- Behavioural Ecology and Neurobiology, Institute of Zoology, University of Graz, Graz, Austria
Insect sounds dominate the acoustic environment in many natural habitats such as rainforests or meadows on a warm summer day. Among acoustic insects, usually males are the calling sex; they generate signals that transmit information about the species-identity, sex, location, or even sender quality to conspecific receivers. Males of some insect species generate signals at distinct time intervals, and other males adjust their own rhythm relative to that of their conspecific neighbors, which leads to fascinating acoustic group displays. Although signal timing in a chorus can have important consequences for the calling energetics, reproductive success and predation risk of individuals, still little is known about the selective forces that favor the evolution of insect choruses. Here, we review recent advances in our understanding of the neuronal network responsible for acoustic pattern generation of a signaler, and pattern recognition in receivers. We also describe different proximate mechanisms that facilitate the synchronous generation of signals in a chorus and provide examples of suggested hypotheses to explain the evolution of chorus synchrony in insects. Some hypotheses are related to sexual selection and inter-male cooperation or competition, whereas others refer to the selection pressure exerted by natural predators. In this article, we summarize the results of studies that address chorus synchrony in the tropical katydid Mecopoda elongata, where some males persistently signal as followers although this reduces their mating success.
Acoustic Communication in Insects
Grasshoppers, crickets, and katydids usually produce sound by stridulation, that is using a striated file-like body structure and associated structures that vibrate when they are rubbed across a sclerotized plectrum (peg). While crickets and katydids rub their forewings against each other, grasshoppers move their hind legs across a peg located at the base of their wings. The sound signals generated can be as short as 0.5 ms (i.e., the female acoustic reply in Phaneropterine species) or can last for many minutes or even longer (e.g., the calling songs of trilling katydids). Acoustic signals can also be classified according to the responses they evoke from conspecific receivers: signals that are generated in aggressive interactions with conspecific rivals are termed aggressive songs, whereas calling songs are used to attract mates (Heller, 1988). When within close range to females, males often generate courtship songs with reduced amplitudes, different temporal patterns, and carrier frequencies. In most species, only males generate acoustic signals, and the mute females approach the singing males (phonotaxis). In duetting species, females reply to signals produced by distant males by emitting a short acoustic signal, which then elicits male phonotaxis (Heller and von Helversen, 1986; Zimmermann et al., 1989). A general feature of acoustic signals in insects is their high degree of stereotypy and redundancy. Since acoustic signals serve as effective premating isolation barriers, they are highly diverse among species. The temporal signal pattern is particularly essential for species recognition among grasshoppers (von Helversen and von Helversen, 1975, 1998), katydids (e.g., Morris et al., 1975; Keuper and Kühne, 1983), and crickets (e.g., Walker, 1957, 1969; Popov and Shuvalov, 1977; Mhatre et al., 2011; Schmidt and Römer, 2011; Schmidt and Balakrishnan, 2015). The carrier frequencies can range from 1 to 2 kHz far into the ultrasonics, and signals can be broadband (as in many katydids) or fall within a narrow frequency band (most crickets). The selective advantage of using either broadband or narrow-band acoustic signals for sound transmission and perception in a noisy environment has been previously described (Rheinlaender and Römer, 1980; Schmidt and Römer, 2011; Schmidt et al., 2011, 2013; Schmidt and Balakrishnan, 2015).
After successfully detecting signals, receivers evaluate the temporal signal pattern to obtain information about the species identity of the signaler. When signal period is rather variable or males advertise themselves by producing long-lasting trills, the period of syllables (for definition, see Table 1) usually contains information about the species identity (e.g., Walker, 1957; Popov and Shuvalov, 1977; Doherty and Callos, 1991; Simmons, 1991; Cade and Cade, 1992). However, when males generate a group of syllables (termed chirps) at fixed time intervals, the signal period could be a cue that indicates species identity (e.g., Walker, 1969). With reference to the current topic of timing in music and speech, the latter is particularly important. The intrinsic signal period of males shows little variability in some acoustic insect species, and males listen and respond to the signals of conspecific neighbors. As a result, the signal timing of chorus members strongly deviates from random, whereby synchrony and signal alternation are extreme forms of temporal patterns that emerge from acoustic interactions. Since signal timing in a group can have important consequences for calling energetics, mate choice, and predation, researchers have been asking questions about the evolution of chorusing for decades. Before going into detail about the various causes and consequences of synchronous insect choruses, we will provide a brief review of recent advances in our understanding of the neuronal basis of signal pattern generation and rhythm perception in insects, both of which are basic requirements for acoustic communication.
Rhythm-Generating Neural Circuits
The temporal patterns of acoustic signals are generated by rhythm-generating networks of the central nervous system. Acoustic insects are valuable model organisms for the study of these networks because the rhythm of their songs is rather simple and their nervous system is rather primitive as compared to vertebrates or mammals. Another advantage is that neurons can be identified on the basis of their response properties and unique anatomy. This allows comparisons of the function of identified homologous neurons that are part of pattern-generating networks across species to be made, which provides important insights into the evolution of both temporal signal patterns and song diversification.
In order to attract females from a distance, males of the Mediterranean field cricket Gryllus bimaculatus emit calling songs that are characterized by aperiodic chirps consisting of about 4–5 syllables. Recently, the network involved in pattern generation was identified in this species. Schöneich and Hedwig (2011) located its position in the CNS by systematically dissecting the connection between abdominal ganglia (for a similar method, see Hennig and Otte, 1996). After transecting the connectives between the third thoracic ganglion (metathoracic ganglion complex) and the first abdominal ganglion, singing behavior was immediately and permanently terminated. Later, four neurons in these ganglia that showed rhythmic activity in phase with the syllable pattern were identified (Schöneich and Hedwig, 2012). Interestingly, a similar, characteristic neuroanatomy of the song pattern generator was found in the metathoracic-abdominal ganglion complex in grasshoppers, where songs are produced through rhythmic movements of hind legs (Gramoll and Elsner, 1987; Hedwig, 1992; Schütze and Elsner, 2001). Even more surprising, the neuronal circuit for courtship song production in drosophila (Clyne and Miesenböck, 2008; von Philipsborn et al., 2011) and rhythmic sound production via tymbals in arctiid moths (Dawson and Fullard, 1995) was also located in thoracic-abdominal ganglia. This suggests a common evolutionary origin for early thoracic-abdominal motor control networks, which may have been linked to ventilation (cf. Robertson et al., 1982; Dumont and Robertson, 1986). By gathering knowledge about the location and function of interneurons that constitute part of the central pattern generator, a framework for further comparative studies can be constructed. In such an attempt it would be worthwhile to investigate the neuronal basis that is responsible for rhythm adjustment in chorusing insects (see below).
Rhythm Perception and Associated Neuronal Correlates
Mate choice experiments performed with various field cricket and katydid species have revealed that the signal traits evaluated by receivers for species recognition are as diverse as the signals (e.g., Heller and von Helversen, 1986; Shaw et al., 1990; Simmons, 1991; Hennig and Weber, 1997; Hennig, 2003, 2009; Poulet and Hedwig, 2005; Greenfield and Schul, 2008; Hartbauer et al., 2014; Hennig et al., 2014). It has been generally accepted that temporal pattern recognition is both hardwired and genetically-determined as compared to olfaction and visual orientation, where learning also plays an important role (Bazhenov et al., 2005; Papaj and Lewis, 2012). To understand the principal mechanisms of species recognition and mate choice in insects, it is necessary to unravel the response properties both of auditory neurons that convey information about acoustic signals to the brain, and the filter network in the brain itself. The expectation in this research was to find a neuronal network and describe synaptic mechanisms that result in selective responses to the conspecific temporal song pattern, which matches the selectivity of these patterns in behavior. Two model organisms were used for this approach: the grasshopper Chorthippus biguttulus and the field cricket G. bimaculatus.
Male Ch. biguttulus grasshoppers generate temporally-structured signals via stridulation and females respond to the temporal pattern of syllable-pause combinations of attractive songs by emitting a short acoustic reply (von Helversen and von Helversen, 1998; Meckenhäuser et al., 2013). Females in this species prefer short pauses and a strong onset accentuation of song elements (von Helversen, 1972; Balakrishnan et al., 2001). Stumpner et al. (1991) studied the response of several neurons to conspecific song models and showed that, of various local neurons in the thorax, one neuron (BSN1) responded to varying syllable-pause combinations in a way that matched behavior. Two other thoracic neurons (SN6, AN4) responded to gaps in the verse of conspecific song models in a highly reliable manner (Stumpner and Ronacher, 1994). By selectively heating individual body segments, the brain was identified as the location where pattern recognition takes place, whereas the oscillator for song production was localized in the thoracic ganglia (Bauer and von Helversen, 1987; Gramoll and Elsner, 1987; Hedwig, 1992; Schütze and Elsner, 2001; Schöneich and Hedwig, 2012). The brain neurons involved in pattern recognition still need to be characterized in this species.
As already mentioned above, male G. bimaculatus attract distant females by producing calling songs that are made up of aperiodic chirps, each consisting of about four syllables. As in many other cricket species, the syllable period represents a crucial parameter for species recognition. Behavioral experiments revealed that song pattern recognition in G. bimaculatus relies on two computations with respect to time (Grobe et al., 2012). Using a modern modeling approach, Hennig et al. (2014) were able to simulate the response of females that listened to various calling song models with different temporal patterns by using a short integration time window that operated as a filter for the pulse rate and a longer integration time window that allowed the evaluation of song energy over time.
Recently, the neuronal network that enables pulse rate recognition in the brain of G. bimaculatus has been identified. It turned out that this complex task depends on the detection of the coincidence of successive pulses in a delay line network (Schöneich et al., 2015). Subsequent sound pulses are encoded in the bursting activity of a neuron that receives sensory input at the thorax and ascends to the brain (AN1). In the brain, the sensory information of this neuron is split into two parallel pathways, one involving two other neurons (LN2 and LN5). The processing of sensory information in these neurons leads to a moderate delay and, thus, to the coincidence of the bursting response of AN1 and LN5 in the postsynaptic neuron LN3 when pulses are separated by a syllable interval of more than 20 ms (see Figure 1A). While LN3 operates as coincidence detector, LN4 represents a feature detector that exhibits temporal band pass characteristics that are highly similar to those of the pulse period tuning of female phonotaxis (Figure 1B; Kostarakos and Hedwig, 2012). This feature-detection mechanism enables recognition of the species-specific temporal song pattern in this field cricket, and is a principal mechanism that evaluates the pulse period of calling songs.
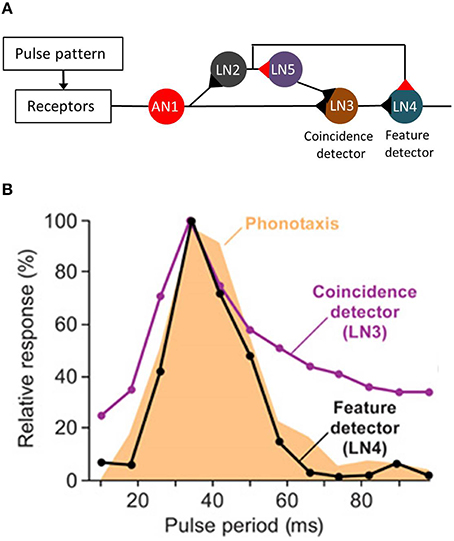
Figure 1. Pulse pattern recognition in G. bimaculatus. (A) Schematic drawing of the feature detector involved in the recognition of pulse rates. Black triangles represent the excitatory synapses and red triangles, the inhibitory synapses. LN2 and LN5 caused a delay of the nervous response in LN3 relative to the excitation mediated via AN1. (B) The pulse period tuning of the feature detector LN4 matched female preference. Modified from Schöneich et al. (2015).
