCN outputs and the hypothalamic balance of life. J Biol Rhythms (2006) 21:458–69. doi:10.1177/0748730406293854
3. Morin LP, Allen CN. The circadian visual system, 2005. Brain Res Rev (2006) 51:1–60. doi:10.1016/j.brainresrev.2005.08.003
4. Antle MC, Silver R. Orchestrating time: arrangements of the brain circadian clock. Trends Neurosci (2005) 28:145–51. doi:10.1016/j.tins.2005.01.003
5. Gamble KL, Allen GC, Zhou T, McMahon DG. Gastrin-releasing peptide mediates light-like resetting of the suprachiasmatic nucleus circadian pacemaker through cAMP response element-binding protein and Per1 activation. J Neurosci (2007) 27:12078–87. doi:10.1523/JNEUROSCI.1109-07.2007
6. Ibata Y, Takahashi Y, Okamura H, Kawakami F, Terubayashi H, Kubo T, et al. Vasoactive intestinal peptide (VIP)-like immunoreactive neurons located in the rat suprachiasmatic nucleus receive a direct retinal projection. Neurosci Lett (1989) 97:1–5. doi:10.1016/0304-3940(89)90129-8
7. Kiss J, Csaki A, Csaba Z, Halasz B. Synaptic contacts of vesicular glutamate transporter 2 fibres on chemically identified neurons of the hypothalamic suprachiasmatic nucleus of the rat. Eur J Neurosci (2008) 28:1760–74. doi:10.1111/j.1460-9568.2008.06463.x
8. Antle MC, Kriegsfeld LJ, Silver R. Signaling within the master clock of the brain: localized activation of mitogen-activated protein kinase by gastrin-releasing peptide. J Neurosci (2005) 25:2447–54. doi:10.1523/JNEUROSCI.4696-04.2005
9. Moore RY, Speh JC, Leak RK. Suprachiasmatic nucleus organization. Cell Tissue Res (2002) 309:89–98. doi:10.1007/s00441-002-0575-2
10. Romijn HJ, Sluiter AA, Pool CW, Wortel J, Buijs RM. Evidence from confocal fluorescence microscopy for a dense, reciprocal innervation between AVP-, somatostatin-, VIP/PHI-, GRP-, and VIP/PHI/GRP-immunoreactive neurons in the rat suprachiasmatic nucleus. Eur J Neurosci (1997) 9:2613–23. doi:10.1111/j.1460-9568.1997.tb01691.x
11. Buhr ED, Takahashi JS. Molecular components of the Mammalian circadian clock. Handb Exp Pharmacol (2013) 217:3–27. doi:10.1007/978-3-642-25950-0_1
12. Herzog ED, Takahashi JS, Block GD. Clock controls circadian period in isolated suprachiasmatic nucleus neurons. Nat Neurosci (1998) 1:708–13. doi:10.1038/3708
13. Shirakawa T, Honma S, Katsuno Y, Oguchi H, Honma KI. Synchronization of circadian firing rhythms in cultured rat suprachiasmatic neurons. Eur J Neurosci (2000) 12:2833–8. doi:10.1046/j.1460-9568.2000.00170.x
14. Welsh DK, Logothetis DE, Meister M, Reppert SM. Individual neurons dissociated from rat suprachiasmatic nucleus express independently phased circadian firing rhythms. Neuron (1995) 14:697–706. doi:10.1016/0896-6273(95)90214-7
15. Welsh DK, Takahashi JS, Kay SA. Suprachiasmatic nucleus: cell autonomy and network properties. Annu Rev Physiol (2010) 72:551–77. doi:10.1146/annurev-physiol-021909-135919
16. Inouye ST, Kawamura H. Persistence of circadian rhythmicity in a mammalian hypothalamic “island” containing the suprachiasmatic nucleus. Proc Natl Acad Sci U S A (1979) 76:5962–6. doi:10.1073/pnas.76.11.5962
17. Shibata S, Oomura Y, Kita H, Hattori K. Circadian rhythmic changes of neuronal activity in the suprachiasmatic nucleus of the rat hypothalamic slice. Brain Res (1982) 247:154–8. doi:10.1016/0006-8993(82)91041-1
18. Brown TM, Piggins HD. Electrophysiology of the suprachiasmatic circadian clock. Prog Neurobiol (2007) 82:229–55. doi:10.1016/j.pneurobio.2007.05.002
19. Colwell CS. Linking neural activity and molecular oscillations in the SCN. Nat Rev Neurosci (2011) 12:553–69. doi:10.1038/nrn3086
20. Gillette MU, McArthur AJ. Circadian actions of melatonin at the suprachiasmatic nucleus. Behav Brain Res (1996) 73:135–9. doi:10.1016/0166-4328(96)00085-X
21. Challet E. Minireview: entrainment of the suprachiasmatic clockwork in diurnal and nocturnal mammals. Endocrinology (2007) 148:5648–55. doi:10.1210/en.2007-0804
22. Houben T, Deboer T, van Oosterhout F, Meijer JH. Correlation with behavioral activity and rest implies circadian regulation by SCN neuronal activity levels. J Biol Rhythms (2009) 24:477–87. doi:10.1177/0748730409349895
23. Houben T, Coomans CP, Meijer JH. Regulation of circadian and acute activity levels by the murine suprachiasmatic nuclei. PLoS One (2014) 9:e110172. doi:10.1371/journal.pone.0110172
24. Groos GA, Mason R. The visual properties of rat and cat suprachiasmatic neurones. J Comp Physiol (1980) 135:349–56. doi:10.1007/BF00657651
25. Meijer JH, Groos GA, Rusak B. Luminance coding in a circadian pacemaker: the suprachiasmatic nucleus of the rat and the hamster. Brain Res (1986) 382:109–18. doi:10.1016/0006-8993(86)90117-4
26. Kim YI, Dudek FE. Membrane properties of rat suprachiasmatic nucleus neurons receiving optic nerve input. J Physiol (1993) 464:229–43. doi:10.1113/jphysiol.1993.sp019632
27. Cui LN, Dyball RE. Synaptic input from the retina to the suprachiasmatic nucleus changes with the light-dark cycle in the Syrian hamster. J Physiol (1996) 497(Pt 2):483–93. doi:10.1113/jphysiol.1996.sp021782
28. Jiang ZG, Yang Y, Liu ZP, Allen CN. Membrane properties and synaptic inputs of suprachiasmatic nucleus neurons in rat brain slices. J Physiol (1997) 499(Pt 1):141–59. doi:10.1113/jphysiol.1997.sp021917
29. Aggelopoulos NC, Meissl H. Responses of neurones of the rat suprachiasmatic nucleus to retinal illumination under photopic and scotopic conditions. J Physiol (2000) 523(Pt 1):211–22. doi:10.1111/j.1469-7793.2000.t01-1-00211.x
30. Albrecht U, Sun ZS, Eichele G, Lee CC. A differential response of two putative mammalian circadian regulators, mper1 and mper2, to light. Cell (1997) 91:1055–64. doi:10.1016/S0092-8674(00)80495-X
31. Shearman LP, Zylka MJ, Weaver DR, Kolakowski LF Jr, Reppert SM. Two period homologs: circadian expression and photic regulation in the suprachiasmatic nuclei. Neuron (1997) 19:1261–9. doi:10.1016/S0896-6273(00)80417-1
32. Takumi T, Matsubara C, Shigeyoshi Y, Taguchi K, Yagita K, Maebayashi Y, et al. A new mammalian period gene predominantly expressed in the suprachiasmatic nucleus. Genes Cells (1998) 3:167–76. doi:10.1046/j.1365-2443.1998.00178.x
33. Zylka MJ, Shearman LP, Weaver DR, Reppert SM. Three period homologs in mammals: differential light responses in the suprachiasmatic circadian clock and oscillating transcripts outside of brain. Neuron (1998) 20:1103–10. doi:10.1016/S0896-6273(00)80492-4
34. Moriya T, Horikawa K, Akiyama M, Shibata S. Correlative association between N-methyl-D-aspartate receptor-mediated expression of period genes in the suprachiasmatic nucleus and phase shifts in behavior with photic entrainment of clock in hamsters. Mol Pharmacol (2000) 58:1554–62. doi:10.1124/mol.58.6.1554
35. Shigeyoshi Y, Taguchi K, Yamamoto S, Takekida S, Yan L, Tei H, et al. Light-induced resetting of a mammalian circadian clock is associated with rapid induction of the mPer1 transcript. Cell (1997) 91:1043–53. doi:10.1016/S0092-8674(00)80494-8
36. Ebling FJ. The role of glutamate in the photic regulation of the suprachiasmatic nucleus. Prog Neurobiol (1996) 50:109–32. doi:10.1016/S0301-0082(96)00032-9
37. Ding JM, Chen D, Weber ET, Faiman LE, Rea MA, Gillette MU. Resetting the biological clock: mediation of nocturnal circadian shifts by glutamate and NO. Science (1994) 266:1713–7. doi:10.1126/science.7527589
38. Shibata S, Watanabe A, Hamada T, Ono M, Watanabe S. N-methyl-D-aspartate induces phase shifts in circadian rhythm of neuronal activity of rat SCN in vitro. Am J Physiol (1994) 267:R360–4.
39. Biello SM, Golombek DA, Harrington ME. Neuropeptide Y and glutamate block each other’s phase shifts in the suprachiasmatic nucleus in vitro. Neuroscience (1997) 77:1049–57. doi:10.1016/S0306-4522(96)00547-7
40. Pittendrigh CS. Circadian systems: entrainment. In: Aschoff J, editor. Biological Rhythms. New York, NY: Plenum Press (1981). p. 95–5622.
