Reversible inactivation of rat premotor cortex impairs temporal preparation, but not inhibitory control, during simple reaction-time performance
- 1 The John B. Pierce Laboratory, New Haven, CT, USA
- 2 Interdepartmental Neuroscience Program, Yale University School of Medicine, New Haven, CT, USA
- 3 Yale College, Yale University, New Haven, CT, USA
- 4 Department of Neurobiology, Yale University School of Medicine, New Haven, CT, USA
Previous studies by our lab and others have established a role for medial areas of the prefrontal cortex (mPFC) in the top–down control of action during simple reaction-time (RT) tasks. However, the neural circuits that allow mPFC to influence activity in the motor system have remained unclear. In the present study, we used a combination of tract-tracing and reversible inactivation methods to examine the role of a motor-related area in the rat frontal cortex, called the rostral forelimb area (RFA), in the top–down control of action. Neural tracing studies involved used electrical microstimulation to identify RFA and injections of biotinylated dextran amines (BDA) to map out connections of RFA with other parts of the frontal cortex. Connections were found between RFA and mPFC, the agranular insular cortex, and the primary motor cortex. Reversible inactivations using muscimol infusions into RFA increased response times and eliminated delay-dependent speeding, but did not increase premature responding. These results are markedly different from what is obtained when muscimol is infused into mPFC, which leads to excessive premature responding and a reduction of RTs to stimuli at short delays (Narayanan et al., 2006). We also tested animals during the RT task after inactivating the agranular insular cortex, which contains neurons that projects to and receives from RFA and mPFC, and found no effects on RT performance. Together, these studies suggest that RFA is a premotor region in the rat frontal cortex that competes with mPFC to control action selection. We suggest that RFA controls the threshold that is used to initiate responding and generates prepotent excitation over responding that is crucial for temporal preparation.
Introduction
Simple reaction-time (RT) tasks require subjects to sustain a behavioral response (e.g., lever press) over a delay period and then to respond quickly to a stimulus, presented at the end of the delay, by terminating the sustained response. RT performance depends on processing in the medial prefrontal cortex (mPFC) in human beings (Stuss et al., 2005) and rodents (Passetti et al., 2002; Risterucci et al., 2003). Using the muscimol method for reversible inactivation, Narayanan et al. (2006) found that mPFC processing has inhibitory effects on RT performance: Rats made more premature responses with mPFC inactivated and also responded faster to stimuli presented after a brief delay period (when rats and humans are normally slow to respond). Subsequent single-unit recording studies (Narayanan and Laubach, 2006, 2009) found mPFC neurons with persistent activity during the delay period, similar to findings in the primate mPFC by Niki and Watanabe (1979). These neurons fire at altered rates in advance of premature errors (Narayanan and Laubach, 2006), similar to what is observed in the rat motor cortex (Laubach et al., 2000). In fact, if mPFC is inactivated with muscimol, forelimb-related motor cortical neurons show reduced levels of persistent spike activity during the delay period and fail to fire differentially in advance of premature responses (Narayanan and Laubach, 2006). These results suggest that mPFC has a role in the top–down control of action, specifically in limiting inappropriate responding during the delay period.
Anatomical tract-tracing studies have consistently reported weak connections between mPFC and the primary motor cortex (in the lateral agranular cortex) and a lack of convergent connections from mPFC and the motor cortex to subcortical motor areas such as the ventrolateral thalamus, red nucleus, and the ventral horn of the spinal cord (Sesack et al., 1989; Gabbott et al., 2003, 2005; Vertes, 2004). However, a recent study by Wang et al. (2008) reported projections from the anterior, but not the posterior, cingulate cortex from the orofacial and forelimb somatosensory and motor cortices. The rostral (or secondary) motor cortex, located in the medial agranular cortex has consistently been shown to have major connections with the caudal “primary” motor cortex (Reep et al., 1990; Wang and Kurata, 1998; Hoover and Vertes, 2007). This area may be a “medial wall” motor area (Picard and Strick, 1996) in rodents which has clear homologies to dorsomedial areas in primates (Preuss, 1995).