Insect Choruses
In some insect species, males congregate in groups where they form acoustic leks (also referred to as “spree” in the temporal domain; Walker, 1983; Kirkpatrick and Ryan, 1991; Höglund and Alatalo, 1995). These male aggregations offer females the opportunity to compare the calling songs of several males simultaneously, which is principally different from sequentially comparing potential mating partners (Kokko, 1997). An analysis of signal timing in males within these aggregations revealed various forms of collective broadcasting where signal timing was non-random. Greenfield (1994a) reviewed various mechanisms for a joint display of signals in groups. These included: (1) Changing light conditions trigger the simultaneous activity of senders in dusk and dawn choruses. (2) Unison bout singing, triggered by males who initiate calling and are then joined by most other signalers. Participants in such choruses usually maintain a high signal rate for several minutes, after which the calling effort gradually decreases to zero. Then, the cycle is repeated after variable intervals of silence. (3) Periodic signal production can be controlled through a central pattern generator that leads to high precision of signal timing, if individuals in a group slowly adapt their signal period to the rhythm of others who exhibit similar, intrinsic, “free-running” signal periods (as in some synchronizing firefly species). (4) In some chorusing species, males are able to maintain a constant phase relationship between their signals and those of other males by responding with a phase shift to the signal produced by a neighbor. Depending on certain properties of signal oscillators and the number of participants, signals are either broadcast in collective synchrony or in a kind of alternation.
When singing within the hearing range of one other, males of the same species often time their signals strictly and temporally. Depending on chorus size and inter-male distance, males either alternate (e.g., Jones, 1963; Latimer, 1981; Meixner and Shaw, 1986; Tauber et al., 2001) or synchronize their periodic signals (Walker, 1969; Shaw et al., 1990; Sismondo, 1990; Greenfield and Roizen, 1993; Nityananda and Balakrishnan, 2007; Greenfield and Schul, 2008; Schul et al., 2014). Synchrony is often found in species that emit signals relatively rapidly (with a period of < 1 s), whereas alternation normally involves slower signal rhythms (a period of >1 s) (Greenfield, 1994b). In principle, alternation in periodic signals is restricted to only two signalers, whereas the number of individuals engaged in synchronous signaling is theoretically unlimited. Depending on the properties of song oscillators, synchrony can either lead to a significant overlap in signals or temporally-fixed delays of signals produced by different males. At close range, synchrony can be rather precise, so that even the syllables within the chirps are synchronized with those of neighboring males: when singing in close proximity, males of the chorusing species Amblycorypha parvipennis tend to synchronize the syllable pattern of their signals (Shaw et al., 1990). Synchronous signal displays are not restricted to the acoustic world, but can also be found in other modalities. Aggregating firefly species collectively broadcast visual displays in almost perfect synchrony, which results in fascinating group displays (Buck and Buck, 1968; Otte and Smiley, 1977; Buck et al., 1981). Furthermore, the vibratory communication signals of wolf spiders (Kotiaho et al., 2004) and the visual communication system of fiddler crabs (Backwell et al., 1998) are characterized by their high degree of synchrony.
Is there a common proximate mechanism that is responsible for synchronous signaling in these different systems? The oscillator properties that lead to synchronous signal displays were first described for fireflies, where a “phase delay model” was suggested to explain flash synchrony in these organisms (Hanson, 1978; Buck et al., 1981). Greenfield (1994b; see also Greenfield et al., 1997) modified this model, hypothesizing the existence of an inhibitory resetting mechanism of signal oscillators to explain the diversity of alternating and synchronous choruses observed among members of the different species. In this model, in the absence of a stimulus, the oscillator level constantly rises to a point where the production of a signal is triggered with a minor delay (effector delay). One important characteristic of this model is that the oscillator level is reset for the duration of the stimulus, which leads to a phase delay. However, the neuronal basis of this model has not yet been described.
While inhibitory resetting can lead to the rapid synchronization of signals in a chorus (e.g., Mecopoda elongata: Sismondo, 1990; Hartbauer et al., 2005), the degree of synchrony is much higher when the signalers mutually adjust their intrinsic signal rates. Mutual rhythm adjustment has been observed to lead to the attainment of almost perfect flash synchrony in firefly individuals (Ermentrout, 1991). Furthermore, a combination of inhibitory resetting and period adjustment is responsible for the high degree of signal overlap among chorusing katydids (Walker, 1969; Nityananda and Balakrishnan, 2007; Murphy et al., 2016). In the same way, perfect synchrony of humans has been attributed to both “phase correction” and “period adjustment” mechanisms (e.g., Semjen et al., 1998; Repp, 2001, 2005; see also Merker et al., 2009).
Evolution of Chorus Synchrony
How synchrony among different individuals could evolve in the absence of a central controlling instance within the group (i.e., an individual that would play a role similar to that of a conductor in an orchestra) is puzzling. Mechanisms that would ultimately favor the evolution of chorus synchrony are thought to be diverse and may have evolved in response to selective forces either driven by other chorus members, through female choice (see Section Female Choice and the Evolution of Chorus Synchrony) or natural predators (see Section Cooperation, Competition, and a Trade-Off between Natural and Sexual Selection). Males that advertise themselves in a chorus may gain one or more of the following mutual (group) benefits by timing signals (reviewed in Greenfield, 1994b): (1) Synchrony preserves a species-specific rhythm or a distinct call envelope that is offset by silent gaps (Walker, 1969; Greenfield and Schul, 2008). (2) In contrast, alternation ensures that females can detect, and discriminate critical signal features during mate choice. (3) Synchrony maximizes the peak signal amplitude of group displays, which is an emergent property also known as the “beacon effect” in the firefly literature (Buck and Buck, 1966, 1978). This property increases the conspicuousness of signals in a group of males as compared to that of a lone singer if females evaluate the peak signal amplitude rather than average signals over a longer period of time. This hypothesis states that males in a group can attract females from a greater distance by timing their signals to achieve nearly perfect synchrony. As a consequence, individuals in a chorus potentially increase their fitness as compared to isolated singing individuals. However, empirical evidence for the existence of a “beacon effect” in acoustic insects is rare and has been restricted to evidence from computer-model simulations of chorus synchrony evolution in an Indian Mecopoda species (Nityananda and Balakrishnan, 2009). A strong increase in the amplitude of synchronous acoustic signals was described in M. elongata (Hartbauer et al., 2014). For a description of other suspected “beacon effects” in bullfrog choruses see Bates et al. (2010) and in the vibratory communication of a treehopper, see Cocroft (1999). Whereas the hypotheses described above are based on sexual selection, the timing of communal displays may also be shaped by natural selection. For example, predators eavesdropping on the calling songs of signalers may have difficulty localizing an isolated signaler in a group of synchronously-signaling individuals due to their cognitive limitations (Otte, 1977; Tuttle and Ryan, 1982). In this way, males may benefit from a reduced per-capita rate of predation by signaling in groups (Lack, 1968; Wiley, 1991; Alem et al., 2011; Brunel-Pons et al., 2011).
The “rhythm conservation” hypothesis and the “beacon effect” hypothesis are not mutually exclusive in that they both explain the evolution of chorus synchrony in male assemblages as a result of inter-male cooperation. The first hypothesis assumes a low amount of variability in the signal period on a species level and suggests that this signal parameter includes important information about species identity, whereas the temporal pattern of syllables that make up chirps is considered to be less relevant. This assumption was recently tested using the katydid species M. elongata from Malaysia, males of which synchronize their periodic signals with a period of about 2 s in small choruses (Sismondo, 1990). Calling songs in this species consist of regular chirps that are made up of about 10 syllables increasing in amplitude. When individual males were allowed to synchronize with periodic white noise signals that lacked any fine-temporal pattern, about 80% of males succeeded as long as the signal period was limited to about 2 s (Hartbauer et al., 2012a). Similarly, males synchronized with a periodic stimulus that consisted of only three syllables. In another experiment, individual males were allowed to either signal in synchrony with a conspecific signal or an artificial, unstructured white noise signal, both of which were presented at 2 s intervals and of equal intensity. Interestingly, 65% of the males generated chirps in synchrony with the conspecific signal, whereas only 35% synchronized with the unstructured signal (see example in Figure 2). However, after introducing a phase transition by delaying the stimulus for 1 s, only 56% of chirps were produced in synchrony with the conspecific stimulus. These results demonstrate that males of this species responded primarily to the signal period and more or less ignored the fine temporal signal patterns. This may be adaptive when considering the potential masking of the fine syllable pattern during transmission.
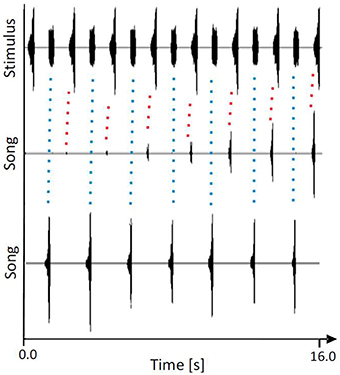
Figure 2. Representative example of a M. elongata male being offered the choice to produce chirps either in synchrony with periodic conspecific chirps (higher peak amplitude in the upper trace) or white noise pulses (lower peak amplitude), presented in alternation. Note that both signals exhibited the same acoustic energy. Middle panel: song initiation. Lower panel: stable entrainment. Note the phase-locking to the chirp that was observed at the onset of the song (indicated by red-dotted lines), but was thereafter observed in synchrony with the artificial pulse (indicated by blue-dotted lines). Modified from Hartbauer et al. (2012b).
Evidence for rhythm as an important signal parameter for species recognition was provided in the same species in female choice experiments. When given a choice between conspecific signals broadcast at different periods, females showed a preference for a fixed signal period of 2 s (Hartbauer et al., 2014). However, in choice tests with song models of periods < 1.5 s, females rarely approached any speaker. This is remarkable because the solo signal rate positively correlates with the energetic costs associated with song production (Hartbauer et al., 2012a). That is, if females selected males with higher signal rates they would thereby select males that invest more energy in mating displays. Their low rate of positive phonotaxis toward speakers with higher signal rates suggests stabilizing selection for the conspecific signal period.
Female Choice and the Evolution of Chorus Synchrony
As noted above, chorus synchrony can be a by-product of species recognition if signalers in a group preserve a species-specific temporal pattern (Greenfield, 1994a). The “rhythm conservation hypothesis” is exemplified by Neoconocephalus nebrascensis, where the male song requires strong amplitude modulations in order to elicit a phonotactic response in females (Deily and Schul, 2009). Thus, males are forced to synchronize the amplitude modulations of their signals when in male assemblages. A similar argument for the cooperative, synchronous display of mating signals has been put forward for the synchronously-flashing firefly Photinus carolinus. In this case, synchrony presumably reduces the visual “clutter” caused by randomly-timed, flashing signals (Copeland and Moiseff, 2010).