41. Nelson DE, Takahashi JS. Comparison of visual sensitivity for suppression of pineal melatonin and circadian phase-shifting in the golden hamster. Brain Res (1991) 554:272–7. doi:10.1016/0006-8993(91)90200-F
42. Slotten HA, Krekling S, Pevet P. Photic and nonphotic effects on the circadian activity rhythm in the diurnal rodent Arvicanthis ansorgei. Behav Brain Res (2005) 165:91–7. doi:10.1016/j.bbr.2005.06.046
43. Ding JM, Buchanan GF, Tischkau SA, Chen D, Kuriashkina L, Faiman LE, et al. A neuronal ryanodine receptor mediates light-induced phase delays of the circadian clock. Nature (1998) 394:381–4. doi:10.1038/28639
44. Gillette MU, Mitchell JW. Signaling in the suprachiasmatic nucleus: selectively responsive and integrative. Cell Tissue Res (2002) 309:99–107. doi:10.1007/s00441-002-0576-1
45. Antle MC, Smith VM, Sterniczuk R, Yamakawa GR, Rakai BD. Physiological responses of the circadian clock to acute light exposure at night. Rev Endocr Metab Disord (2009) 10:279–91. doi:10.1007/s11154-009-9116-6
46. Schwartz WJ, Tavakoli-Nezhad M, Lambert CM, Weaver DR, de la Iglesia HO. Distinct patterns of period gene expression in the suprachiasmatic nucleus underlie circadian clock photoentrainment by advances or delays. Proc Natl Acad Sci U S A (2011) 108:17219–24. doi:10.1073/pnas.1107848108
47. Albus H, Vansteensel MJ, Michel S, Block GD, Meijer JH. A GABAergic mechanism is necessary for coupling dissociable ventral and dorsal regional oscillators within the circadian clock. Curr Biol (2005) 15:886–93. doi:10.1016/j.cub.2005.03.051
48. Davidson AJ, Castanon-Cervantes O, Leise TL, Molyneux PC, Harrington ME. Visualizing jet lag in the mouse suprachiasmatic nucleus and peripheral circadian timing system. Eur J Neurosci (2009) 29:171–80. doi:10.1111/j.1460-9568.2008.06534.x
49. Nagano M, Adachi A, Nakahama K, Nakamura T, Tamada M, Meyer-Bernstein E, et al. An abrupt shift in the day/night cycle causes desynchrony in the mammalian circadian center. J Neurosci (2003) 23:6141–51.
50. Nakamura W, Yamazaki S, Takasu NN, Mishima K, Block GD. Differential response of period 1 expression within the suprachiasmatic nucleus. J Neurosci (2005) 25:5481–7. doi:10.1523/JNEUROSCI.0889-05.2005
51. Reddy AB, Field MD, Maywood ES, Hastings MH. Differential resynchronisation of circadian clock gene expression within the suprachiasmatic nuclei of mice subjected to experimental jet lag. J Neurosci (2002) 22:7326–30.
52. Yan L, Silver R. Resetting the brain clock: time course and localization of mPER1 and mPER2 protein expression in suprachiasmatic nuclei during phase shifts. Eur J Neurosci (2004) 19:1105–9. doi:10.1111/j.1460-9568.2004.03189.x
53. Rohling JH, VanderLeest HT, Michel S, Vansteensel MJ, Meijer JH. Phase resetting of the mammalian circadian clock relies on a rapid shift of a small population of pacemaker neurons. PLoS One (2011) 6:e25437. doi:10.1371/journal.pone.0025437
54. Schaap J, Albus H, VanderLeest HT, Eilers PH, Detari L, Meijer JH. Heterogeneity of rhythmic suprachiasmatic nucleus neurons: implications for circadian waveform and photoperiodic encoding. Proc Natl Acad Sci U S A (2003) 100:15994–9. doi:10.1073/pnas.2436298100
55. Card JP, Brecha N, Karten HJ, Moore RY. Immunocytochemical localization of vasoactive intestinal polypeptide-containing cells and processes in the suprachiasmatic nucleus of the rat: light and electron microscopic analysis. J Neurosci (1981) 1:1289–303.
56. van Esseveldt KE, Lehman MN, Boer GJ. The suprachiasmatic nucleus and the circadian time-keeping system revisited. Brain Res Brain Res Rev (2000) 33:34–77. doi:10.1016/S0165-0173(00)00025-4
57. Meijer JH, Watanabe K, Schaap J, Albus H, Detari L. Light responsiveness of the suprachiasmatic nucleus: long-term multiunit and single-unit recordings in freely moving rats. J Neurosci (1998) 18:9078–87.
58. Shibata S, Oomura Y, Kita H, Liou SY, Ueki S. Field potentials in the suprachiasmatic nucleus of rat hypothalamic slice produced by optic nerve stimulation. Brain Res Bull (1984) 12:377–9. doi:10.1016/0361-9230(84)90108-4
59. Dardente H, Poirel VJ, Klosen P, Pevet P, Masson-Pevet M. Per and neuropeptide expression in the rat suprachiasmatic nuclei: compartmentalization and differential cellular induction by light. Brain Res (2002) 958:261–71. doi:10.1016/S0006-8993(02)03563-1
60. Guido ME, de GL, Goguen D, Robertson HA, Rusak B. Differential effects of glutamatergic blockade on circadian and photic regulation of gene expression in the hamster suprachiasmatic nucleus. Brain Res Mol Brain Res (1999) 67:247–57. doi:10.1016/S0169-328X(99)00074-1
61. Karatsoreos IN, Yan L, LeSauter J, Silver R. Phenotype matters: identification of light-responsive cells in the mouse suprachiasmatic nucleus. J Neurosci (2004) 24:68–75. doi:10.1523/JNEUROSCI.1666-03.2004
62. Kuhlman SJ, Silver R, Le Sauter J, Bult-Ito A, McMahon DG. Phase resetting light pulses induce Per1 and persistent spike activity in a subpopulation of biological clock neurons. J Neurosci (2003) 23:1441–50.
63. Schwartz WJ, Carpino A Jr, de la Iglesia HO, Baler R, Klein DC, Nakabeppu Y, et al. Differential regulation of fos family genes in the ventrolateral and dorsomedial subdivisions of the rat suprachiasmatic nucleus. Neuroscience (2000) 98:535–47. doi:10.1016/S0306-4522(00)00140-8
64. Yan L, Takekida S, Shigeyoshi Y, Okamura H. Per1 and Per2 gene expression in the rat suprachiasmatic nucleus: circadian profile and the compartment-specific response to light. Neuroscience (1999) 94:141–50. doi:10.1016/S0306-4522(99)00223-7
65. VanderLeest HT, Houben T, Michel S, Deboer T, Albus H, Vansteensel MJ, et al. Seasonal encoding by the circadian pacemaker of the SCN. Curr Biol (2007) 17:468–73. doi:10.1016/j.cub.2007.01.048
66. Mrugala M, Zlomanczuk P, Jagota A, Schwartz WJ. Rhythmic multiunit neural activity in slices of hamster suprachiasmatic nucleus reflect prior photoperiod. Am J Physiol Regul Integr Comp Physiol (2000) 278:R987–94.
67. Rohling J, Wolters L, Meijer JH. Simulation of day-length encoding in the SCN: from single-cell to tissue-level organization. J Biol Rhythms (2006) 21:301–13. doi:10.1177/0748730406290317
68. Rohling J, Meijer JH, VanderLeest HT, Admiraal J. Phase differences between SCN neurons and their role in photoperiodic encoding; a simulation of ensemble patterns using recorded single unit electrical activity patterns. J Physiol Paris (2006) 100:261–70. doi:10.1016/j.jphysparis.2007.05.005
69. Brown TM, Piggins HD. Spatiotemporal heterogeneity in the electrical activity of suprachiasmatic nuclei neurons and their response to photoperiod. J Biol Rhythms (2009) 24:44–54. doi:10.1177/0748730408327918
70. Naito E, Watanabe T, Tei H, Yoshimura T, Ebihara S. Reorganization of the suprachiasmatic nucleus coding for day length. J Biol Rhythms (2008) 23:140–9. doi:10.1177/0748730408314572
71. Pittendrigh CS, Kyner WT, Takamura T. The amplitude of circadian oscillations: temperature dependence, latitudinal clines, and the photoperiodic time measurement. J Biol Rhythms (1991) 6:299–313. doi:10.1177/074873049100600402
72. Evans JA, Elliott JA, Gorman MR. Photoperiod differentially modulates photic and nonphotic phase response curves of hamsters. Am J Physiol Regul Integr Comp Physiol (2004) 286:R539–46. doi:10.1152/ajpregu.00456.2003
73. Refinetti R. Compression and expansion of circadian rhythm in mice under long and short photoperiods. Integr Physiol Behav Sci (2002) 37:114–27. doi:10.1007/BF02688824
74. VanderLeest HT, Rohling JH, Michel S, Meijer JH. Phase shifting capacity of the circadian pacemaker determined by the SCN neuronal network organization. PLoS One (2009) 4:e4976.
75. Ramkisoensing A, Gu C, van Engeldorp Gastelaars HM, Michel S, Deboer T, Rohling JH, et al. Enhanced phase resetting in the synchronized suprachiasmatic nucleus network. J Biol Rhythms (2014) 29:4–15. doi:10.1177/0748730413516750
76. Arnol’d VI, Levi M. Geometrical Methods in the Theory of Ordinary Differential Equations. New York, NY: Springer-Verlag (1988).