The rostral motor cortex is commonly referred to as the “rostral forelimb area” (RFA) (Donoghue and Wise, 1982; Neafsey and Sievert, 1982; Neafsey et al., 1986). This area contains neurons that lack responses to somatosensory and proprioceptive stimulation (Sievert and Neafsey, 1986) and that become active prior to changes in response force during isometric lever pressing and earlier than neurons in the primary motor cortex (Donoghue, 1985). During more complex multi-joint movements, such as reaching and grasping, RFA neurons are robustly active and not distinguishable from neurons in the primary motor cortex (Hyland, 1998). Unfortunately, there are no published studies on RFA neuronal activity during speeded performance tasks, such as the simple RT task. However, unilateral lesion studies have been done in the medial agranular cortex, and result in increased usage of the paw ipsilateral to the lesion and increased RTs to contralateral stimuli (Brown et al., 1991), spatial response biases in choice RT designs involving sustained head entries (Brown et al., 1991; Brasted et al., 2000), and disruptions of the execution of rewarded action sequences involving lever pressing (Ostlund et al., 2009). Importantly, there were no effects of damage in the medial agranular cortex on measures of inhibitory control, both in terms of premature and perseverative responding in the studies that used the choice RT designs (Brown et al., 1991; Brasted et al., 2000).
In the present study, we examined the role of RFA in a lever-release simple RT task using a combination of tract-tracing and reversible inactivation methods. The tract-tracing studies were done to determine if connections exist between RFA and the parts of mPFC where we have previously shown evidence for neural correlates of top–down control (e.g., Narayanan and Laubach, 2006). The reversible inactivation studies were done to determine the role of RFA in controlling speeded performance. As our study is the first to address the role of RFA in the control of speeded forelimb usage, we chose to constrain our predictions of potential deficits based on a conceptual model for top–down control (Figure 1A, based on Boulinguez et al., 2008; Jaffard et al., 2008; Narayanan and Laubach, 2009; Figure 1B, based on Narayanan et al., 2006). The model makes four distinct predictions about how inactivation of RFA may impair RT performance (Figure 1C). First, there could be a deficit in response initiation, due to elevated Threshold, which would lead to slowing of RTs across delays. Second, there could be a deficit in stimulus integration (or attention) which would lead to slowing at short, but not long delays. (At long delays, temporal effects of the decay of inhibition dominate the model, and so stimulus parameters, such as intensity or duration, should only have effects at short delays.) Third, there could be a deficit in prepotent excitation which would lead to slowing at longer delays. Fourth, there could be a deficit in proactive inhibition which would lead to speeding at short delays (as reported for mPFC by Narayanan et al., 2006). Using the muscimol method, we were able to test these predictions, and found that RFA has a distinct role compared to mPFC. Rather than serving for inhibitory control over inappropriate actions, RFA serves to enhance response speed as a function of delay duration (as the controller for prepotent excitation in our model) and thus has a role in the temporal preparation of the forthcoming response.