Darwin (1871) noted that female preference may promote the evolution of exaggerated mating displays. The evolution of such traits could be the result of a Fisherian process in which stronger preferences and more exaggerated traits coevolve (Fisher, 1915, 1930). In most communication systems, females prefer males that advertise themselves by producing conspicuous signals that are energetically expensive to produce. This is called “Zahavi's handicap principle” after Zahavi (1975), who explained the existence of such a preference by claiming that signals are reliable indicators of male quality when their production is expensive for the signaler, and that prolonged signaling lowers the fitness of the sender (reviewed in Johnstone, 1995). The energetic costs associated with the production of acoustic signals are usually determined by at least three signal parameters: duration, amplitude, and signal rate (Prestwich, 1994; Reinhold et al., 1998; McLister, 2001; Robinson and Hall, 2002). In the context of mate choice, these signal parameters are regarded as “condition-dependent handicaps,” which indicate the quality of a sender (West-Eberhard, 1979; Andersson, 1994). Furthermore, signal traits that provide true information about the phenotypic and genetic qualities of the senders and exclude the possibility of cheating are known as “revealing handicaps” (Maynard Smith, 1985, 1991).
On the other hand, preferences for certain signal traits may be the outcome of a sensory bias in receivers that already existed before signalers evolved the traits to exploit it. In a mating context, this hypothesis suggests that, when confronted with a choice situation, females do not necessarily select males on the basis of their acoustic signal traits (indicative of male quality). Instead, certain signals can more strongly stimulate the sensory system in receivers, increasing the likelihood of mating (Ryan, 1990; Ryan et al., 1990; Kirkpatrick and Ryan, 1991; Ryan and Keddy-Hector, 1992; Arak and Enquist, 1993). For example, males of lebinthine crickets generate unusually high-frequency calls that elicit a startle response in females. In response to these calls, females generate vibratory signals that allow males to locate them (ter Hofstede et al., 2015). Arak and Enquist (1995) provided some examples in which the sensory bias in receivers creates competition between senders, with the result of more conspicuous and costly signals.
In male aggregations of anurans and katydids, females often select males on the basis of relative signal timing rather than other signal features (Greenfield, 1994b; Gerhardt and Huber, 2002). Such mating systems are especially interesting for evolutionary biologists since, by choosing males on this basis, there are no obvious direct or indirect fitness benefits for females (Alexander, 1975; Greenfield, 1994b). Any preference for a certain temporal relationship between competing signals drives the evolution of mechanisms that enable the exact timing of signals generated in a group. This “receiver bias” hypothesis suggests that synchrony or alternation has emerged as a consequence of inter-male rivalry due to inter-sexual selection (e.g., Alexander, 1975; Arak and Enquist, 1993; Greenfield, 1994a,b, 1997; Greenfield et al., 1997; Snedden and Greenfield, 1998; Gerhardt and Huber, 2002; Copeland and Moiseff, 2010). Therefore, by studying signal interactions among males in a chorus and their evaluation by receivers, one can study traits and selection at different levels. In feedback loops, traits emerge at the group level and influence the evolution of signal timing mechanisms at the individual level (Greenfield, 2015; Party et al., 2015).
Leader Preference
In male assemblages, the synchronicity of calls is usually limited in precision, with some signals leading others. Relative signal timing can enhance or reduce male attractiveness if the females exhibit a preference for a certain temporal relationship between signals displayed in imperfect synchrony. Indeed, some anurans prefer signals that are timed in advance to others (leader signals) (reviewed in Klump and Gerhardt, 1992) which was also observed in many Orthopteran species (Shelly and Greenfield, 1991; Greenfield and Roizen, 1993; Minckley and Greenfield, 1995; Galliart and Shaw, 1996; Greenfield et al., 1997; Snedden and Greenfield, 1998). Such a preference constitutes a precedence effect, which is defined as the preference for the leading signal when two closely-timed, identical signals are presented from different directions [humans (Zurek, 1987; Litovsky et al., 1999), Mammals, birds, frogs, and insects (Cranford, 1982; Wyttenbach and Hoy, 1993; Greenfield et al., 1997; Dent and Dooling, 2004; Lee et al., 2009; Marshall and Gerhardt, 2010)]. This preference may be due to the fact that the leading signal suppresses the echo (reverberation) of subsequent signals that reach the receiver in a complex acoustic environment and, thus, improves sound localization.
Neoconocephalus spiza is a well-studied example of a synchronizing katydid species in which females display a strong leader preference. As a consequence, individual males compete in an attempt to jam one other's signals, with synchrony emerging as an epiphenomenon (Greenfield and Roizen, 1993; Snedden and Greenfield, 1998). The observation that males regularly switch between leader and follower roles in duets, exhibiting similar “free-running” chirp periods, provides support for the hypothesis that an ongoing competition for leadership exists (Greenfield and Roizen, 1993). In this species, males stop producing unattractive follower signals within a certain critical period of time after perceiving the signals from competitors (the so-called “forbidden interval”). Unlike N. spiza males, males of M. elongata establish mostly fixed temporal relationships for their signals over long periods of time, so that individual males assume either leader or follower roles during the duet (Hartbauer et al., 2005). Even in small four-male choruses, individuals often maintain either the leader or follower role over long periods of time (Hartbauer et al., 2014). The relative timing of synchronized chirps of different males strongly influences female choice. In two-choice experiments, M. elongata females showed a strong preference for those chirps leading by only 70–140 ms (Fertschai et al., 2007; Hartbauer et al., 2014). There is also a trade-off between time and intensity: the advantage of a signal leading by 140 ms can be compensated by an increase in loudness of follower signals by 8 dB (for similar trade-offs in other synchronizing insects and some anuran species, see Klump and Gerhardt, 1992; Greenfield, 1994b; Howard and Palmer, 1995; Grafe, 1996; Greenfield et al., 1997; Snedden and Greenfield, 1998; Höbel, 2010). The relatively high intensity value that is necessary for leader compensation implies that females must be in close proximity to the follower to prefer this male from a chorus. As a consequence, males who persistently signal as followers in a chorus should have a reduced fitness, posing an intriguing question about the evolutionary stability of follower roles. Before discussing hypotheses that may provide an answer to this question (see Section Cooperation, Competition, and a Trade-Off between Natural and Sexual Selection), we describe an oscillator property that favors the ability of males to attain call leadership in a chorus, and results obtained from a realistic computer model of a M. elongata chorus.
An Oscillator Property Responsible for Attaining Leadership
Sismondo (1990) demonstrated that synchrony and alternation in M. elongata are consequences of song oscillator properties, which can be illustrated in the form of phase response curves. In entrainment experiments and using realistic computer models, we demonstrated that males could establish stable synchrony and bi-stable alternation of signals over a broad range of stimulus periods, covering the whole spectrum of solo chirp periods found in a male population (1.7–2.4 s; Hartbauer et al., 2005). However, the synchrony observed was not perfect, and males tended to produce their chirps as a leader only if interacting with a male that exhibited a slower intrinsic signal rate. The member of the duet with the shorter chirp period (i.e., a difference of more than 150 ms in the intrinsic signal period duration) had an increased probability of attaining leadership (Hartbauer et al., 2005). This correlation between the intrinsic signal period and lead probability has also been described in the firefly P. cribellata (e.g., Buck et al., 1981) and two other katydid species (Meixner and Shaw, 1986; Greenfield and Roizen, 1993).
A Realistic Model of a M. elongata Chorus
Once a realistic model of male duets had been established (Hartbauer et al., 2005), the model was extended to simulate a chorus that consisted of 15 artificial males (Hartbauer, 2008). A major advantage of this approach is that manipulations of receiver properties and chorus composition could be performed that greatly exceeded those possible in behavioral experiments. In particular, parameters such as chorus density, selective attention paid to a neighbor subset, and temporal variability of synchrony due to males joining or leaving a chorus could be modified.
The results of chorus simulations revealed that synchrony in M. elongata is the outcome of an ongoing phase resetting process that propels song oscillators forward and backward during every cycle. Therefore, synchrony in M. elongata seems to be maintained on a chirp-to-chirp basis and does not depend on the mutual adjustment of intrinsic signal periods, as in a firefly (Ermentrout, 1991) or a katydid species (Murphy et al., 2016). Even in rather complex chorus situations, in which the signal oscillators and inter-male distances between nearest neighbors varied, agents that signaled at faster intrinsic rates established the leadership position more often than other chorus members. These simulation results were confirmed in real M. elongata choruses that consisted of 3–4 equally spaced males. In this situation, a single male led more than 50% of all signal interactions in 68% of choruses (Hartbauer et al., 2014). A correlation could also be drawn between the intrinsic signal period and the likelihood of producing leader signals in an Indian Mecopoda species (Nityananda and Balakrishnan, 2007). Unlike the Malaysian M. elongata species, males of the Indian species also altered their intrinsic signal period to match that of their competitors, a behavior that did not allow for the establishment of consistent leader and follower roles (Nityananda and Balakrishnan, 2008).
Manipulation of Chorus Density
An analysis of data from computer simulations also revealed that removing two or three agents from a synchronous chorus had only a minor effect on chorus synchrony, whereas adding agents who initially signaled at random phases greatly disturbed synchrony (Hartbauer, 2008). Therefore, in order to avoid a temporal loss of synchrony, males joining a synchronous chorus should already be phase-locked with other chorus members. Empirical evidence for such synchronous initiation of songs has recently been provided for Neoconocephalus ensiger (Murphy et al., 2016). Males of this katydid species seem to adjust the intrinsic signal period of their song oscillators prior to initiating the song in order to match the rate of periodic signals. Phase-locked song initiation behavior was also observed in males that were stimulated with a periodic pacer (Hartbauer, 2008). This behavior may be regarded as an adaptation to counteract the vulnerability of a synchronous chorus.
Selective Attention
Based on the results of computer simulations, Greenfield et al. (1997) argued that selective attention must be paid to a subset of males before synchrony and, especially, alternation can become a evolutionarily-stable signaling strategy. Selective attention can be gained at the neuronal, behavioral and ecological level and restricts the receivers' attention to signals broadcast by neighbors. Evidence for selective attention at the behavioral level has been provided from playback experiments conducted with alternating grasshopper and katydid species (Greenfield and Snedden, 2003). Individuals of these species need to pay selective attention to close neighbors when alternating in a chorus because, in principle, strict signal alternation is limited to only two acoustically interacting males. Evidence for selective attention at the neuronal level has been found by studying the membrane properties of individual interneurons; when signals that differ in loudness compete, the representation of the softer signal is suppressed (Pollack, 1988; Römer and Krusch, 2000). This enables receivers in a chorus to pay selective attention to the loudest signaler. Similarly, inhibitory mechanisms may result in a stronger representation of leader signals in imperfect synchrony (Nityananda et al., 2007). Despite the neuronal evidence for selective attention to leading signals, field studies indicate that the spacing of males appears to play a more important role in restricting the attention of a receiver to close neighbors (Nityananda and Balakrishnan, 2008). Simulating selective attention to only three nearest neighbors in a chorus model did not alter the likelihood of males with higher intrinsic signal rates to attain call leadership, but waves of synchronized signaling spread out among the agents (Hartbauer, 2008). This phenomenon, which is known as “wave-synchrony,” has also been observed in fireflies that flash in synchrony. It has inspired the development of a Mecopoda-based controller that enables the navigation of a swarm of autonomous micro-robots (Hartbauer and Römer, 2007).