78. Johnson CH, Kondo T. Light pulses induce “singular” behavior and shorten the period of the circadian phototaxis rhythm in the CW15 strain of Chlamydomonas. J Biol Rhythms (1992) 7:313–27. doi:10.1177/074873049200700405
79. Leloup JC, Gonze D, Goldbeter A. Limit cycle models for circadian rhythms based on transcriptional regulation in Drosophila and Neurospora. J Biol Rhythms (1999) 14:433–48. doi:10.1177/074873099129000948
80. Lakin-Thomas PL, Cote GG, Brody S. Circadian rhythms in Neurospora crassa: biochemistry and genetics. Crit Rev Microbiol (1990) 17:365–416. doi:10.3109/10408419009114762
81. Meijer JH, Colwell CS, Rohling JH, Houben T, Michel S. Dynamic neuronal network organization of the circadian clock and possible deterioration in disease. Prog Brain Res (2012) 199:143–62. doi:10.1016/B978-0-444-59427-3.00009-5
82. Guilding C, Scott F, Bechtold DA, Brown TM, Wegner S, Piggins HD. Suppressed cellular oscillations in after-hours mutant mice are associated with enhanced circadian phase-resetting. J Physiol (2013) 541:1663–80. doi:10.1113/jphysiol.2012.242198
83. Gu C, Ramkisoensing A, Liu Z, Meijer JH, Rohling JH. The proportion of light-responsive neurons determines the limit cycle properties of the suprachiasmatic nucleus. J Biol Rhythms (2014) 29:16–27. doi:10.1177/0748730413516752
84. Kaila K. Ionic basis of GABAA receptor channel function in the nervous system. Prog Neurobiol (1994) 42:489–537. doi:10.1016/0301-0082(94)90049-3
85. Van VC, Abbott LF, Ermentrout GB. When inhibition not excitation synchronizes neural firing. J Comput Neurosci (1994) 1:313–21. doi:10.1007/BF00961879
86. Borgers C, Krupa M, Gielen S. The response of a classical Hodgkin-Huxley neuron to an inhibitory input pulse. J Comput Neurosci (2010) 28:509–26. doi:10.1007/s10827-010-0233-8
87. Liu C, Reppert SM. GABA synchronizes clock cells within the suprachiasmatic circadian clock. Neuron (2000) 25:123–8. doi:10.1016/S0896-6273(00)80876-4
88. Evans JA, Leise TL, Castanon-Cervantes O, Davidson AJ. Dynamic interactions mediated by nonredundant signaling mechanisms couple circadian clock neurons. Neuron (2013) 80:973–83. doi:10.1016/j.neuron.2013.08.022
89. Belenky MA, Sollars PJ, Mount DB, Alper SL, Yarom Y, Pickard GE. Cell-type specific distribution of chloride transporters in the rat suprachiasmatic nucleus. Neuroscience (2010) 165:1519–37. doi:10.1016/j.neuroscience.2009.11.040
90. Choi HJ, Lee CJ, Schroeder A, Kim YS, Jung SH, Kim JS, et al. Excitatory actions of GABA in the suprachiasmatic nucleus. J Neurosci (2008) 28:5450–9. doi:10.1523/JNEUROSCI.5750-07.2008
91. Irwin RP, Allen CN. GABAergic signaling induces divergent neuronal Ca2+ responses in the suprachiasmatic nucleus network. Eur J Neurosci (2009) 30:1462–75. doi:10.1111/j.1460-9568.2009.06944.x
92. Farajnia S, van Westering TL, Meijer JH, Michel S. Seasonal induction of GABAergic excitation in the central mammalian clock. Proc Natl Acad Sci U S A (2014) 111:9627–32. doi:10.1073/pnas.1319820111
93. Gooley JJ, Lu J, Chou TC, Scammell TE, Saper CB. Melanopsin in cells of origin of the retinohypothalamic tract. Nat Neurosci (2001) 4:1165. doi:10.1038/nn768
94. Hannibal J, Fahrenkrug J. Melanopsin: a novel photopigment involved in the photoentrainment of the brain’s biological clock? Ann Med (2002) 34:401–7. doi:10.1080/078538902320772151
95. Hattar S, Liao HW, Takao M, Berson DM, Yau KW. Melanopsin-containing retinal ganglion cells: architecture, projections, and intrinsic photosensitivity. Science (2002) 295:1065–70. doi:10.1126/science.1069609
96. Abrahamson EE, Leak RK, Moore RY. The suprachiasmatic nucleus projects to posterior hypothalamic arousal systems. Neuroreport (2001) 12:435–40. doi:10.1097/00001756-200102120-00048
97. Moga MM, Moore RY. Putative excitatory amino acid projections to the suprachiasmatic nucleus in the rat. Brain Res (1996) 743:171–7. doi:10.1016/S0006-8993(96)01051-7
98. Gompf HS, Fuller PM, Hattar S, Saper CB, Lu J. Impaired circadian photosensitivity in mice lacking glutamate transmission from retinal melanopsin cells. J Biol Rhythms (2015) 30:35–41. doi:10.1177/0748730414561545
99. Dragich JM, Loh DH, Wang LM, Vosko AM, Kudo T, Nakamura TJ, et al. The role of the neuropeptides PACAP and VIP in the photic regulation of gene expression in the suprachiasmatic nucleus. Eur J Neurosci (2010) 31:864–75. doi:10.1111/j.1460-9568.2010.07119.x
100. Hannibal J, Brabet P, Fahrenkrug J. Mice lacking the PACAP type I receptor have impaired photic entrainment and negative masking. Am J Physiol Regul Integr Comp Physiol (2008) 295:R2050–8. doi:10.1152/ajpregu.90563.2008
101. Brown TM, Colwell CS, Waschek JA, Piggins HD. Disrupted neuronal activity rhythms in the suprachiasmatic nuclei of vasoactive intestinal polypeptide-deficient mice. J Neurophysiol (2007) 97:2553–8. doi:10.1152/jn.01206.2006
102. Maywood ES, Reddy AB, Wong GK, O’Neill JS, O’Brien JA, McMahon DG, et al. Synchronization and maintenance of timekeeping in suprachiasmatic circadian clock cells by neuropeptidergic signaling. Curr Biol (2006) 16:599–605. doi:10.1016/j.cub.2006.02.023
103. Maywood ES, Chesham JE, O’Brien JA, Hastings MH. A diversity of paracrine signals sustains molecular circadian cycling in suprachiasmatic nucleus circuits. Proc Natl Acad Sci U S A (2011) 108:14306–11. doi:10.1073/pnas.1101767108
104. Aton SJ, Colwell CS, Harmar AJ, Waschek J, Herzog ED. Vasoactive intestinal polypeptide mediates circadian rhythmicity and synchrony in mammalian clock neurons. Nat Neurosci (2005) 8:476–83. doi:10.1038/nn1419
105. Colwell CS, Michel S, Itri J, Rodriguez W, Tam J, Lelievre V, et al. Disrupted circadian rhythms in VIP- and PHI-deficient mice. Am J Physiol Regul Integr Comp Physiol (2003) 285:R939–49. doi:10.1152/ajpregu.00200.2003
106. Harmar AJ, Marston HM, Shen S, Spratt C, West KM, Sheward WJ, et al. The VPAC(2) receptor is essential for circadian function in the mouse suprachiasmatic nuclei. Cell (2002) 109:497–508. doi:10.1016/S0092-8674(02)00736-5
107. Ciarleglio CM, Gamble KL, Axley JC, Strauss BR, Cohen JY, Colwell CS, et al. Population encoding by circadian clock neurons organizes circadian behavior. J Neurosci (2009) 29:1670–6. doi:10.1523/JNEUROSCI.3801-08.2009
108. Reed HE, Meyer-Spasche A, Cutler DJ, Coen CW, Piggins HD. Vasoactive intestinal polypeptide (VIP) phase-shifts the rat suprachiasmatic nucleus clock in vitro. Eur J Neurosci (2001) 13:839–43. doi:10.1046/j.0953-816x.2000.01437.x
109. Piggins HD, Antle MC, Rusak B. Neuropeptides phase shift the mammalian circadian pacemaker. J Neurosci (1995) 15:5612–22.
110. Kudo T, Tahara Y, Gamble KL, McMahon DG, Block GD, Colwell CS. Vasoactive intestinal peptide produces long-lasting changes in neural activity in the suprachiasmatic nucleus. J Neurophysiol (2013) 110:1097–106. doi:10.1152/jn.00114.2013
111. Lucassen EA, van Diepen HC, Houben T, Michel S, Colwell CS, Meijer JH. Role of vasoactive intestinal peptide in seasonal encoding by the suprachiasmatic nucleus clock. Eur J Neurosci (2012) 35:1466–74. doi:10.1111/j.1460-9568.2012.08054.x
112. Aida R, Moriya T, Araki M, Akiyama M, Wada K, Wada E, et al. Gastrin-releasing peptide mediates photic entrainable signals to dorsal subsets of suprachiasmatic nucleus via induction of period gene in mice. Mol Pharmacol (2002) 61:26–34. doi:10.1124/mol.61.1.26
113. McArthur AJ, Coogan AN, Ajpru S, Sugden D, Biello SM, Piggins HD. Gastrin-releasing peptide phase-shifts suprachiasmatic nuclei neuronal rhythms in vitro. J Neurosci (2000) 20:5496–502.