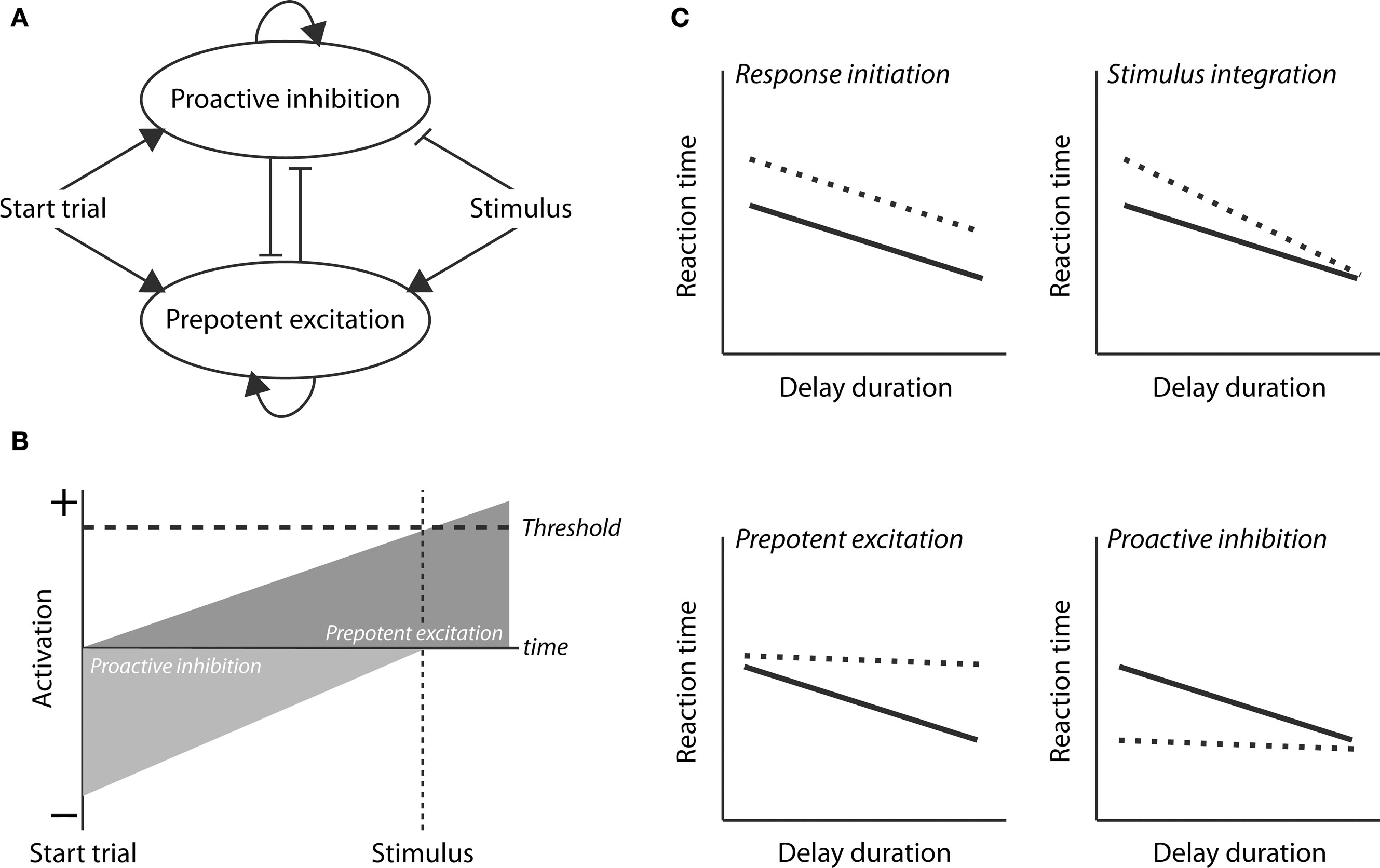
Figure 1. Conceptual model for control of simple RT performance. (A) A neural circuit model for top–down control of action (based on Jaffard et al., 2008 and Narayanan and Laubach, 2009). Two control processes are active during the delay period. Proactive inhibition serves to limit inappropriate responding until the stimulus is delivered. prepotent excitation serves to increase the level of activation in the motor system. The difference between these processes serves as an Activation function and responses are initiated if this function is greater than the threshold. (B) Time-course of the proactive inhibition and prepotent excitation signals during the delay period, as originally proposed in Narayanan et al. (2006). (C) Four distinct processes that underlie performance and could be impaired by inactivation of the RFA. A deficit in response initiation, due to elevated Threshold, would lead to slowing across delays. A deficit in stimulus integration (or attention) would lead to slowing at short, but not long delays. A deficit in prepotent excitation would lead to slowing at longer delays. A deficit in proactive inhibition would lead to speeding at short delays (as reported by Narayanan et al., 2006).