Is Chorus Synchrony in M. elongata the Outcome of a Sensory Bias?
One proximate explanation for the preference of females for leading signals in behavior is based on a sensory bias in receivers. In the auditory system of insects, like in other vertebrates and mammals, direction-sensitive interneurons receive excitatory and inhibitory input from opposite auditory sides (review in Hedwig and Pollack, 2008). Thus, for a female receiver located between two acoustically interacting males, the signals of leader and follower males are asymmetrically represented in the auditory pathway, depending on the timed interaction of excitation and inhibition (Römer et al., 2002). Given that the leader signal has a temporal advantage, it may effectively suppress the representation of the follower signal, and the different representation of otherwise identical signals may bias the orientation of the female to the leader. The interaction of excitatory and inhibitory input may also explain quantitative values in time-intensity trading (Römer et al., 2002; Fertschai et al., 2007). In the auditory system of katydids, two interneurons that have properties favoring leading signals in a choice situation have been examined and may convey leader-biased bilateral information (Römer et al., 2002; Siegert et al., 2011). Depending on the strength of inhibition, the response to lagging signals was almost completely suppressed during the presentation of leading signals. Time-intensity-trading experiments revealed that follower signals needed a 15–20 dB advantage to compensate for the follower role, depending on the magnitude of the time difference.
However, the crucial question in the context of a possible sensory bias is whether the leader-biased response of auditory neurons evolved before or after male synchrony. It has been commonly accepted that a sensory bias can be the by-product of a sensory mechanism that evolved in a non-sexual context (Endler and McLellan, 1988; Ryan, 1990; Ryan et al., 1990; Kirkpatrick and Ryan, 1991; Ryan and Keddy-Hector, 1992; Arak and Enquist, 1993; Boughman, 2002; Arnqvist, 2006) and, therefore, that it already existed before signalers evolved traits to exploit it (“sensory exploitation” hypothesis) (Ryan and Rand, 1990, 1993; Ryan et al., 1990; Ryan, 1999). Ultimately, any bias in sensory processing with respect to closely timed signals has the potential to drive the evolution of communal signal displays toward synchrony or alternation (Greenfield, 1994a).
Strong support for the “sensory bias” hypothesis in Mecopoda would be the demonstration that in distantly-related orthopteran species, where synchrony does not occur, the responses to lagging signals in directionally-sensitive interneurons are also suppressed. The results of experiments conducted with locusts and field crickets have, thus far, been ambiguous (Figure 3). A recent phylogenetic study conducted in the genus Neconocephalus, in which—with the exception of one species—discontinuously-calling species synchronize their calls (Greenfield, 1990; Greenfield and Schul, 2008; Deily and Schul, 2009) revealed that females do not always show a strong leader preference, which does not support the “sensory bias” hypothesis (Greenfield and Schul, 2008). The most parsimonious explanation for imperfect synchronous chorusing in M. elongata is that the phase change mechanism in males enables them to synchronize their chirps, and females choose leading males as a passive consequence of the precedence effect in the auditory system (see also Party et al., 2014). However, it is also possible that a feedback loop, which originated from a sensory bias, exists that gradually strengthened the leader preference once imperfect chorus synchrony had been established.
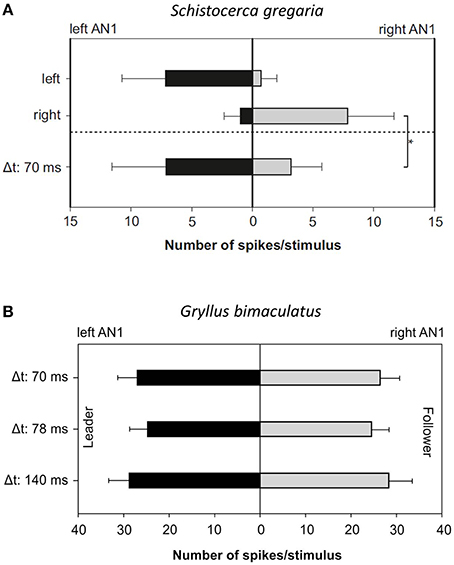
Figure 3. Summary of the bilateral AN1 responses to a Mecopoda chirp in Schistocerca gregaria and G. bimaculatus. The chirp of a solo-singing Mecopoda was presented individually from both sides (left and right) or as a leader–follower presentation with a time lag of 70 ms. (A) The mean bilateral AN1 response of five S. gregaria individuals indicated a significantly stronger excitation on the leader side (p < 0.01; Mann–Whitney Rank Sum Test, Bonferroni corrected). (B) No significant differences at three different time delays were observed in G. bimaculatus (average responses obtained from 5 individuals).
The Adaptive Nature of a Sensory Bias
Whether a sensory bias can be adaptive or not is still a matter of debate. Female choice based on a sensory bias may provide the females with fitness benefits due to lower search costs, even if the choice does not result in offspring with superior genes that are associated with positive fitness consequences (Kirkpatrick, 1987; Guilford and Dawkins, 1991; Hill, 1994; Dawkins and Guilford, 1996). This seems to hold true for M. elongata females, since positive phonotaxis lasted three times longer when identical chirps were presented in strict alternation, as compared to a leader-follower situation (Fertschai et al., 2007). Such delayed responses to alternating chirps can be explained at the neuronal level, since alternating chirps elicit identical—and, thus, ambiguous—neuronal excitation on both sides, whereas leading signals cause asymmetrical responses in favor of the leader, which would allow females to reliably choose between two similar, alternative signals. Therefore, females that quickly choose from among males may enjoy fitness benefits by reducing the risk of predation that is associated with a prolonged search for mates (e.g., Belwood and Morris, 1987; Siemers and Güttinger, 2006).
The solo chirp rate of M. elongata is an important predictor for leadership in acoustic interactions between males. If this parameter were correlated with traits that indicated male quality such as body size or fertility, females would gain fitness benefits by choosing the leader from among a group of males. However, neither male age, body size, spermatophore volume, or the number of living offspring correlated with the solo chirp period of individual males (Hartbauer et al., 2015), corroborating the results of a nutritional study in which the solo chirp rate was shown to be a poor predictor of nutritional status (Hartbauer et al., 2006). Similarly, in the European tree frog Hyla arborea, the quality of males did not correlate with signal timing, although females preferentially oriented toward the first of two identical calls that overlapped in time (Richardson et al., 2008). In this frog species and in the katydid Ephippiger ephippiger, call leadership and overall energetic investment in acoustic signals correlated positively (Berg and Greenfield, 2005). In this respect, the systems in H. arborea and E. ephippiger are analogous to that of M. elongata where the probability of producing leader signals depends on a trait (intrinsic signal period) that is associated with calling energetics (Hartbauer et al., 2006), but does not correlate with indicators of male fitness. In the same way, female E. diurnus do not gain any obvious benefits by preferring leading calls although males are able to adjust the song oscillator phase to establish leadership (Party et al., 2014).
Cooperation, Competition, and a Trade-Off Between Natural and Sexual Selection
Why do some M. elongata males participate in a chorus although they are less attractive for females as followers and probably would be more successful singing in isolation? One possible explanation may be that, in some species, females prefer signals that emerge from group displays over signals produced by lone singing males, which forces males to congregate [insects (Morris et al., 1978; Cade, 1981; Doolan and Mac Nally, 1981; Shelly and Greenfield, 1991), Hyla microcephala (Schwartz, 1994); but see Party et al., 2015]. Choice tests performed with M. elongata females confirmed their preference for conspicuous group displays (Hartbauer et al., 2014). However, this result does not explain why leader and follower roles were maintained by individuals in M. elongata choruses, where followers were at a disadvantage due to the strong female preference for signals from leaders (Fertschai et al., 2007). Below, several alternative, although not mutually exclusive, hypotheses are presented to explain why persistent followers still exist in M. elongata:
(1) Signaling as a follower may be beneficial when resulting from inter-male cooperation because overlapping chirps in a chorus may amplify the peak amplitude of the signals that are displayed synchronously (Figure 4A), and the resulting “beacon effect” may help distant receivers detect communal displays (see Figure 4B). In this case, females seem to evaluate the peak signal amplitude of communal displays, rather than average acoustic power. Interestingly, sound recordings revealed an elevated sound pressure level in the order of 6 dB in a chorus consisting of 3–4 acoustically-interacting M. elongata males (2 m nearest-neighbor distance; Hartbauer et al., 2014). Despite imperfect synchrony, the high degree of signal overlap found in this chorus situation resulted in an average increase of the root-mean-square amplitude that is almost identical to that found during the simultaneous playback of four identical, conspecific signals that perfectly overlapped in time. Given the fact that syllables comprising male chirps are interrupted by brief pauses, this result is surprising and may be attributed to signal plasticity, which is known to increase the probability of temporal overlap among the loud syllables of leader and follower signals (Hartbauer et al., 2012a). As a result, signal overlap in “four male choruses” is so high that the average duration of jointly produced signals is only 1.4 times longer (343 ms) as compared to the average signal duration of solo singing males (250 ms). It is also interesting to note that the increased signal amplitude of communal signal displays was a prerequisite for the successful simulation of the evolution of chorus synchrony in an Indian Mecopoda chirper, where females also preferred “leader males” (Nityananda and Balakrishnan, 2009). This observation is in contrast to results gathered for Achroia grisella (wax moth) leks, for which such a prerequisite does not exist (Alem et al., 2011).
An inherent problem encountered when interpreting many group effects is the dilution of per capita mating success as compared to that of lone singing males. However, the increased amplitudes of group displays may enhance the mating probabilities of individual males if one considers the noisy background against which acoustic communication often takes place. Given these complex acoustic conditions, overlapping signals may allow individuals to increase the conspicuousness of their rhythmic signals in a group. Additionally, enhanced group signals were more attractive for females as compared to the solo song of a male (Hartbauer et al., 2014). These data suggest that chorus synchrony in M. elongata is the outcome of inter-male cooperation, whereby even follower males may benefit from higher mating opportunities (but see the next argument).
(2) Inter-male competition for attractive leading signals may explain the high degree of signal overlap in a Mecopoda chorus. If chorus synchrony in M. elongata is the outcome of such competition, males that intrinsically produce signals more rapidly are expected to maintain similar or even slightly higher signal rates in a chorus compared to solo singing, although reduced signal rates in a chorus would facilitate signal overlap with competitors. Results obtained in small choruses consisting of 3–4 males seem to support this “competitive hypothesis” because consistent leader males increased their signal rate by 4% on average in choruses as compared to when they sang in isolation (Hartbauer et al., 2014). Therefore, the observed “beacon effect” is likely the by-product of inter-male competition for the attractive leader role rather than a cooperative effort to increase the peak signal amplitude of rhythmic communal mating displays.