114. Kallingal GJ, Mintz EM. Site-specific effects of gastrin-releasing peptide in the suprachiasmatic nucleus. Eur J Neurosci (2014) 39:630–9. doi:10.1111/ejn.12411
115. Jac M, Kiss A, Sumova A, Illnerova H, Jezova D. Daily profiles of arginine vasopressin mRNA in the suprachiasmatic, supraoptic and paraventricular nuclei of the rat hypothalamus under various photoperiods. Brain Res (2000) 887:472–6. doi:10.1016/S0006-8993(00)03050-X
116. Tournier BB, Dardente H, Simonneaux V, Vivien-Roels B, Pevet P, Masson-Pevet M, et al. Seasonal variations of clock gene expression in the suprachiasmatic nuclei and pars tuberalis of the European hamster (Cricetus cricetus). Eur J Neurosci (2007) 25:1529–36. doi:10.1111/j.1460-9568.2007.05421.x
117. Freeman GM Jr, Krock RM, Aton SJ, Thaben P, Herzog ED. GABA networks destabilize genetic oscillations in the circadian pacemaker. Neuron (2013) 78:799–806. doi:10.1016/j.neuron.2013.04.003
118. Girardet C, Blanchard MP, Ferracci G, Leveque C, Moreno M, Francois-Bellan AM, et al. Daily changes in synaptic innervation of VIP neurons in the rat suprachiasmatic nucleus: contribution of glutamatergic afferents. Eur J Neurosci (2010) 31:359–70. doi:10.1111/j.1460-9568.2009.07071.x
119. Becquet D, Girardet C, Guillaumond F, Francois-Bellan AM, Bosler O. Ultrastructural plasticity in the rat suprachiasmatic nucleus. Possible involvement in clock entrainment. Glia (2008) 56:294–305. doi:10.1002/glia.20613
120. Colwell CS. Rhythmic coupling among cells in the suprachiasmatic nucleus. J Neurobiol (2000) 43:379–88. doi:10.1002/1097-4695(20000615)43:4<379::AID-NEU6>3.0.CO;2-0
121. Long MA, Jutras MJ, Connors BW, Burwell RD. Electrical synapses coordinate activity in the suprachiasmatic nucleus. Nat Neurosci (2005) 8:61–6. doi:10.1038/nn1361
122. Rash JE, Olson CO, Pouliot WA, Davidson KG, Yasumura T, Furman CS, et al. Connexin36 vs. connexin32, “miniature” neuronal gap junctions, and limited electrotonic coupling in rodent suprachiasmatic nucleus. Neuroscience (2007) 149:350–71. doi:10.1016/j.neuroscience.2007.06.052
123. Wang MH, Chen N, Wang JH. The coupling features of electrical synapses modulate neuronal synchrony in hypothalamic suprachiasmatic nucleus. Brain Res (2014) 1550:9–17. doi:10.1016/j.brainres.2014.01.007
124. Deans MR, Gibson JR, Sellitto C, Connors BW, Paul DL. Synchronous activity of inhibitory networks in neocortex requires electrical synapses containing connexin36. Neuron (2001) 31:477–85. doi:10.1016/S0896-6273(01)00373-7
125. Pfeuty B, Mato G, Golomb D, Hansel D. Electrical synapses and synchrony: the role of intrinsic currents. J Neurosci (2003) 23:6280–94.
126. Fortier PA. Effects of electrical coupling among layer 4 inhibitory interneurons on contrast-invariant orientation tuning. Exp Brain Res (2011) 208:127–38. doi:10.1007/s00221-010-2483-0
127. Galarreta M, Hestrin S. Electrical synapses between GABA-releasing interneurons. Nat Rev Neurosci (2001) 2:425–33. doi:10.1038/35077566
128. Hjorth J, Blackwell KT, Kotaleski JH. Gap junctions between striatal fast-spiking interneurons regulate spiking activity and synchronization as a function of cortical activity. J Neurosci (2009) 29:5276–86. doi:10.1523/JNEUROSCI.6031-08.2009
129. Hoge GJ, Davidson KG, Yasumura T, Castillo PE, Rash JE, Pereda AE. The extent and strength of electrical coupling between inferior olivary neurons is heterogeneous. J Neurophysiol (2011) 105:1089–101. doi:10.1152/jn.00789.2010
130. Vandecasteele M, Glowinski J, Venance L. Electrical synapses between dopaminergic neurons of the substantia nigra pars compacta. J Neurosci (2005) 25:291–8. doi:10.1523/JNEUROSCI.4167-04.2005
131. Connors BW, Long MA. Electrical synapses in the mammalian brain. Annu Rev Neurosci (2004) 27:393–418. doi:10.1146/annurev.neuro.26.041002.131128
132. Bouskila Y, Dudek FE. Neuronal synchronization without calcium-dependent synaptic transmission in the hypothalamus. Proc Natl Acad Sci U S A (1993) 90:3207–10. doi:10.1073/pnas.90.8.3207
133. Dijk DJ, Duffy JF. Circadian regulation of human sleep and age-related changes in its timing, consolidation and EEG characteristics. Ann Med (1999) 31:130–40. doi:10.3109/07853899908998789
134. Van Someren EJ. Circadian and sleep disturbances in the elderly. Exp Gerontol (2000) 35:1229–37. doi:10.1016/S0531-5565(00)00191-1
135. Van Someren EJ. Circadian rhythms and sleep in human aging. Chronobiol Int (2000) 17:233–43. doi:10.1081/CBI-100101046
136. Farajnia S, Michel S, Deboer T, VanderLeest HT, Houben T, Rohling JH, et al. Evidence for neuronal desynchrony in the aged suprachiasmatic nucleus clock. J Neurosci (2012) 32:5891–9. doi:10.1523/JNEUROSCI.0469-12.2012
137. Van RO, Zhang Y, Zee PC, Turek FW. Grafting fetal suprachiasmatic nuclei in the hypothalamus of old hamsters restores responsiveness of the circadian clock to a phase shifting stimulus. Brain Res (1994) 643:338–42. doi:10.1016/0006-8993(94)90044-2
138. Hurd MW, Zimmer KA, Lehman MN, Ralph MR. Circadian locomotor rhythms in aged hamsters following suprachiasmatic transplant. Am J Physiol (1995) 269:R958–68.
139. Cai A, Lehman MN, Lloyd JM, Wise PM. Transplantation of fetal suprachiasmatic nuclei into middle-aged rats restores diurnal Fos expression in host. Am J Physiol (1997) 272:R422–8.
140. Nakamura TJ, Nakamura W, Yamazaki S, Kudo T, Cutler T, Colwell CS, et al. Age-related decline in circadian output. J Neurosci (2011) 31:10201–5. doi:10.1523/JNEUROSCI.0451-11.2011
141. Miller MM, Gould BE, Nelson JF. Aging and long-term ovariectomy alter the cytoarchitecture of the hypothalamic-preoptic area of the C57BL/6J mouse. Neurobiol Aging (1989) 10:683–90. doi:10.1016/0197-4580(89)90005-5
142. Roozendaal B, van Gool WA, Swaab DF, Hoogendijk JE, Mirmiran M. Changes in vasopressin cells of the rat suprachiasmatic nucleus with aging. Brain Res (1987) 409:259–64. doi:10.1016/0006-8993(87)90710-4
143. Nygard M, Hill RH, Wikstrom MA, Kristensson K. Age-related changes in electrophysiological properties of the mouse suprachiasmatic nucleus in vitro. Brain Res Bull (2005) 65:149–54. doi:10.1016/j.brainresbull.2004.12.006
144. Aujard F, Herzog ED, Block GD. Circadian rhythms in firing rate of individual suprachiasmatic nucleus neurons from adult and middle-aged mice. Neuroscience (2001) 106:255–61. doi:10.1016/S0306-4522(01)00285-8
145. Biello SM. Circadian clock resetting in the mouse changes with age. Age (2009) 31:293–303. doi:10.1007/s11357-009-9102-7
146. Satinoff E, Li H, Tcheng TK, Liu C, McArthur AJ, Medanic M, et al. Do the suprachiasmatic nuclei oscillate in old rats as they do in young ones? Am J Physiol (1993) 265:R1216–22.
147. Watanabe A, Shibata S, Watanabe S. Circadian rhythm of spontaneous neuronal activity in the suprachiasmatic nucleus of old hamster in vitro. Brain Res (1995) 695:237–9. doi:10.1016/0006-8993(95)00713-Z
148. Farajnia S, Deboer T, Rohling JH, Meijer JH, Michel S. Aging of the suprachiasmatic clock. Neuroscientist (2014) 20:44–55. doi:10.1177/1073858413498936
149. Chee CA, Roozendaal B, Swaab DF, Goudsmit E, Mirmiran M. Vasoactive intestinal polypeptide neuron changes in the senile rat suprachiasmatic nucleus. Neurobiol Aging (1988) 9:307–12. doi:10.1016/S0197-4580(88)80070-8
150. Kawakami F, Okamura H, Tamada Y, Maebayashi Y, Fukui K, Ibata Y. Loss of day-night differences in VIP mRNA levels in the suprachiasmatic nucleus of aged rats. Neurosci Lett (1997) 222:99–102. doi:10.1016/S0304-3940(97)13355-9
151. Kallo I, Kalamatianos T, Piggins HD, Coen CW. Ageing and the diurnal expression of mRNAs for vasoactive intestinal peptide and for the VPAC2 and PAC1 receptors in the suprachiasmatic nucleus of male rats. J Neuroendocrinol (2004) 16:758–66. doi:10.1111/j.1365-2826.2004.01232.x
152. Palomba M, Nygard M, Florenzano F, Bertini G, Kristensson K, Bentivoglio M. Decline of the presynaptic network, including GABAergic terminals, in the aging suprachiasmatic nucleus of the mouse. J Biol Rhythms (2008) 23:220–31. doi:10.1177/0748730408316998
153. Zhou JN, Hofman MA, Swaab DF. VIP neurons in the human SCN in relation to sex, age, and Alzheimer’s disease. Neurobiol Aging (1995) 16:571–6. doi:10.1016/0197-4580(95)00043-E
154. Swaab DF, Fliers E, Partiman TS. The suprachiasmatic nucleus of the human brain in relation to sex, age and senile dementia. Brain Res (1985) 342:37–44. doi:10.1016/0006-8993(85)91350-2
155. Weinert H, Weinert D, Schurov I, Maywood ES, Hastings MH. Impaired expression of the mPer2 circadian clock gene in the suprachiasmatic nuclei of aging mice. Chronobiol Int (2001) 18:559–65. doi:10.1081/CBI-100103976
156. Kolker DE, Fukuyama H, Huang DS, Takahashi JS, Horton TH, Turek FW. Aging alters circadian and light-induced expression of clock genes in golden hamsters. J Biol Rhythms (2003) 18:159–69. doi:10.1177/0748730403251802
157. Wyse CA, Coogan AN. Impact of aging on diurnal expression patterns of CLOCK and BMAL1 in the mouse brain. Brain Res (2010) 1337:21–31. doi:10.1016/j.brainres.2010.03.113
158. Chang HC, Guarente L. SIRT1 mediates central circadian control in the SCN by a mechanism that decays with aging. Cell (2013) 153:1448–60. doi:10.1016/j.cell.2013.05.027
159. Asai M, Yoshinobu Y, Kaneko S, Mori A, Nikaido T, Moriya T, et al. Circadian profile of Per gene mRNA expression in the suprachiasmatic nucleus, paraventricular nucleus, and pineal body of aged rats. J Neurosci Res (2001) 66:1133–9. doi:10.1002/jnr.10010
160. Sellix MT, Evans JA, Leise TL, Castanon-Cervantes O, Hill DD, DeLisser P, et al. Aging differentially affects the re-entrainment response of central and peripheral circadian oscillators. J Neurosci (2012) 32:16193–202. doi:10.1523/JNEUROSCI.3559-12.2012