Materials and Methods
Experiment 1: Frontal Connections of the Rostral Forelimb Area
Subjects
Eight male Long–Evans rats (275–325 g) were purchased from Harlan, housed individually, and maintained with full access to food and water. All procedures were approved by the Animal Care and Use Committee at the John B. Pierce Laboratory and conformed to the standards of the U.S. National Institutes of Health Guide for the Care and Use of Laboratory Animals. All efforts were taken to minimize the number of animal subjects in this study and reduce pain and suffering.
Surgery
Anesthesia was initiated with isoflurane (4%) and maintained with intraperitoneal injections of either pentobarbital (50 mg/kg) or ketamine (100 mg/kg) and diazepam (10 mg/kg). A surgical level of anesthesia was maintained over the course of surgery with supplements (30 mg/kg) of ketamine every 45 min–1 h. Eyes were covered in ophthalmic antibiotic ointment to prevent desiccation. Under aseptic conditions, the scalp was disinfected with betadine, injected subcutaneously with lidocaine (0.2 ml) and retracted to expose the skull surface. The skull was leveled between bregma and lambda. Craniotomies were made bilaterally above the region known to contain RFA (Donoghue and Wise, 1982; Neafsey and Sievert, 1982; Neafsey et al., 1986; Wang and Kurata, 1998; Wang et al., 2008). A relatively anterior location (∼4 mm to bregma) was the focus of this study due to presence of vessels on the surface of the cortex at ∼3.5 mm anterior to bregma. A stainless-steel stimulating electrode (AM Systems, #564410; 250-μm thick with 1 mm tip exposure) was lowered into the brain and a unipolar 100 mV pulse was delivered using a stimulus-isolation unit with constant-current output (Grass SIU7) connected to a square-pulse stimulator (Grass S48). Microstimulation in the region of RFA evoked discrete movements of the forelimbs, whiskers, jaw, and tongue. Forelimb movements in the region of RFA involved complex “retraction” movements, in which one or both limbs moved up and then in the posterior direction. These movements were distinct from those found in the caudal (primary) motor cortex, where pilot studies found that microstimulation produced discrete flexion and extension movements of the contralateral forelimb or wrist.
Once the desired dorsoventral coordinates were determined (∼1–1.5 mm ventral to the cortical surface), 100 nl of BDA (Sigma-Aldrich; see Reiner et al., 2000 for review) was infused at sites that produced forelimb movements, and not whisker, jaw, or tongue movements, at a rate of 0.01 μl/min. BDA was prepared as 10% solution in dH2O and was loaded into a Hamilton syringe. The syringe was lowered to the desired coordinates at a rate of 0.2 mm/min. After injection, the Hamilton syringe was left in place for several minutes and then was raised at 0.2 mm/min until removed from the brain. The craniotomy was then sealed with cyanoacrylate (SloZap, Pacer Technologies) accelerated by Zip-Kicker (Pacer Technologies), and the wound margin closed with surgical staples. Rats were given carprofen intramuscularly (5 mg/kg) after surgery for analgesia, and allowed to recover in their home cages. Carprofen was administered in the home cage via drinking water at concentration of 50 μg/ml (5 mg/kg/day) for 48 h after surgery. Baytril (3 ml of 22.7 mg/ml, diluted in 500 ml of water) was also provided for 1 week.
Histology
One week after BDA infusion, rats were anesthetized with 100 mg/kg sodium pentobarbital and then transcardially perfused with chilled 4% paraformaldehyde and stored at 4°C. After removal, brains were post-fixed for an additional 1–2 days and then put into a solution with 20% w/v sucrose and 4% paraformaldehyde at 4°C for cryoprotection. Brains were sectioned at 50 μm on a freezing microtome. Alternating sections were stained for BDA (as described below) and Nissl material (using thionin). Sections stained for BDA were washed in three 5-min baths of PBS before being immersed in methanol solution containing 0.1% hydrogen peroxide. Sections were washed again in three 5-min baths of PBS before being incubated in streptavidin–biotin with 0.1% Triton-100 (Sigma-Aldrich) overnight at 4°C. After three 5-min washes of PBS, the reaction product was visualized by incubating the sections in 0.02% 3-3′-diaminobenzidine tetrahydrochloride (DAB) (Sigma-Aldrich) and 0.0009% hydrogen peroxide in dH2O with cobalt chloride and nickel salt intensification (Adams, 1981). In this process, nickel ammonium sulfate was added just to the DAB solution to a final concentration of 0.01% just prior to the addition of the hydrogen peroxide. Sections were incubated in this solution for 3 min. Sections were washed three times more in PBS, mounted onto gelatin-coated slides, dried, and coverslipped with Permount (Sigma-Aldrich).