(3) Although inter-male competition for attractive leader signals may explain chorus synchrony, it fails to explain the evolutionary stability of followers in a M. elongata chorus. An alternative hypothesis suggests that sustained signaling as a follower is an evolutionary stable signaling strategy if a trade-off exists between mate attraction and conspicuousness to predators/parasitoids. In field studies, we observed a tachinid fly homing in on M. elongata males (Figure 5). This fly belongs to one of 13 different species of Ormiin parasitoid flies that parasitize crickets and katydids in Asia (Lehmann, 2003). Lee et al. (2009) showed that Ormia ochracea (Diptera, Tachinidae), a tachinid fly that parasitizes field crickets, selectively orients toward the leading of two—otherwise identical—sound sources, while the lagging source had a minimal influence on the orientation of the fly. Therefore, the parasitoid fly homing in on M. elongata males may exhibit a similar leader preference as Mecopoda females, and these males would consequently suffer higher costs when signaling as leaders (review in Zuk and Kolluru, 1998). Because parasitoids are detrimental to survival and reproduction in crickets, katydids and cicada [Crickets (Cade, 1975; Zuk et al., 1998), katydids (Lehmann and Heller, 1998) and the cicada (Lakes-Harlan et al., 2000)], this hypothesis requires further testing. Ultimately, the existence of a leader preference in parasitoid flies suggests that the maintenance of follower singing in M. elongata is an evolutionary stable signaling strategy that trades lower attractiveness against reduced parasitation risk. Apparently, further studies are needed to quantify the selection pressure of this parasitoid fly on the signaling system of M. elongata.
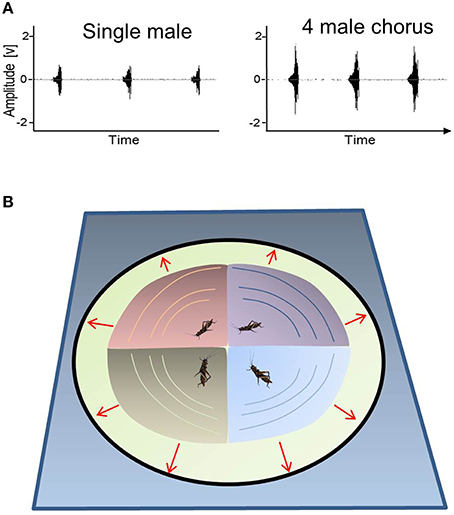
Figure 4. Signal overlap in M. elongata and model of the extension of acoustic space as the result of chorus synchrony. Four males singing in synchrony overlapped their periodic signals to a high degree. This led to a strong increase in signal amplitude (A) and to the enlargement of acoustic space (B). In this way, a group of synchronized males can attract females from a greater distance as compared to lone singing males. In the case of signal alternation, the area in which a single male signals at higher amplitude as compared to its competitors is strongly reduced (shown as areas with different colors).
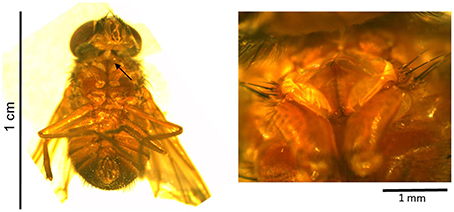
Figure 5. Habitus (left) and hearing organ (right) of a female tachinid fly of an unknown Tachinid species homing in on M. elongata males. Arrow indicates the position of the fly's ear. This fly belongs to the tribe Ormiini of an unknown genus (potentially Therobia, Phasioormia, or Homotrixa).
A summary of various selection pressures that favor chorus synchrony in M. elongata is illustrated in Figure 6. Females prefer males that signal at a conspecific period of about 2 s, which forces males to synchronize their signals in a group in order to maintain this species-specific rhythm. Since females also prefer leading signals, males in a group compete for the leader role, whereby chorus synchrony emerges as a by-product (Hartbauer et al., 2014). However, chorus synchrony is imperfect and leader and follower roles often remain stable for long periods of time. The natural selection exerted by parasitoid flies that infest singing leader males may stabilize persistent follower roles. Signaling as a follower is disadvantageous in terms of reproductive success, but results in a lower risk of falling victim to a parasitoid fly (selfish strategy). Additionally, followers that persistently signal can benefit from the “beacon effect,” which extends the acoustic space in such a way as to allow females to detect conspicuous group signals. Since females more frequently approached groups producing conspicuous group signals in a choice situation as opposed to a lone singing male producing a quieter song (Hartbauer et al., 2014), males that join a synchronous chorus may increase both their mating chances and the chances of all chorus members. Additionally, computer simulations have been used to demonstrate an increase in the per capita mating possibilities for chorus members advertising themselves in a noisy acoustic environment due to strongly-operating “beacon effects” (chorus size = 4 males, inter-male distance = 10 m; Hartbauer et al., 2014). Therefore, sexual selection favors synchronous group displays, but follower roles are evolutionarily stabilized as a consequence of emergent group properties (beacon effect) and natural selection.
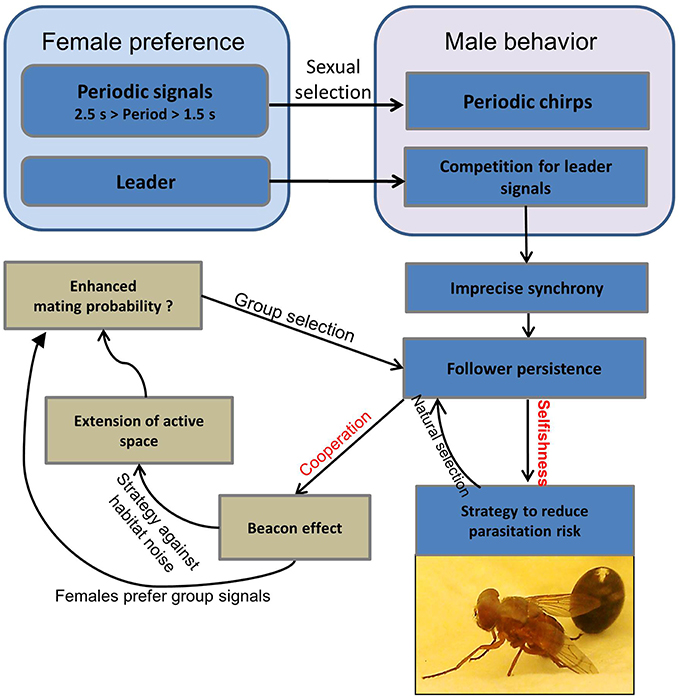
Figure 6. Selection pressures potentially involved in the evolution of chorus synchrony in M. elongata. For explanation, see text (Section Cooperation, Competition, and a Trade-off between Natural and Sexual Selection). Parasitoid flies hatch from puparia (visible as the ball in the background).
Ethics Statement
Insects that were used in this study were taken from a laboratory breed and do not belong to endangered species. Neurophysiological experiments have been performed in accordance with Austrian animal welfare laws.
Author Contributions
MH has drafted and written this manuscript. HR contributed with helpful comments and corrections.
Funding
This research was funded by the Austrian Science Fund (FWF) [P21808-B09].
Conflict of Interest Statement
The authors declare that the research was conducted in the absence of any commercial or financial relationships that could be construed as a potential conflict of interest.
Acknowledgments
We thank H. Rosli, University of Malaya, Kuala Lumpur, for his generous help with the establishment of Mecopoda breeds. Sincere thanks are given to two reviewers for valuable suggestions and comments.
References
Alem, S., Koselj, K., Siemers, B. M., and Greenfield, M. D. (2011). Bat predation and the evolution of leks in acoustic moths. Behav. Ecol. Sociobiol. 65, 2105–2116. doi: 10.1007/s00265-011-1219-x
Alexander, R. D. (1975). “Natural selection, specialized chorusing behavior in acoustical insects,” in Insects, Science and Society, ed D. Pimentel (New York, NY: Academic Press), 35–77.
Arak, A., and Enquist, M. (1993). Hidden preferences and the evolution of signals. Philos. Trans. R. Soc. Lond. B 340, 207–213. doi: 10.1098/rstb.1993.0059
Arak, A., and Enquist, M. (1995). Conflict receiver bias and the evolution of signal form. Philos. Trans. R. Soc. Lond. B 349, 337–344. doi: 10.1098/rstb.1995.0122
Arnqvist, G. (2006). Sensory exploitation and sexual conflict. Philos. Trans. R. Soc. Lond. B 361, 375–386. doi: 10.1098/rstb.2005.1790
Backwell, P., Jennions, M., Passmore, N., and Christy, J. (1998). Synchronized courtship in fiddler crabs. Nature 391, 31–32. doi: 10.1038/34076
Balakrishnan, R., von Helversen, D., and von Helversen, O. (2001). Song pattern recognition in the grasshopper Chorthippus biguttulus: the mechanism of syllable onset and offset detection. J. Comp. Physiol. A 187, 255–264. doi: 10.1007/s003590100197
Bates, M. E., Cropp, B. F., Gonchar, M., Knowles, J., Simmons, J. A., and Simmons, A. M. (2010). Spatial location influences vocal interactions in bullfrog choruses. J. Acoust. Soc. Am. 127, 2664–2677. doi: 10.1121/1.3308468
Bauer, M., and von Helversen, O. (1987). Separate localization of sound recognizing and sound producing neural mechanisms in a grasshopper. J. Comp. Physiol. A 161, 95–101. doi: 10.1007/BF00609458
Bazhenov, M., Stopfer, M., Sejnowski, T. J., and Laurent, G. (2005). Fast odor learning improves reliability of odor responses in the locust antennal lobe. Neuron 46, 483–492. doi: 10.1016/j.neuron.2005.03.022
Belwood, J. J., and Morris, G. K. (1987). Bat predation and its influence on calling behaviour in Neotropical katydids. Science 238, 64–67. doi: 10.1126/science.238.4823.64
Berg, A., and Greenfield, M. D. (2005). Sexual selection in insect choruses: influences of call power and relative timing. J. Insect Behav. 18, 59–75. doi: 10.1007/s10905-005-9347-7
Boughman, J. W. (2002). How sensory drive can promote speciation. Trends Ecol. Evol. 17, 571–577. doi: 10.1016/S0169-5347(02)02595-8
Brunel-Pons, O., Alem, S., and Greenfield, M. D. (2011). The complex auditory scene at leks: balancing antipredator behaviour and competitive signalling in an acoustic moth. Anim. Behav. 81, 231–239. doi: 10.1016/j.anbehav.2010.10.010
Buck, J., and Buck, E. (1966). Biology of synchronous flashing of fireflies. Nature 211, 562–564. doi: 10.1038/211562a0
Buck, J., and Buck, E. (1968). Mechanism of rhythmic synchronous flashing of fireflies. Science 159, 1319–1327. doi: 10.1126/science.159.3821.1319
Buck, J., and Buck, E. (1978). Toward a functional interpretation of synchronous flashing by fireflies. Am. Nat. 112, 471–492. doi: 10.1086/283291
Buck, J., Buck, E., Case, J. F., and Hanson, F. E. (1981). Control of flashing in fireflies. J. Comp. Physiol. A 144, 287–298. doi: 10.1007/BF00612560
Cade, W. H. (1975). Acoustically orienting parasitoids: fly phonotaxis to cricket song. Science 190, 1312–1313. doi: 10.1126/science.190.4221.1312
Cade, W. H. (1981). Field cricket spacing, and the phonotaxis of crickets and parasitoid flies to clumped and isolated cricket songs. Zeitschrift Tierpsychol. 55, 365–375. doi: 10.1111/j.1439-0310.1981.tb01278.x
Cade, W. H., and Cade, E. S. (1992). Male mating success, calling and searching behaviour at high and low densities in the field cricket, Gryllus integer. Anim. Behav. 43, 49–56. doi: 10.1016/S0003-3472(05)80070-3
Clyne, J. D., and Miesenböck, G. (2008). Sex-specific control and tuning of the pattern generator for courtship song in Drosophila. Cell 133, 354–363. doi: 10.1016/j.cell.2008.01.050
Cocroft, R. B. (1999). Offspring-parent communication in a subsocial treehopper (Hemiptera: Membracidae: Umbonia crassicornis). Behaviour 136, 1–21. doi: 10.1163/156853999500640
Copeland, J., and Moiseff, A. (2010). Firefly synchrony: a behavioral strategy to minimize visual clutter. Science 329, 181. doi: 10.1126/science.1190421
Cranford, J. L. (1982). Localization of paired sound sources in cats: effects of variable arrival times. J. Acoust. Soc. Am. 72, 1309–1311. doi: 10.1121/1.388409
Darwin, C. (1871). The Descent of Man, and Selection in Relation to Sex, 1st Edn. London: John Murray.