161. Borbely AA, Achermann P. Sleep homeostasis and models of sleep regulation. J Biol Rhythms (1999) 14:557–68.
162. Tobler I, Borbely AA. Sleep EEG in the rat as a function of prior waking. Electroencephalogr Clin Neurophysiol (1986) 64:74–6. doi:10.1016/0013-4694(86)90044-1
163. Dijk DJ, Beersma DG, Daan S, Bloem GM, Van den Hoofdakker RH. Quantitative analysis of the effects of slow wave sleep deprivation during the first 3 h of sleep on subsequent EEG power density. Eur Arch Psychiatry Neurol Sci (1987) 236:323–8. doi:10.1007/BF00377420
164. Lancel M, van RH, Glatt A. Effects of circadian phase and duration of sleep deprivation on sleep and EEG power spectra in the cat. Brain Res (1991) 548:206–14. doi:10.1016/0006-8993(91)91123-I
165. Strijkstra AM, Daan S. Dissimilarity of slow-wave activity enhancement by torpor and sleep deprivation in a hibernator. Am J Physiol (1998) 275:R1110–7.
166. Huber R, Deboer T, Tobler I. Topography of EEG dynamics after sleep deprivation in mice. J Neurophysiol (2000) 84:1888–93.
167. Deboer T, Tobler I. Sleep regulation in the Djungarian hamster: comparison of the dynamics leading to the slow-wave activity increase after sleep deprivation and daily torpor. Sleep (2003) 26:567–72.
168. Rattenborg NC, Martinez-Gonzalez D, Lesku JA. Convergent evolution of complex brains, cognition and sleep functions in mammals and birds. Neurosci Biol (2009) 33:253–70. doi:10.1016/j.neubiorev.2008.08.010
169. Deboer T, Vansteensel MJ, Detari L, Meijer JH. Sleep states alter activity of suprachiasmatic nucleus neurons. Nat Neurosci (2003) 6:1086–90. doi:10.1038/nn1122
170. Deboer T, Detari L, Meijer JH. Long term effects of sleep deprivation on the mammalian circadian pacemaker. Sleep (2007) 30:257–62.
171. Cermakian N, Lamont EW, Boudreau P, Boivin DB. Circadian clock gene expression in brain regions of Alzheimer’s disease patients and control subjects. J Biol Rhythms (2011) 26:160–70. doi:10.1177/0748730410395732
172. Harper DG, Stopa EG, Kuo-Leblanc V, McKee AC, Asayama K, Volicer L, et al. Dorsomedial SCN neuronal subpopulations subserve different functions in human dementia. Brain (2008) 131:1609–17. doi:10.1093/brain/awn049
173. Sterniczuk R, Antle MC, Laferla FM, Dyck RH. Characterization of the 3xTg-AD mouse model of Alzheimer’s disease: part 2. Behavioral and cognitive changes. Brain Res (2010) 1348:149–55. doi:10.1016/j.brainres.2010.06.011
174. Sterniczuk R, Dyck RH, Laferla FM, Antle MC. Characterization of the 3xTg-AD mouse model of Alzheimer’s disease: part 1. Circadian changes. Brain Res (2010) 1348:139–48. doi:10.1016/j.brainres.2010.05.013
175. Duncan MJ, Smith JT, Franklin KM, Beckett TL, Murphy MP, St Clair DK, et al. Effects of aging and genotype on circadian rhythms, sleep, and clock gene expression in APPxPS1 knock-in mice, a model for Alzheimer’s disease. Exp Neurol (2012) 236:249–58. doi:10.1016/j.expneurol.2012.05.011
176. Morton AJ, Wood NI, Hastings MH, Hurelbrink C, Barker RA, Maywood ES. Disintegration of the sleep-wake cycle and circadian timing in Huntington’s disease. J Neurosci (2005) 25:157–63. doi:10.1523/JNEUROSCI.3842-04.2005
177. Pallier PN, Maywood ES, Zheng Z, Chesham JE, Inyushkin AN, Dyball R, et al. Pharmacological imposition of sleep slows cognitive decline and reverses dysregulation of circadian gene expression in a transgenic mouse model of Huntington’s disease. J Neurosci (2007) 27:7869–78. doi:10.1523/JNEUROSCI.0649-07.2007
178. Kudo T, Schroeder A, Loh DH, Kuljis D, Jordan MC, Roos KP, et al. Dysfunctions in circadian behavior and physiology in mouse models of Huntington’s disease. Exp Neurol (2011) 228:80–90. doi:10.1016/j.expneurol.2010.12.011
179. Kudo T, Loh DH, Truong D, Wu Y, Colwell CS. Circadian dysfunction in a mouse model of Parkinson’s disease. Exp Neurol (2011) 232:66–75. doi:10.1016/j.expneurol.2011.08.003
180. Verkerk AJ, Pieretti M, Sutcliffe JS, Fu YH, Kuhl DP, Pizzuti A, et al. Identification of a gene (FMR-1) containing a CGG repeat coincident with a breakpoint cluster region exhibiting length variation in fragile X syndrome. Cell (1991) 65:905–14. doi:10.1016/0092-8674(91)90397-H
181. Hagerman RJ, Staley LW, O’Conner R, Lugenbeel K, Nelson D, McLean SD, et al. Learning-disabled males with a fragile X CGG expansion in the upper premutation size range. Pediatrics (1996) 97:122–6.
182. Gould EL, Loesch DZ, Martin MJ, Hagerman RJ, Armstrong SM, Huggins RM. Melatonin profiles and sleep characteristics in boys with fragile X syndrome: a preliminary study. Am J Med Genet (2000) 95:307–15. doi:10.1002/1096-8628(20001211)95:4<307::AID-AJMG3>3.0.CO;2-3
183. Zhang J, Fang Z, Jud C, Vansteensel MJ, Kaasik K, Lee CC, et al. Fragile X-related proteins regulate mammalian circadian behavioral rhythms. Am J Hum Genet (2008) 83:43–52. doi:10.1016/j.ajhg.2008.06.003
184. Lewy AJ, Wehr TA, Goodwin FK, Newsome DA, Markey SP. Light suppresses melatonin secretion in humans. Science (1980) 210:1267–9. doi:10.1126/science.7434030
185. Jung CM, Khalsa SB, Scheer FA, Cajochen C, Lockley SW, Czeisler CA, et al. Acute effects of bright light exposure on cortisol levels. J Biol Rhythms (2010) 25:208–16. doi:10.1177/0748730410368413
186. Chang AM, Santhi N, St Hilaire M, Gronfier C, Bradstreet DS, Duffy JF, et al. Human responses to bright light of different durations. J Physiol (2012) 590:3103–12. doi:10.1113/jphysiol.2011.226555
187. Chellappa SL, Steiner R, Oelhafen P, Lang D, Gotz T, Krebs J, et al. Acute exposure to evening blue-enriched light impacts on human sleep. J Sleep Res (2013) 22:573–80. doi:10.1111/jsr.12050
188. Gallant AR, Lundgren J, Allison K, Stunkard AJ, Lambert M, O’Loughlin J, et al. Validity of the night eating questionnaire in children. Int J Eat Disord (2012) 45:861–5. doi:10.1002/eat.22021
189. Knutsson A. Health disorders of shift workers. Occup Med (2003) 53:103–8. doi:10.1093/occmed/kqg048
190. Haus EL, Smolensky MH. Shift work and cancer risk: potential mechanistic roles of circadian disruption, light at night, and sleep deprivation. Sleep Med Rev (2013) 17:273–84. doi:10.1016/j.smrv.2012.08.003
191. Ruger M, Scheer FA. Effects of circadian disruption on the cardiometabolic system. Rev Endocr Metab Disord (2009) 10:245–60. doi:10.1007/s11154-009-9122-8
192. Reid KJ, Zee PC. Circadian rhythm disorders. Semin Neurol (2009) 29:393–405. doi:10.1055/s-0029-1237120
193. Wulff K, Porcheret K, Cussans E, Foster RG. Sleep and circadian rhythm disturbances: multiple genes and multiple phenotypes. Curr Opin Genet Dev (2009) 19:237–46. doi:10.1016/j.gde.2009.03.007
194. Bray MS, Shaw CA, Moore MW, Garcia RA, Zanquetta MM, Durgan DJ, et al. Disruption of the circadian clock within the cardiomyocyte influences myocardial contractile function, metabolism, and gene expression. Am J Physiol Heart Circ Physiol (2008) 294:H1036–47. doi:10.1152/ajpheart.01291.2007
195. Scheer FA, Hilton MF, Mantzoros CS, Shea SA. Adverse metabolic and cardiovascular consequences of circadian misalignment. Proc Natl Acad Sci U S A (2009) 106:4453–8. doi:10.1073/pnas.0808180106
196. Oishi K, Ohkura N. Chronic circadian clock disruption induces expression of the cardiovascular risk factor plasminogen activator inhibitor-1 in mice. Blood Coagul Fibrinolysis (2013) 24:106–8. doi:10.1097/MBC.0b013e32835bfdf3