The dataset analyzed in this manuscript was based on three brains. Five others were excluded for various reasons. One brain was overexposed to nickel and thus there was too much background staining. Two brains lacked transport of BDA beyond the injection site. Two brains lacked evidence of injection. In three brains, a fluorescence microscope (Motic PRM-L), connected to a Bioquant Image Analysis System, was used to identify brain regions with fibers and synaptic boutons and to take photomicrographs of the injection sites and other regions of interest. (Images were adjusted in Photoshop for use in Figure 2, based only on the “Auto Contrast” function).
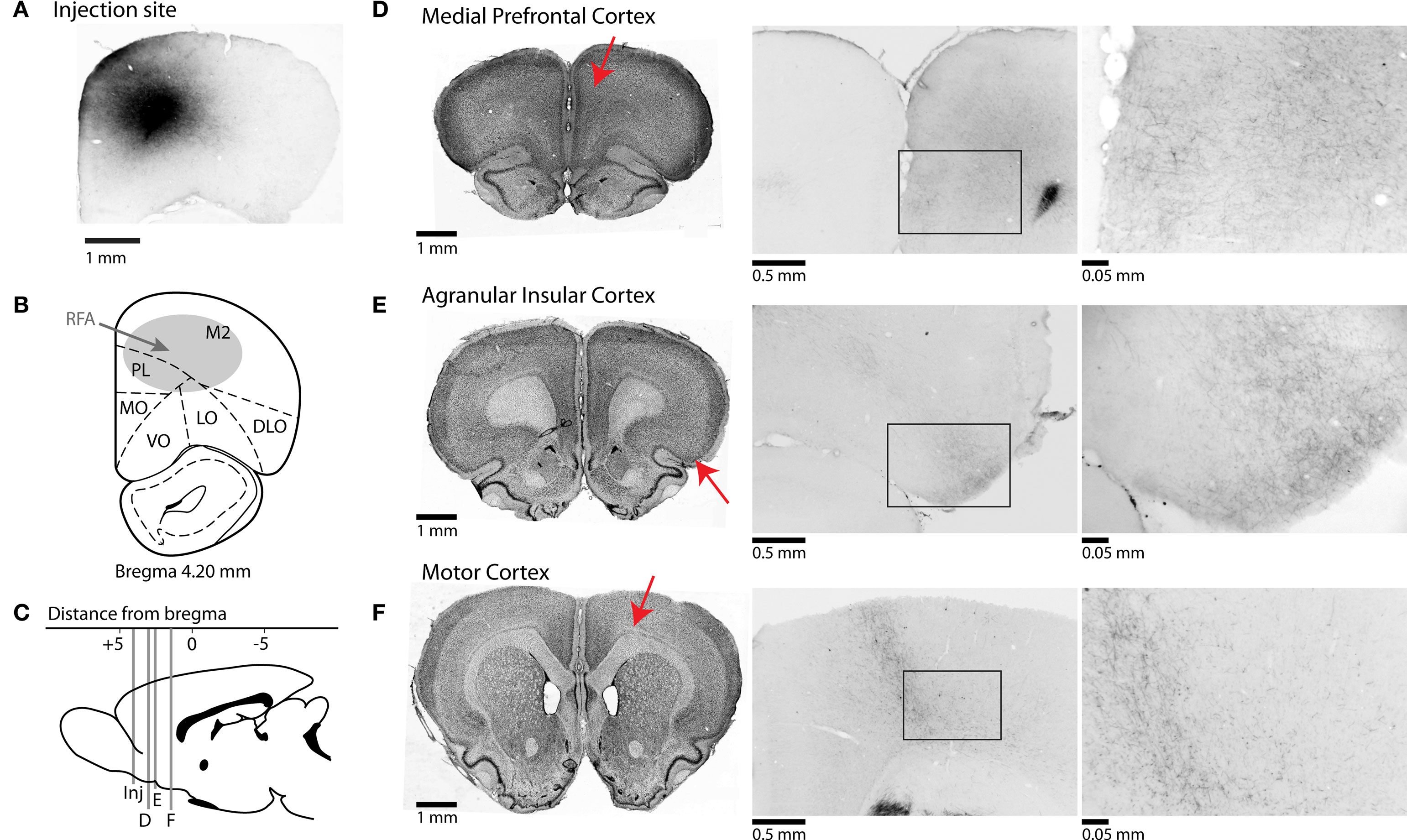
Figure 2. Afferent projections from RFA to frontal cortical regions. (A) Photomicrograph of injection site from one case stained for BDA. (B) Representative section showing approximate location of injections into the rostral forelimb area (RFA). (C) Mid-sagittal representative section showing AP-coordinates of projections of rostral forelimb area to multiple cortical areas. Red arrows indicate the approximate locations of the sections in the subsequent panels. (D–F) Corticocortical projections of rostral forelimb area. Nissl-stained sections shown on left represent coronal slices to which BDA-stained sections in middle and right sections correspond. Photomicrographs in center are taken at 4× magnification, and at right at 40× magnification. (D) RFA projects to areas of anterior cingulate cortex and prelimbic cortex, and fibers are seen in superficial layers of prelimbic at higher magnification. (E) Connections to agranular insular cortex are seen at high magnification across superficial and deeper layers of cortex. (F) Projections are found in deeper layers of primary motor cortex.
Experiment 2: Effects of Inactivation of the Rostral Forelimb Area on the Performance of a SRT Task with a Single Delay Period
Subjects