Dawkins, M. S., and Guilford, T. (1996). Sensory bias and the adaptiveness of female choice. Am. Nat. 148, 937–942. doi: 10.1086/285964
Dawson, J. W., and Fullard, J. H. (1995). The neuroethology of sound production in tiger moths (Lepidoptera, Arctiidae). II. Location of the tymbal central pattern generator in Cycnia tenera Hubner. J. Comp. Physiol. A 176, 541–549. doi: 10.1007/BF00196419
Deily, J. A., and Schul, J. (2009). Selective phonotaxis in Neoconocephalus nebrascensis (Orthoptera: Tettigoniidae): call recognition at two temporal scales. J. Comp. Physiol. A 195, 31–37. doi: 10.1007/s00359-008-0379-2
Dent, M. L., and Dooling, R. J. (2004). The precedence effect in three species of birds (Melopsittacus undulatus, Serinus canaria and Taeniopygia guttata). J. Comp. Psychol. 118, 325–331. doi: 10.1037/0735-7036.118.3.325
Doherty, J. A., and Callos, J. D. (1991). Acoustic communication in the trilling field cricket, Gryllus rubens (Orthoptera: Gryllidae). J. Insect Behav. 4, 67–82. doi: 10.1007/BF01092552
Doolan, J. M., and Mac Nally, R. C. (1981). Spatial dynamics and breeding ecology in the cicada Cystosoma saundersii: the interaction between distributions of resources and intraspecific behaviour. J. Anim. Ecol. 50, 925–940. doi: 10.2307/4147
Dumont, J. P., and Robertson, R. M. (1986). Neuronal circuits: an evolutionary perspective. Science 233, 849–853. doi: 10.1126/science.233.4766.849
Endler, J. A., and McLellan, T. (1988). The process of evolution: towards a newer synthesis. Ann. Rev. Ecol. Syst. 19, 395–421. doi: 10.1146/annurev.es.19.110188.002143
Ermentrout, G. B. (1991). An adaptive model for synchrony in the firefly Pteroptyx malaccae. J. Math. Biol. 29, 571–585. doi: 10.1007/BF00164052
Fertschai, I., Stradner, J., and Römer, H. (2007). Neuroethology of female preference in the synchronously singing bushcricket Mecopoda elongata (Tettigoniidae; Orthoptera): why do followers call at all? J. Exp. Biol. 210, 465–476. doi: 10.1242/jeb.02655
Galliart, P. L., and Shaw, K. C. (1996). The effect of variation in parameters of the male calling song of the katydid, Amblycorypha parvipennis (Orthoptera: Tettigoniidae), on female phonotaxis and phonoresponse. J. Insect Behav. 9, 841–855. doi: 10.1007/BF02208973
Gerhardt, V. H. C., and Huber, F. (2002). Acoustic Communication in Insects and Anurans: Common Problems and Diverse Solutions. Chicago; London: The University of Chicago Press.
Grafe, T. U. (1996). The function of call alternation in the African reed frog (Hyperolius marmoratus): precise call timing prevents auditory masking. Behav. Ecol. Sociobiol. 38, 149–158. doi: 10.1007/s002650050227
Gramoll, S., and Elsner, N. (1987). Morphology of local “stridulation” interneurons in the metathoracic ganglion of the acridid grasshopper Omocestus viridulus L. J. Comp. Neurol. 263, 593–606. doi: 10.1002/cne.902630410
Greenfield, M. D. (1990). “Evolution of acoustic communication in the genus Neoconocephalus: discontinuous songs, synchrony, and interspecific interactions,” in The Tettigoniidae: Biology, Systematics and Evolution, eds W. J. Bailey, and D. C. F. Rentz (Berlin: Springer), 71–97.
Greenfield, M. D. (1994a). Cooperation and conflict in the evolution of signal interactions. Ann. Rev. Ecol. Syst. 25, 97–126. doi: 10.1146/annurev.es.25.110194.000525
Greenfield, M. D. (1994b). Synchronous and alternating choruses in insects and anurans: common mechanisms and diverse functions. Am. Zool. 34, 605–615. doi: 10.1093/icb/34.6.605
Greenfield, M. D. (1997). “Acoustic communication in Orthoptera,” in The Bionomics of Grasshoppers, eds S. K. Gangwere, M. C., Muralirangan, and M. Muralirangan (Wallingford: CAB International), 197–230.
Greenfield, M. D. (2015). Signal interactions and interference in insect choruses: Singing and listening in the social environment. J. Comp. Physiol. A 201, 143–154. doi: 10.1007/s00359-014-0938-7
Greenfield, M. D., and Roizen, I. (1993). Katydid synchronous chorusing is an evolutionarily stable outcome of female choice. Nature 364, 618–620. doi: 10.1038/364618a0
Greenfield, M. D., and Schul, J. (2008). Mechanisms and evolution of synchronous chorusing: emergent properties and adaptive functions in Neoconocephalus katydids (Orthoptera: Tettigoniidae). J. Comp. Physiol. A 122, 289–297. doi: 10.1037/0735-7036.122.3.289
Greenfield, M. D., and Snedden, W. A. (2003). Selective attention and the spatiotemporal structure of orthopteran choruses. Behaviour 140, 1–26. doi: 10.1163/156853903763999863
Greenfield, M. D., Tourtellot, M. K., and Snedden, W. A. (1997). Precedence effects and the evolution of chorusing. Proc. Biol. Sci. 264, 1355–1361. doi: 10.1098/rspb.1997.0188
Grobe, B., Rothbart, M. M., Hanschke, A., and Hennig, R. M. (2012). Auditory processing at two time scales by the cricket Gryllus bimaculatus. J. Exp. Biol. 215, 1681–1690. doi: 10.1242/jeb.065466
Guilford, T., and Dawkins, M. S. (1991). Receiver psychology and the evolution of animal signals. Anim. Behav. 42, 1–14. doi: 10.1016/S0003-3472(05)80600-1
Hartbauer, M. (2008). Chorus model of the synchronizing bushcricket species Mecopoda elongata. Ecol. Model. 213, 105–118. doi: 10.1016/j.ecolmodel.2007.11.010
Hartbauer, M., Haitzinger, L., Kainz, M., and Römer, H. (2014). Competition and cooperation in a synchronous bushcricket chorus. R. Soc. Open Sci. 1:140167. doi: 10.1098/rsos.140167
Hartbauer, M., Kratzer, S., and Römer, H. (2006). Chirp rate is independent of male condition in a synchronising bushcricket. J. Insect Physiol. 52, 221–230. doi: 10.1016/j.jinsphys.2005.10.006
Hartbauer, M., Kratzer, S., Steiner, K., and Römer, H. (2005). Mechanisms for synchrony and alternation in song interactions of the bushcricket Mecopoda elongata (Tettigoniidae: Orthoptera). J. Comp. Physiol. A 191, 175–188. doi: 10.1007/s00359-004-0586-4
Hartbauer, M., and Römer, H. (2007). A novel distributed swarm control strategy based on coupled signal oscillators. Bioinsp. Biomim. 2, 42–56. doi: 10.1088/1748-3182/2/3/002
Hartbauer, M., Siegert, M. E., Fertschai, I., and Römer, H. (2012b). Acoustic signal perception in a noisy habitat: lessons from synchronising insects. J. Comp. Physiol. A 198, 397–409. doi: 10.1007/s00359-012-0718-1
Hartbauer, M., Siegert, M. E., and Römer, H. (2015). Male age and female mate choice in a synchronizing katydid. J. Comp. Physiol. A 201, 763–772. doi: 10.1007/s00359-015-1012-9
Hartbauer, M., Stabentheiner, A., and Römer, H. (2012a). Signalling plasticity and energy saving in a tropical bushcricket. J. Comp. Physiol. A 198, 203–217. doi: 10.1007/s00359-011-0700-3
Hedwig, B. (1992). On the control of stridulation in the acridid grasshopper Omocestus viridulus L. I. Interneurons involved in rhythm generation and bilateral coordination. J. Comp. Physiol. A 171, 117–128. doi: 10.1007/BF00195967
Hedwig, B., and Pollack, G. S. (2008). “Invertebrate auditory pathways,” in The Senses: A Comprehensive Reference, eds A. I. Basbaum, A. Kaneko, G. M. Shepherd, and G. Westheimer (San Diego, CA: Academic Press), 525–564.
Heller, K. G., and von Helversen, D. (1986). Acoustic communication in phaneropterid bushcrickets: species-specific delay of female stridulatory response and matching male sensory time window. Behav. Ecol. Sociobiol. 18, 189–198. doi: 10.1007/BF00290822
Hennig, R. M. (2003). Acoustic feature extraction by cross-correlation in crickets? J. Comp. Physiol. A 189, 589–598. doi: 10.1007/s00359-003-0438-7
Hennig, R. M. (2009). Walking in Fourier's space: algorithms for the computation of periodicities in song patterns by the cricket Gryllus bimaculatus. J. Comp. Physiol. A 195, 971–987. doi: 10.1007/s00359-009-0473-0
Hennig, R. M., and Otte, D. (1996). Distributed control of song pattern generation in crickets revealed by lesions to the thoracic ganglia. Zoology 99, 268–276.