197. Gerstner JR, Bremer QZ, Vander Heyden WM, Lavaute TM, Yin JC, Landry CF. Brain fatty acid binding protein (Fabp7) is diurnally regulated in astrocytes and hippocampal granule cell precursors in adult rodent brain. PLoS One (2008) 3:e1631. doi:10.1371/journal.pone.0001631
198. Marcheva B, Ramsey KM, Buhr ED, Kobayashi Y, Su H, Ko CH, et al. Disruption of the clock components CLOCK and BMAL1 leads to hypoinsulinemia and diabetes. Nature (2010) 466:627–31. doi:10.1038/nature09253
199. Turek FW, Joshu C, Kohsaka A, Lin E, Ivanova G, McDearmon E, et al. Obesity and metabolic syndrome in circadian clock mutant mice. Science (2005) 308:1043–5. doi:10.1126/science.1108750
200. Barclay JL, Husse J, Bode B, Naujokat N, Meyer-Kovac J, Schmid SM, et al. Circadian desynchrony promotes metabolic disruption in a mouse model of shiftwork. PLoS One (2012) 7:e37150. doi:10.1371/journal.pone.0037150
201. Phan TX, Chan GC, Sindreu CB, Eckel-Mahan KL, Storm DR. The diurnal oscillation of MAP (mitogen-activated protein) kinase and adenylyl cyclase activities in the hippocampus depends on the suprachiasmatic nucleus. J Neurosci (2011) 31:10640–7. doi:10.1523/JNEUROSCI.6535-10.2011
202. Angeles-Castellanos M, Salgado-Delgado R, Rodriguez K, Buijs RM, Escobar C. The suprachiasmatic nucleus participates in food entrainment: a lesion study. Neuroscience (2010) 165:1115–26. doi:10.1016/j.neuroscience.2009.11.061
203. Kalsbeek A, La FS, Van HC, Buijs RM. Suprachiasmatic GABAergic inputs to the paraventricular nucleus control plasma glucose concentrations in the rat via sympathetic innervation of the liver. J Neurosci (2004) 24:7604–13. doi:10.1523/JNEUROSCI.5328-03.2004
204. Kalsbeek A, Foppen E, Schalij I, Van Heijningen C, van der Vliet J, Fliers E, et al. Circadian control of the daily plasma glucose rhythm: an interplay of GABA and glutamate. PLoS One (2008) 3:e3194. doi:10.1371/journal.pone.0003194
205. Kreier F, Kap YS, Mettenleiter TC, Van HC, van Heijningen C, van der Vliet J, et al. Tracing from fat tissue, liver, and pancreas: a neuroanatomical framework for the role of the brain in type 2 diabetes. Endocrinology (2006) 147:1140–7. doi:10.1210/en.2005-0667
206. Kreier F, Fliers E, Voshol PJ, Van Eden CG, Havekes LM, Kalsbeek A, et al. Selective parasympathetic innervation of subcutaneous and intra-abdominal fat – functional implications. J Clin Invest (2002) 110:1243–50. doi:10.1172/JCI0215736
207. Kalsbeek A, Yi CX, Cailotto C, La Fleur SE, Fliers E, Buijs RM. Mammalian clock output mechanisms. Essays Biochem (2011) 49:137–51. doi:10.1042/BSE0480137
208. Kalsbeek A, Fliers E, Romijn JA, La Fleur SE, Wortel J, Bakker O, et al. The suprachiasmatic nucleus generates the diurnal changes in plasma leptin levels. Endocrinology (2001) 142:2677–85. doi:10.1210/endo.142.6.8197
209. Coomans CP, van den Berg SA, Lucassen EA, Houben T, Pronk AC, van der Spek RD, et al. The suprachiasmatic nucleus controls circadian energy metabolism and hepatic insulin sensitivity. Diabetes (2013) 62:1102–8. doi:10.2337/db12-0507
210. Fonken LK, Aubrecht TG, Melendez-Fernandez OH, Weil ZM, Nelson RJ. Dim light at night disrupts molecular circadian rhythms and increases body weight. J Biol Rhythms (2013) 28:262–71. doi:10.1177/0748730413493862
211. Fonken LK, Lieberman RA, Weil ZM, Nelson RJ. Dim light at night exaggerates weight gain and inflammation associated with a high-fat diet in male mice. Endocrinology (2013) 154:3817–25. doi:10.1210/en.2013-1121
212. Coomans CP, van den Berg SA, Houben T, van Klinken JB, van den Berg R, Pronk AC, et al. Detrimental effects of constant light exposure and high-fat diet on circadian energy metabolism and insulin sensitivity. FASEB J (2013) 27:1721–32. doi:10.1096/fj.12-210898
213. Ohta H, Yamazaki S, McMahon DG. Constant light desynchronizes mammalian clock neurons. Nat Neurosci (2005) 8:267–9. doi:10.1038/nn1395
214. Ohta H, Mitchell AC, McMahon DG. Constant light disrupts the developing mouse biological clock. Pediatr Res (2006) 60:304–8. doi:10.1203/01.pdr.0000233114.18403.66
215. Mendoza J, Pevet P, Challet E. High-fat feeding alters the clock synchronization to light. J Physiol (2008) 586:5901–10. doi:10.1113/jphysiol.2008.159566
216. Grosbellet E, Gourmelen S, Pevet P, Criscuolo F, Challet E. Leptin normalizes photic synchronization in male ob/ob mice, via indirect effects on the suprachiasmatic nucleus. Endocrinology (2014) 156(3):1080–90. doi:10.1210/en.2014-1570
217. Mrosovsky N. Locomotor activity and non-photic influences on circadian clocks. Biol Rev Camb Philos Soc (1996) 71:343–72. doi:10.1111/j.1469-185X.1996.tb01278.x
218. Mrosovsky N. Phase response curves for social entrainment. J Comp Physiol A (1988) 162:35–46. doi:10.1007/BF01342701
219. Canal MM, Piggins HD. Resetting of the hamster circadian system by dark pulses. Am J Physiol Regul Integr Comp Physiol (2006) 290:R785–92. doi:10.1152/ajpregu.00548.2005
220. Antle MC, Mistlberger RE. Circadian clock resetting by sleep deprivation without exercise in the Syrian hamster. J Neurosci (2000) 20:9326–32.
221. Van RO, Turek FW. Stimulated activity mediates phase shifts in the hamster circadian clock induced by dark pulses or benzodiazepines. Nature (1989) 339:49–51. doi:10.1038/339049a0
222. Albers HE, Ferris CF. Neuropeptide Y: role in light-dark cycle entrainment of hamster circadian rhythms. Neurosci Lett (1984) 50:163–8. doi:10.1016/0304-3940(84)90480-4
223. Tominaga K, Shibata S, Ueki S, Watanabe S. Effects of 5-HT1A receptor agonists on the circadian rhythm of wheel-running activity in hamsters. Eur J Pharmacol (1992) 214:79–84. doi:10.1016/0014-2999(92)90099-P
224. Marchant EG, Mistlberger RE. Morphine phase-shifts circadian rhythms in mice: role of behavioural activation. Neuroreport (1995) 7:209–12. doi:10.1097/00001756-199512290-00050
225. Mrosovsky N, Salmon PA. Triazolam and phase-shifting acceleration re-evaluated. Chronobiol Int (1990) 7:35–41. doi:10.3109/07420529009056952
226. Wee BE, Turek FW. Midazolam, a short-acting benzodiazepine, resets the circadian clock of the hamster. Pharmacol Biochem Behav (1989) 32:901–6. doi:10.1016/0091-3057(89)90056-7
227. Challet E, Pevet P, Malan A. Intergeniculate leaflet lesion and daily rhythms in food-restricted rats fed during daytime. Neurosci Lett (1996) 216:214–8. doi:10.1016/0304-3940(96)13012-3
228. Cutrera RA, Kalsbeek A, Pevet P. Specific destruction of the serotonergic afferents to the suprachiasmatic nuclei prevents triazolam-induced phase advances of hamster activity rhythms. Behav Brain Res (1994) 62:21–8. doi:10.1016/0166-4328(94)90034-5
229. Edgar DM, Reid MS, Dement WC. Serotonergic afferents mediate activity-dependent entrainment of the mouse circadian clock. Am J Physiol (1997) 273:R265–9.
230. Marchant EG, Watson NV, Mistlberger RE. Both neuropeptide Y and serotonin are necessary for entrainment of circadian rhythms in mice by daily treadmill running schedules. J Neurosci (1997) 17:7974–87.
231. Dudley TE, DiNardo LA, Glass JD. Endogenous regulation of serotonin release in the hamster suprachiasmatic nucleus. J Neurosci (1998) 18:5045–52.