Hennig, R. M., and Weber, T. (1997). Filtering of temporal parameters of the calling song by cricket females of two closely related species: a behavioral analysis. J. Comp. Physiol. A 180, 621–630. doi: 10.1007/s003590050078
Hennig, R. M., Heller, K.-G., and Clemens, J. (2014). Time and timing in the acoustic recognition system of crickets. Front Physiol 5:286. doi: 10.3389/fphys.2014.00286
Hill, G. E. (1994). Geographic variation in male ornamentation and female preference in the house finch: a comparative test of models of sexual selection. Behav. Ecol. 5, 64–73. doi: 10.1093/beheco/5.1.64
Höbel, G. (2010). Interaction between signal timing and signal feature preferences: causes and implications for sexual selection. Anim. Behav. 79, 1257–1266. doi: 10.1016/j.anbehav.2010.02.026
Howard, R. D., and Palmer, J. G. (1995). Female choice in Bufo americanus: Effects of dominant frequency and call order. Copeia 1995, 212–217. doi: 10.2307/1446818
Johnstone, R. A. (1995). Sexual selection, honest advertisement and the handicap principle: reviewing the evidence. Biol. Rev. 70, 1–65. doi: 10.1111/j.1469-185X.1995.tb01439.x
Jones, M. D. R. (1963). Sound signals and alternation behaviour in Pholidoptera. Nature 4896, 928–929. doi: 10.1038/199928a0
Keuper, A., and Kühne, R. (1983). The acoustic behaviour of the bushcricket Tettigonia cantans II. Transmission of airborne-sound and vibration signals in the biotope. Behav. Proc. 8, 125–145. doi: 10.1016/0376-6357(83)90002-5
Kirkpatrick, M. (1987). “The evolutionary forces acting on female mating preferences in polygynous animals,” in Sexual Selection: Testing the Alternatives, eds J. W. Bradbury and M. B. Andersson (Chichester: Wiley), 67–82.
Kirkpatrick, M., and Ryan, M. J. (1991). The paradox of the lek and the evolution of mating preferences. Nature 350, 33–38. doi: 10.1038/350033a0
Klump, G. M., and Gerhardt, H. C. (1992). “Mechanisms and function of call-timing in male-male interactions in frogs,” in Playback and Studies of Animal Communication, ed P. K. McGregor (New York, NY: Plenum Press), 153–174.
Kokko, H. (1997). The lekking game: can female choice explain aggregated male displays? J. Theor. Biol. 187, 57–64.
Kostarakos, K., and Hedwig, B. (2012). Calling song recognition in female crickets: temporal tuning of identified brain neurons matches behavior. J. Neurosci. 32, 9601–9612. doi: 10.1523/JNEUROSCI.1170-12.2012
Kotiaho, J. S., Alatalo, R. V., Mappes, J., and Parri, S. (2004). Adaptive significance of synchronous chorusing in an acoustically signalling wolf spider. Proc. R. Soc. B 271, 1847–1850. doi: 10.1098/rspb.2004.2788
Lakes-Harlan, R., Stolting, H., and Moore, T. E. (2000). Phonotactic behaviour of a parasitoid fly (Emblemasoma auditrix, Diptera, Sarcophagidae) in response to the calling song of its host cicada (Okanagana rimosa, Homoptera, Cicadidae). Zoology 103, 31–39.
Latimer, W. (1981). The Acoustic Behaviour of Platycleis albopunctata (Goeze) (Orthoptera, Tettigoniidae). Behaviour 76, 182–206. doi: 10.1163/156853981X00077
Lee, N., Elias, D. O., and Mason, A. C. (2009). A precedence effect resolves phantom sound source illusions in the parasitoid fly Ormia ochracea. Proc. Natl. Acad. Sci. U.S.A. 106, 6357–6362. doi: 10.1073/pnas.0809886106
Lehmann, G. U. C. (2003). Review of biogeography, host range and evolution of acoustic hunting in ormiini (Insecta, Diptera, Tachinidae), Parasitoids of night-calling bushcrickets and crickets (Insecta, Orthoptera, Ensifera). Zool. Anz. 242, 107–120. doi: 10.1078/0044-5231-00091
Lehmann, G. U. C., and Heller, K. G. (1998). Bushcricket song structure and predation by the acoustically orienting parasitoid fly Therobia leonidei (Diptera: Tachinidae: Ormiini). Behav. Ecol. Sociobiol. 43, 239–245. doi: 10.1007/s002650050488
Litovsky, R. Y., Colburn, H. S., Yost, W. A., and Guzman, S. J. (1999). The precedence effect. J. Acoust. Soc. Am. 106, 1633–1654. doi: 10.1121/1.427914
Marshall, V. T., and Gerhardt, H. C. (2010). A precedence effect underlies preferences for calls with leading pulses in the grey treefrog, Hyla versicolor. Anim. Behav. 80, 139–145. doi: 10.1016/j.anbehav.2010.04.014
Maynard Smith, J. (1985). Sexual selection, handicaps and true fitness. J. Theor. Biol. 115, 1–8. doi: 10.1016/S0022-5193(85)80003-5
Maynard Smith, J. (1991). Honest signalling: the Philip Sidney game. Anim. Behav. 42, 1034–1035. doi: 10.1016/S0003-3472(05)80161-7
McLister, J. D. (2001). Physical factors affecting the cost and efficiency of sound production in the treefrog Hyla versicolor. J. Exp. Biol. 204, 69–80.
Meckenhäuser, G., Hennig, R. M., and Nawrot, M. P. (2013). Critical song features for auditory pattern recognition in crickets. PLoS ONE 8:e55349. doi: 10.1371/journal.pone.0055349
Meixner, A. J., and Shaw, K. C. (1986). Acoustic and associated behavior of the coneheaded katydid, Neoconocephalus nebrascensis (Orthoptera: Tettigoniidae). Ann. Entom. Soc. Am. 79, 554–565. doi: 10.1093/aesa/79.4.554
Merker, B., Madison, G., and Eckerdal, P. (2009). On the role and origin of isochrony in human rhythmic entrainment. Cortex 45, 4–17. doi: 10.1016/j.cortex.2008.06.011
Mhatre, N., Bhattacharya, M., Robert, D., and Balakrishnan, R. (2011). Matching sender and receiver: poikilothermy and frequency tuning in a tree cricket. J. Exp. Biol. 214, 2569–2578. doi: 10.1242/jeb.057612
Minckley, R. L., and Greenfield, M. D. (1995). Psychoacoustics of female phonotaxis and the evolution of male signal interactions in Orthoptera. Ethol. Ecol. Evol. 7, 235–243.
Morris, G. K., Kerr, G. E., and Gwynne, D. T. (1975). Calling song function in the bog katydid, Metrioptera sphagnorum (F. Walker) (Orthoptera, Tettigoniidae): female phonotaxis to normal and altered song. Zeitschrift Tierpsychol. 37, 502–514. doi: 10.1111/j.1439-0310.1975.tb00891.x
Morris, G. K., Kerr, G., and Fullard, J. H. (1978). Phonotactic preferences of female meadow katydids (Orthoptera: Tettigoniidae: Conocephalus nigropleurum). Can. J. Zool. 56, 1479–1487. doi: 10.1139/z78-205
Murphy, M. A., Thomspon, N. L., and Schul, J. (2016). Keeping up with the neighbour: a novel mechanism of call synchrony in Neoconocephalus ensiger katydids. J. Comp. Physiol. A. 202, 225–234. doi: 10.1007/s00359-016-1068-1
Nityananda, V., and Balakrishnan, R. (2007). Synchrony during acoustic interactions in the bushcricket Mecopoda ‘Chirper’ (Tettigoniidae: Orthoptera) is generated by a combination of chirp-by-chirp resetting and change in intrinsic chirp rate. J. Comp. Physiol. A 193, 51–65. doi: 10.1007/s00359-006-0170-1
Nityananda, V., and Balakrishnan, R. (2008). Leaders and followers in katydid choruses in the field: call intensity, spacing and consistency. Anim. Behav. 76, 723–735. doi: 10.1016/j.anbehav.2008.04.015
Nityananda, V., and Balakrishnan, R. (2009). Modeling the role of competition and cooperation in the evolution of katydid acoustic synchrony. Behav. Ecol. 20, 484–489. doi: 10.1093/beheco/arp022
Nityananda, V., Stradner, J., Balakrishnan, R., and Römer, H. (2007). Selective attention in a synchronising bushcricket: physiology, behaviour and ecology. J. Comp. Physiol. A 193, 983–991. doi: 10.1007/s00359-007-0251-9
Otte, D. (1977). “Communication in Orthoptera,” in How Animals Communicate, ed T. A. Sebeok (Bloomington: Indiana University Press), 334–361.
Otte, D., and Smiley, J. (1977). Synchrony in Texas fireflies with a consideration of male interaction models. Biol. Behav. 2, 143–158.
Papaj, D. R., and Lewis, A. C. (2012). Insect Learning: Ecology and Evolutionary Perspectives. Dordrecht: Springer Science & Business Media.
Party, V., Brunel-Pons, O., and Greenfield, M. D. (2014). Priority of precedence: receiver psychology, female preference for leading calls and sexual selection in insect choruses. Anim. Behav. 87, 175–185. doi: 10.1016/j.anbehav.2013.10.029
Party, V., Streiff, R., Marin-Cudraz, T., and Greenfield, M. D. (2015). Group synchrony and alternation as an emergent property: elaborate chorus structure in a bushcricket is an incidental by-product of female preference for leading calls. Behav. Ecol. Sociobiol. 69, 1957–1973. doi: 10.1007/s00265-015-2008-8
Popov, A. V., and Shuvalov, V. F. (1977). Phonotactic behavior of crickets. J. Comp. Physiol. A 119, 111–126. doi: 10.1007/BF00655876
Poulet, J. F. A., and Hedwig, B. (2005). Auditory orientation in crickets: Pattern recognition controls reactive steering. Proc. Natl. Acad. Sci. U.S.A. 102, 15665–15669. doi: 10.1073/pnas.0505282102
Prestwich, K. N. (1994). The energetics of acoustic signaling in anurans and insects. Am. Zool. 34, 625–643. doi: 10.1093/icb/34.6.625
Reinhold, K., Greenfield, M. D., Jang, Y., and Broce, A. (1998). Energetic cost of sexual attractiveness: ultrasonic advertisement in wax moths. Anim. Behav. 55, 905–913. doi: 10.1006/anbe.1997.0594
Repp, B. H. (2001). Processes underlying adaptations to tempo changes in sensorimotor synchronization. Hum. Mov. Sci. 20, 177–312. doi: 10.1016/S0167-9457(01)00049-5
Repp, B. H. (2005). Sensorimotor synchronization: a review of the tapping literature. Psychol. Bull. Rev. 12, 969–992. doi: 10.3758/BF03206433
Rheinlaender, J., and Römer, H. (1980). Bilateral coding of sound direction in the CNS of the bushcricket Tettigonia viridissima L. (Orthoptera, Tettigoniidae). J. Comp. Physiol. A 140, 101–111. doi: 10.1007/BF00606302
Richardson, C., Lena, J.-P., Joly, P., and Lengagne, T. (2008). Are leaders good mates? A study of call timing and male quality in a chorus situation. Anim. Behav. 76, 1487–1495. doi: 10.1016/j.anbehav.2008.06.019
Robertson, R. M., Pearson, K. G., and Reichert, H. (1982). Flight interneurons in the locust and the origin of insect wings. Science 217, 177–179. doi: 10.1126/science.217.4555.177
Robinson, D. J., and Hall, M. J. (2002). Sound signalling in Orthoptera. Adv. Insect Physiol. 29, 151–278. doi: 10.1016/S0065-2806(02)29003-7
Römer, H., and Krusch, M. (2000). A gain-control mechanism for processing of chorus sounds in the afferent auditory pathway of the bushcricket Tettigonia viridissima (Orthoptera; Tettigoniidae). J. Comp. Physiol. A 186, 181–191. doi: 10.1007/s003590050018
Römer, H., Hedwig, B., and Ott, S. R. (2002). Contralateral inhibition as a sensory bias: the neural basis for a female preference in a synchronously calling bushcricket, Mecopoda elongata. Eur. J. Neurosci. 15, 1655–1662. doi: 10.1046/j.1460-9568.2002.02003.x
Ryan, M. J. (1990). Sexual selection, sensory systems, and sensory exploitation. Oxf. Surv. Evol. Biol. 7, 157–195.
Ryan, M. J. (1999). Sexual selection and sensory exploitation. Science 283, 1083. doi: 10.1126/science.283.5405.1083a
Ryan, M. J., and Keddy-Hector, A. C. (1992). Directional patterns of female mate choice and the role of sensory biases. Am. Nat. 139, 4–35. doi: 10.1086/285303
Ryan, M. J., and Rand, A. S. (1990). The sensory basis of sexual selection for complex calls in the Túngara frog, Physalaemus pustulosus (sexual selection for sensory exploitation). Evolution 44, 305–314. doi: 10.2307/2409409
Ryan, M. J., and Rand, A. S. (1993). Sexual selection and signal evolution: the ghost of biases past. Philos. Trans. R. Soc. Lond. B 340, 187–195. doi: 10.1098/rstb.1993.0057
Ryan, M. J., Fox, J. H., Wilczynski, W., and Rand, A. S. (1990). Sexual selection for sensory exploitation in the frog Physalaemus pustulosus. Nature 343, 66–69. doi: 10.1038/343066a0
Schmidt, A. K. D., and Balakrishnan, R. (2015). Ecology of acoustic signalling and the problem of masking interference in insects. J. Comp. Physiol. A 201, 133–142. doi: 10.1007/s00359-014-0955-6
Schmidt, A. K. D., and Römer, H. (2011). Solutions to the cocktail party problem in insects: selective filters, spatial release from masking and gain control in tropical crickets. PLoS ONE 6:e28593. doi: 10.1371/journal.pone.0028593
Schmidt, A. K. D., Riede, K., and Römer, H. (2011). High background noise shapes selective auditory filters in a tropical cricket. J. Exp. Biol. 214, 1754–1762. doi: 10.1242/jeb.053819
Schmidt, A. K. D., Römer, H., and Riede, K. (2013). Spectral niche segregation and community organization in a tropical cricket assemblage. Behav. Ecol. 24, 470–480. doi: 10.1093/beheco/ars187
Schöneich, S., and Hedwig, B. (2011). Neural basis of singing in crickets: Central pattern generation in abdominal ganglia. Naturwissenschaften 98, 1069–1073. doi: 10.1007/s00114-011-0857-1
Schöneich, S., and Hedwig, B. (2012). Cellular basis for singing motor pattern generation in the field cricket (Gryllus bimaculatus DeGeer). Brain Behav. 2, 707–725. doi: 10.1002/brb3.89
Schöneich, S., Kostarakos, K., and Hedwig, B. (2015). An auditory feature detection circuit for sound pattern recognition. Sci. Adv. 1:e1500325. doi: 10.1126/sciadv.1500325
Schul, J., Bush, S. L., and Frederick, K. H. (2014). “Evolution of call patterns and pattern recognition mechanisms in Neoconocephalus katydids,” in Insect Hearing and Acoustic Communication, Animal Signals and Communication, ed B. Hedwig (Heidelberg: Springer), 167–183.
Schütze, H, Elsner, N. (2001). Stridulatory pattern generation in acridid grasshoppers: metathoracic interneurons in Stenobothrus rubicundus (Germar 1817). J. Comp. Physiol. A. 187, 529–540. doi: 10.1007/s003590100225
Schwartz, J. J. (1994). Male advertisement and female choice in frogs: Recent findings and new approaches to the study of communication in a dynamic acoustic environment. Am. Zool. 34, 616–624. doi: 10.1093/icb/34.6.616
Semjen, A., Vorberg, D., and Schulze, H.-H. (1998). Getting synchronized with the metronome: Comparisons between phase and period correction. Psychol. Res. 61, 44–55. doi: 10.1007/s004260050012
Shaw, K. C., Galliart, P. L., and Smith, B. (1990). Acoustic Behavior of Amblycorypha parvipennis (Orthoptera: Tettigoniidae). Ann. Entom. Soc. Am. 83, 617–625. doi: 10.1093/aesa/83.3.617
Shelly, T. E., and Greenfield, M. D. (1991). Dominions and desert clickers (Orthoptera: Acrididae): Influences of resources and male signaling on female settlement patterns. Behav. Ecol. Sociobiol. 28, 133–140. doi: 10.1007/BF00180990
Siegert, M. E., Römer, H., Hashim, R., and Hartbauer, M. (2011). Neuronal correlates of a preference for leading signals in the synchronizing bushcricket Mecopoda elongata (Orthoptera, Tettigoniidae). J. Exp. Biol. 214, 3924–3934. doi: 10.1242/jeb.057901
Siemers, B. M., and Güttinger, R. (2006). Prey conspicuousness can explain apparent prey selectivity. Curr. Biol. 16, R157–R159. doi: 10.1016/j.cub.2006.02.056
Simmons, L. W. (1991). Female choice and the relatedness of mates in the field cricket, Gryllus bimaculatus. Anim. Behav. 41, 493–501. doi: 10.1016/S0003-3472(05)80852-8
Sismondo, E. (1990). Synchronous, alternating, and phase-locked stridulation by a tropical katydid. Science 249, 55–58. doi: 10.1126/science.249.4964.55
Snedden, W. A., and Greenfield, M. D. (1998). Females prefer leading males: relative call timing and sexual selection in katydid choruses. Anim. Behav. 56, 1091–1098. doi: 10.1006/anbe.1998.0871
Stumpner, A., and Ronacher, B. (1994). Neurophysiological aspects of song pattern recognition and sound localization in grasshoppers. Am. Zool. 34, 696–705. doi: 10.1093/icb/34.6.696
Stumpner, A., Ronacher, B., and Helversen, O. V. (1991). Auditory interneurones in the metathoracic ganglion of the grasshopper Chorthippus biguttulus: II. Processing of temporal patterns of the song of the male. J. Exp. Biol. 158, 411–430.
Tauber, E., Cohen, D., Greenfield, M. D., and Pener, M. P. (2001). Duet singing and female choice in the bushcricket Phaneroptera nana. Behaviour 138, 411–430. doi: 10.1163/156853901750382089
ter Hofstede, H. M., Schöneich, S., Robillard, T., and Hedwig, B. (2015). Evolution of a communication system by sensory exploitation of startle behavior. Curr. Biol. 25, 3245–3252. doi: 10.1016/j.cub.2015.10.064
Tuttle, M. D., and Ryan, M. J. (1982). The role of synchronized calling, ambient light, and ambient noise, in anti-bat-predator behavior of a treefrog. Behav. Ecol. Sociobiol. 11, 125–131. doi: 10.1007/BF00300101
von Helversen, D. (1972). Gesang des Männchens und Lautschema des Weibchens bei der Feldheuschrecke Chorthippus biguttulus (Orthoptera, Acrididae). J. Comp. Physiol. A 81, 381–422. doi: 10.1007/BF00697757
von Helversen, D., and von Helversen, O. (1975). Verhaltensgenetische Untersuchungen am akustischen Kommunikationssystem der Feldheuschrecken (Orthoptera, Acridiae). I. Der Gesang von Artbastarden zwischen Chorthippus biguttulus und Ch. mollis. J. Comp. Physiol. A 104, 273–299. doi: 10.1007/BF01379053
von Helversen, D., and von Helversen, O. (1998). Acoustic pattern recognition in a grasshopper: processing in the time or frequency domain? Biol. Cybern. 79, 467–476. doi: 10.1007/s004220050496
von Philipsborn, A. C., Liu, T., Yu, J. Y., Masser, C., Bidaye, S. S., and Dickson, B. J. (2011). Neuronal control of drosophila courtship song. Neuron 69, 509–522. doi: 10.1016/j.neuron.2011.01.011
Walker, T. J. (1957). Specificity in the response of female tree crickets (Orthoptera, Gryllidae, Oecanthinae) to calling songs of the males. Anal. Entomol. Soc. Am. 50, 626–636. doi: 10.1093/aesa/50.6.626
Walker, T. J. (1969). Acoustic Synchrony: two Mechanisms in the Snowy Tree Cricket. Science 166, 891–894. doi: 10.1126/science.166.3907.891
Walker, T. J. (1983). “Diel patterns of calling in nocturnal Orthoptera,” in Orthopteran Mating Systems: Sexual Competition in a Diverse Group of Insects, eds D. T. Gwynne and G. K. Morris (Boulder, CO: Westview), 45–72.
West-Eberhard, M. J. (1979). Sexual selection, social competition, and evolution. Proc. Am. Philos. Soc. 123, 222–234.
Wiley, R. H. (1991). Lekking in birds and mammals: behavioral and evolutionary issues. Adv. Stat. Behav. 20, 201–291. doi: 10.1016/S0065-3454(08)60322-8
Wyttenbach, R., and Hoy, R. (1993). Demonstration of the precedence effect in an insect. J. Acoust. Soc. Am. 94, 777–784. doi: 10.1121/1.408207
Zahavi, A. (1975). Mate selection - a selection for a handicap. J. Theor. Biol. 53, 205–214. doi: 10.1016/0022-5193(75)90111-3
Zimmermann, U., Rheinlaender, J., and Robinson, D. (1989). Cues for male phonotaxis in the duetting bushcricket Leptophyes punctatissima. J. Comp. Physiol. A 164, 621–628. doi: 10.1007/BF00614504
Zuk, M., and Kolluru, G. R. (1998). Exploitation of sexual signals by predators and parasitoids. Q. Rev. Biol. 73, 415–438. doi: 10.1086/420412
Zuk, M., Rotenberry, J. T., and Simmons, L. W. (1998). Calling songs of field crickets (Teleogryllus oceanicus) with and without phonotactic parasitoid infection. Evolution 52, 166–171. doi: 10.2307/2410931
Keywords: insect choruses, chorus synchrony, female choice, rhythm generation, pattern recognition, cooperation
Citation: Hartbauer M and Römer H (2016) Rhythm Generation and Rhythm Perception in Insects: The Evolution of Synchronous Choruses. Front. Neurosci. 10:223. doi: 10.3389/fnins.2016.00223
Received: 04 February 2016; Accepted: 06 May 2016;
Published: 31 May 2016.
Edited by:
Andrea Ravignani, Vrije Universiteit Brussel, BelgiumReviewed by:
Bjorn Hellmut Merker, Formerly affiliated with Mid Sweden University, SwedenMichael Greenfield, Université François Rabelais Tours, France
Copyright © 2016 Hartbauer and Römer. This is an open-access article distributed under the terms of the Creative Commons Attribution License (CC BY). The use, distribution or reproduction in other forums is permitted, provided the original author(s) or licensor are credited and that the original publication in this journal is cited, in accordance with accepted academic practice. No use, distribution or reproduction is permitted which does not comply with these terms.
*Correspondence: Manfred Hartbauer, manfred.hartbauer@uni-graz.at