232. Grossman GH, Farnbauch L, Glass JD. Regulation of serotonin release in the Syrian hamster intergeniculate leaflet region. Neuroreport (2004) 15:103–6. doi:10.1097/00001756-200401190-00021
233. Sumova A, Maywood ES, Selvage D, Ebling FJ, Hastings MH. Serotonergic antagonists impair arousal-induced phase shifts of the circadian system of the Syrian hamster. Brain Res (1996) 709:88–96. doi:10.1016/0006-8993(95)01314-8
234. Morin LP, Blanchard J. Organization of the hamster intergeniculate leaflet: NPY and ENK projections to the suprachiasmatic nucleus, intergeniculate leaflet and posterior limitans nucleus. Vis Neurosci (1995) 12:57–67. doi:10.1017/S0952523800007318
235. Card JP, Moore RY. Organization of lateral geniculate-hypothalamic connections in the rat. J Comp Neurol (1989) 284:135–47. doi:10.1002/cne.902840110
236. Mantyh PW, Kemp JA. The distribution of putative neurotransmitters in the lateral geniculate nucleus of the rat. Brain Res (1983) 288:344–8. doi:10.1016/0006-8993(83)90115-4
237. Takatsuji K, Tohyama M. Geniculo-geniculate projection of enkephalin and neuropeptide Y containing neurons in the intergeniculate leaflet of the thalamus in the rat. J Chem Neuroanat (1989) 2:19–27.
238. Glass JD, Guinn J, Kaur G, Francl JM. On the intrinsic regulation of neuropeptide Y release in the mammalian suprachiasmatic nucleus circadian clock. Eur J Neurosci (2010) 31:1117–26. doi:10.1111/j.1460-9568.2010.07139.x
239. Biello SM, Janik D, Mrosovsky N. Neuropeptide Y and behaviorally induced phase shifts. Neuroscience (1994) 62:273–9. doi:10.1016/0306-4522(94)90331-X
240. Biello SM, Mrosovsky N. Blocking the phase-shifting effect of neuropeptide Y with light. Proc Biol Sci (1995) 259:179–87. doi:10.1098/rspb.1995.0026
241. Horikawa K, Yokota S, Fuji K, Akiyama M, Moriya T, Okamura H, et al. Nonphotic entrainment by 5-HT1A/7 receptor agonists accompanied by reduced Per1 and Per2 mRNA levels in the suprachiasmatic nuclei. J Neurosci (2000) 20:5867–73.
242. Fukuhara C, Brewer JM, Dirden JC, Bittman EL, Tosini G, Harrington ME. Neuropeptide Y rapidly reduces period 1 and period 2 mRNA levels in the hamster suprachiasmatic nucleus. Neurosci Lett (2001) 314:119–22. doi:10.1016/S0304-3940(01)02304-7
243. Maywood ES, Mrosovsky N. A molecular explanation of interactions between photic and non-photic circadian clock-resetting stimuli. Brain Res Gene Expr Patterns (2001) 1:27–31. doi:10.1016/S1567-133X(01)00005-9
244. Maywood ES, Okamura H, Hastings MH. Opposing actions of neuropeptide Y and light on the expression of circadian clock genes in the mouse suprachiasmatic nuclei. Eur J Neurosci (2002) 15:216–20. doi:10.1046/j.0953-816x.2001.01852.x
245. Maywood ES, Mrosovsky N, Field MD, Hastings MH. Rapid down-regulation of mammalian period genes during behavioral resetting of the circadian clock. Proc Natl Acad Sci U S A (1999) 96:15211–6. doi:10.1073/pnas.96.26.15211
246. Cutler DJ, Piggins HD, Selbie LA, Mason R. Responses to neuropeptide Y in adult hamster suprachiasmatic nucleus neurones in vitro. Eur J Pharmacol (1998) 345:155–62. doi:10.1016/S0014-2999(98)00012-0
247. Liou SY, Albers HE. Single unit response of neurons within the hamster suprachiasmatic nucleus to neuropeptide Y. Brain Res Bull (1991) 27:825–8. doi:10.1016/0361-9230(91)90216-7
248. van den Pol AN, Obrietan K, Chen G, Belousov AB. Neuropeptide Y-mediated long-term depression of excitatory activity in suprachiasmatic nucleus neurons. J Neurosci (1996) 16:5883–95.
249. Gribkoff VK, Pieschl RL, Wisialowski TA, van den Pol AN, Yocca FD. Phase shifting of circadian rhythms and depression of neuronal activity in the rat suprachiasmatic nucleus by neuropeptide Y: mediation by different receptor subtypes. J Neurosci (1998) 18:3014–22.
250. Prosser RA, Miller JD, Heller HC. A serotonin agonist phase-shifts the circadian clock in the suprachiasmatic nuclei in vitro. Brain Res (1990) 534:336–9. doi:10.1016/0006-8993(90)90153-3
251. Shibata S, Tsuneyoshi A, Hamada T, Tominaga K, Watanabe S. Phase-resetting effect of 8-OH-DPAT, a serotonin1A receptor agonist, on the circadian rhythm of firing rate in the rat suprachiasmatic nuclei in vitro. Brain Res (1992) 582:353–6. doi:10.1016/0006-8993(92)90156-4
252. Yamazaki S, Kerbeshian MC, Hocker CG, Block GD, Menaker M. Rhythmic properties of the hamster suprachiasmatic nucleus in vivo. J Neurosci (1998) 18:10709–23.
253. Schaap J, Meijer JH. Opposing effects of behavioural activity and light on neurons of the suprachiasmatic nucleus. Eur J Neurosci (2001) 13:1955–62. doi:10.1046/j.0953-816x.2001.01561.x
254. van OF, Lucassen EA, Houben T, VanderLeest HT, Antle MC, Meijer JH. Amplitude of the SCN clock enhanced by the behavioral activity rhythm. PLoS One (2012) 7:e39693. doi:10.1371/journal.pone.0039693
255. Leise TL, Harrington ME, Molyneux PC, Song I, Queenan H, Zimmerman E, et al. Voluntary exercise can strengthen the circadian system in aged mice. Age (2013) 35:2137–52. doi:10.1007/s11357-012-9502-y
256. Power A, Hughes AT, Samuels RE, Piggins HD. Rhythm-promoting actions of exercise in mice with deficient neuropeptide signaling. J Biol Rhythms (2010) 25:235–46. doi:10.1177/0748730410374446
257. Schroeder AM, Truong D, Loh DH, Jordan MC, Roos KP, Colwell CS. Voluntary scheduled exercise alters diurnal rhythms of behaviour, physiology and gene expression in wild-type and vasoactive intestinal peptide-deficient mice. J Physiol (2012) 590:6213–26. doi:10.1113/jphysiol.2012.233676
258. Cuesta M, Aungier J, Morton AJ. Behavioral therapy reverses circadian deficits in a transgenic mouse model of Huntington’s disease. Neurobiol Dis (2014) 63:85–91. doi:10.1016/j.nbd.2013.11.008
259. Yamanaka Y, Suzuki Y, Todo T, Honma K, Honma S. Loss of circadian rhythm and light-induced suppression of pineal melatonin levels in Cry1 and Cry2 double-deficient mice. Genes Cells (2010) 15:1063–71. doi:10.1111/j.1365-2443.2010.01443.x
260. Van Someren EJ, Lijzenga C, Mirmiran M, Swaab DF. Long-term fitness training improves the circadian rest-activity rhythm in healthy elderly males. J Biol Rhythms (1997) 12:146–56. doi:10.1177/074873049701200206
261. Buxton OM, Frank SA, L’Hermite-Baleriaux M, Leproult R, Turek FW, Van CE. Roles of intensity and duration of nocturnal exercise in causing phase delays of human circadian rhythms. Am J Physiol (1997) 273:E536–42.
262. Mistlberger RE, Skene DJ. Nonphotic entrainment in humans? J Biol Rhythms (2005) 20:339–52. doi:10.1177/0748730405277982
263. Teri L, Gibbons LE, McCurry SM, Logsdon RG, Buchner DM, Barlow WE, et al. Exercise plus behavioral management in patients with Alzheimer disease: a randomized controlled trial. JAMA (2003) 290:2015–22. doi:10.1001/jama.290.15.2015
264. Teri L, McCurry SM, Logsdon RG, Gibbons LE, Buchner DM, Larson EB. A randomized controlled clinical trial of the Seattle protocol for activity in older adults. J Am Geriatr Soc (2011) 59:1188–96. doi:10.1111/j.1532-5415.2011.03454.x
265. McCurry SM, Gibbons LE, Logsdon RG, Vitiello MV, Teri L. Nighttime insomnia treatment and education for Alzheimer’s disease: a randomized, controlled trial. J Am Geriatr Soc (2005) 53:793–802. doi:10.1111/j.1532-5415.2005.53252.x
266. Youngstedt SD. Effects of exercise on sleep. Clin Sports Med (2005) 24:355–65. doi:10.1016/j.csm.2004.12.003
267. Cohen R, Kronfeld-Schor N, Ramanathan C, Baumgras A, Smale L. The substructure of the suprachiasmatic nucleus: similarities between nocturnal and diurnal spiny mice. Brain Behav Evol (2010) 75:9–22. doi:10.1159/000282172
268. Schwartz WJ, Reppert SM, Eagan SM, Moore-Ede MC. In vivo metabolic activity of the suprachiasmatic nuclei: a comparative study. Brain Res (1983) 274:184–7. doi:10.1016/0006-8993(83)90538-3
269. Kubota A, Inouye ST, Kawamura H. Reversal of multiunit activity within and outside the suprachiasmatic nucleus in the rat. Neurosci Lett (1981) 27:303–8. doi:10.1016/0304-3940(81)90447-X
270. Sato T, Kawamura H. Circadian rhythms in multiple unit activity inside and outside the suprachiasmatic nucleus in the diurnal chipmunk (Eutamias sibiricus). Neurosci Res (1984) 1:45–52. doi:10.1016/0168-0102(84)90029-4
271. Kurumiya S, Kawamura H. Circadian oscillation of the multiple unit activity in the guinea pig suprachiasmatic nucleus. J Comp Physiol A (1988) 162:301–8. doi:10.1007/BF00606118
272. Mrosovsky N, Edelstein K, Hastings MH, Maywood ES. Cycle of period gene expression in a diurnal mammal (Spermophilus tridecemlineatus): implications for nonphotic phase shifting. J Biol Rhythms (2001) 16:471–8. doi:10.1177/074873001129002141
273. Caldelas I, Poirel VJ, Sicard B, Pevet P, Challet E. Circadian profile and photic regulation of clock genes in the suprachiasmatic nucleus of a diurnal mammal Arvicanthis ansorgei. Neuroscience (2003) 116:583–91. doi:10.1016/S0306-4522(02)00654-1
274. Yan L, Okamura H. Gradients in the circadian expression of Per1 and Per2 genes in the rat suprachiasmatic nucleus. Eur J Neurosci (2002) 15:1153–62. doi:10.1046/j.1460-9568.2002.01955.x
275. Hastings MH, Field MD, Maywood ES, Weaver DR, Reppert SM. Differential regulation of mPER1 and mTIM proteins in the mouse suprachiasmatic nuclei: new insights into a core clock mechanism. J Neurosci (1999) 19:RC11.
276. Bae K, Jin X, Maywood ES, Hastings MH, Reppert SM, Weaver DR. Differential functions of mPer1, mPer2, and mPer3 in the SCN circadian clock. Neuron (2001) 30:525–36. doi:10.1016/S0896-6273(01)00302-6
277. DeCoursey PJ, Krulas JR, Mele G, Holley DC. Circadian performance of suprachiasmatic nuclei (SCN)-lesioned antelope ground squirrels in a desert enclosure. Physiol Behav (1997) 62:1099–108. doi:10.1016/S0031-9384(97)00263-1
278. DeCoursey PJ, Krulas JR. Behavior of SCN-lesioned chipmunks in natural habitat: a pilot study. J Biol Rhythms (1998) 13:229–44. doi:10.1177/074873098129000075
279. Brown TM, Wynne J, Piggins HD, Lucas RJ. Multiple hypothalamic cell populations encoding distinct visual information. J Physiol (2011) 589:1173–94. doi:10.1113/jphysiol.2010.199877
280. Meijer JH, Rusak B, Harrington ME. Photically responsive neurons in the hypothalamus of a diurnal ground squirrel. Brain Res (1989) 501:315–23. doi:10.1016/0006-8993(89)90648-3
281. Jiao YY, Lee TM, Rusak B. Photic responses of suprachiasmatic area neurons in diurnal degus (Octodon degus) and nocturnal rats (Rattus norvegicus). Brain Res (1999) 817:93–103. doi:10.1016/S0006-8993(98)01218-9
282. Earnest DJ, Sladek CD. Circadian vasopressin release from perifused rat suprachiasmatic explants in vitro: effects of acute stimulation. Brain Res (1987) 422:398–402. doi:10.1016/0006-8993(87)90952-8
283. Katona C, Rose S, Smale L. The expression of Fos within the suprachiasmatic nucleus of the diurnal rodent Arvicanthis niloticus. Brain Res (1998) 791:27–34. doi:10.1016/S0006-8993(97)01092-5
284. Earnest DJ, Olschowka JA. Circadian regulation of c-fos expression in the suprachiasmatic pacemaker by light. J Biol Rhythms (1993) 8(Suppl):S65–71.
285. Gillette MU, Reppert SM. The hypothalamic suprachiasmatic nuclei: circadian patterns of vasopressin secretion and neuronal activity in vitro. Brain Res Bull (1987) 19:135–9. doi:10.1016/0361-9230(87)90176-6
286. Harrington ME, Nance DM, Rusak B. Double-labeling of neuropeptide Y-immunoreactive neurons which project from the geniculate to the suprachiasmatic nuclei. Brain Res (1987) 410:275–82. doi:10.1016/0006-8993(87)90325-8
287. Lincoln G, Messager S, Andersson H, Hazlerigg D. Temporal expression of seven clock genes in the suprachiasmatic nucleus and the pars tuberalis of the sheep: evidence for an internal coincidence timer. Proc Natl Acad Sci U S A (2002) 99:13890–5. doi:10.1073/pnas.212517599
288. Sumova A, Travnickova Z, Peters R, Schwartz WJ, Illnerova H. The rat suprachiasmatic nucleus is a clock for all seasons. Proc Natl Acad Sci U S A (1995) 92:7754–8. doi:10.1073/pnas.92.17.7754
289. Nuesslein-Hildesheim B, O’Brien JA, Ebling FJ, Maywood ES, Hastings MH. The circadian cycle of mPER clock gene products in the suprachiasmatic nucleus of the Siberian hamster encodes both daily and seasonal time. Eur J Neurosci (2000) 12:2856–64. doi:10.1046/j.1460-9568.2000.00173.x
290. Tournier BB, Menet JS, Dardente H, Poirel VJ, Malan A, Masson-Pevet M, et al. Photoperiod differentially regulates clock genes’ expression in the suprachiasmatic nucleus of Syrian hamster. Neuroscience (2003) 118:317–22. doi:10.1016/S0306-4522(03)00008-3
291. Johnston JD, Ebling FJ, Hazlerigg DG. Photoperiod regulates multiple gene expression in the suprachiasmatic nuclei and pars tuberalis of the Siberian hamster (Phodopus sungorus). Eur J Neurosci (2005) 21:2967–74. doi:10.1111/j.1460-9568.2005.04148.x
292. Leach G, Ramanathan C, Langel J, Yan L. Responses of brain and behavior to changing day-length in the diurnal grass rat (Arvicanthis niloticus). Neuroscience (2013) 234:31–9. doi:10.1016/j.neuroscience.2013.01.002
293. Cuesta M, Mendoza J, Clesse D, Pevet P, Challet E. Serotonergic activation potentiates light resetting of the main circadian clock and alters clock gene expression in a diurnal rodent. Exp Neurol (2008) 210:501–13. doi:10.1016/j.expneurol.2007.11.026
294. Cutrera RA, Saboureau M, Pevet P. Phase-shifting effect of 8-OH-DPAT, a 5-HT1A/5-HT7 receptor agonist, on locomotor activity in golden hamster in constant darkness. Neurosci Lett (1996) 210:1–4. doi:10.1016/0304-3940(96)12655-0
295. Horikawa K, Shibata S. Phase-resetting response to (+)8-OH-DPAT, a serotonin 1A/7 receptor agonist, in the mouse in vivo. Neurosci Lett (2004) 368:130–4. doi:10.1016/j.neulet.2004.06.072
296. Novak CM, Albers HE. Novel phase-shifting effects of GABAA receptor activation in the suprachiasmatic nucleus of a diurnal rodent. Am J Physiol Regul Integr Comp Physiol (2004) 286:R820–5. doi:10.1152/ajpregu.00575.2003
297. Dardente H, Menet JS, Challet E, Tournier BB, Pevet P, Masson-Pevet M. Daily and circadian expression of neuropeptides in the suprachiasmatic nuclei of nocturnal and diurnal rodents. Brain Res Mol Brain Res (2004) 124:143–51. doi:10.1016/j.molbrainres.2004.01.010
298. Mammen AP, Jagota A. Immunocytochemical evidence for different patterns in daily rhythms of VIP and AVP peptides in the suprachiasmatic nucleus of diurnal Funambulus palmarum. Brain Res (2011) 1373:39–47. doi:10.1016/j.brainres.2010.12.018
299. Rocha VA, Frazao R, Campos LM, Mello P, Donato J Jr, Cruz-Rizzolo RJ, et al. Intrinsic organization of the suprachiasmatic nucleus in the capuchin monkey. Brain Res (2014) 1543:65–72. doi:10.1016/j.brainres.2013.10.037
300. van den Pol AN, Tsujimoto KL. Neurotransmitters of the hypothalamic suprachiasmatic nucleus: immunocytochemical analysis of 25 neuronal antigens. Neuroscience (1985) 15:1049–86. doi:10.1016/0306-4522(85)90254-4
301. Smale L, Boverhof J. The suprachiasmatic nucleus and intergeniculate leaflet of Arvicanthis niloticus, a diurnal murid rodent from East Africa. J Comp Neurol (1999) 403:190–208. doi:10.1002/(SICI)1096-9861(19990111)403:2<190::AID-CNE4>3.0.CO;2-K
302. Card JP, Moore RY. The suprachiasmatic nucleus of the golden hamster: immunohistochemical analysis of cell and fiber distribution. Neuroscience (1984) 13:415–31. doi:10.1016/0306-4522(84)90240-9
Keywords: SCN, electrophysiology, diurnal, disease, aging, exercise, photoperiod, plasticity
Citation: Ramkisoensing A and Meijer JH (2015) Synchronization of biological clock neurons by light and peripheral feedback systems promotes circadian rhythms and health. Front. Neurol. 6:128. doi: 10.3389/fneur.2015.00128
Received: 13 February 2015; Accepted: 19 May 2015;
Published: 05 June 2015
Edited by:
Alena Sumova, Academy of Sciences of the Czech Republic, Czech RepublicReviewed by:
Niels C. Rattenborg, Max Planck Institute of Ornithology, GermanyHugh D. Piggins, University of Manchester, UK
Copyright: © 2015 Ramkisoensing and Meijer. This is an open-access article distributed under the terms of the Creative Commons Attribution License (CC BY). The use, distribution or reproduction in other forums is permitted, provided the original author(s) or licensor are credited and that the original publication in this journal is cited, in accordance with accepted academic practice. No use, distribution or reproduction is permitted which does not comply with these terms.
*Correspondence: Johanna H. Meijer, Laboratory for Neurophysiology, Department of Molecular Cell Biology, Leiden University Medical Center, Postal Zone S5-P, P.O. Box 9600, Leiden 2300 RC, Netherlands, j.h.meijer@lumc.nl