Eight male Long–Evans rats (275–325 g) were purchased from Harlan, housed individually, and maintained with full access to food and water for a period of at least 1 week after entry to the animal colony at the John B. Pierce Laboratory. Then, animals were motivated by food regulation while water was available ad libitum. Rats received 15–25 ml of a 20% sucrose solution during each behavioral session. Additional food (10 g standard lab chow) was provided 1–3 h after each behavioral session in the home cage. Rats were maintained at 85–90% free-food access body weight during the course of these experiments, and received one day of free access to food per week. All procedures were approved by the Animal Care and Use Committee at the John B. Pierce Laboratory and conformed to the standards of the U.S. National Institutes of Health Guide for the Care and Use of Laboratory Animals. All efforts were taken to minimize the number of animal subjects in this study and reduce subject pain and suffering.
Behavioral apparatus
Operant chambers (MedAssociates) were equipped with a lever, a drinking tube, and a speaker driven to produce an 8 kHz tone at 70 dBA, using audio equipment from Tucker-Davis Technologies that was controlled by custom-written scripts for the Data Acquisition Toolbox for Matlab (Mathworks). Each chamber also contained a house light, which was turned on at the beginning of each session, and a fan, which produced broadband noise at approximately 60 dBA. Behavioral arenas were housed in sound-attenuating chambers (MedAssociates). Twenty-percent sucrose solution was delivered via a syringe pump (MedAssociates) connected to a standard metal drinking tube (Ancare) via Tygon tubing. Each conditioned response in the task activated the pump at 0.04 ml/s for 1.5–3 s, depending on the stage of training. Behavior was monitored during all sessions via a closed circuit video camera, mounted in each behavioral arena, and was recorded to video tape in some sessions.
Behavioral training
Rats had regulated access to food in the home cage for 5 days, during which they were provided with 10 g of food at the time of day when behavioral training was subsequently carried out, until they had reached 85–90% free-feeding body weight. A conditioning session was then run in which an audible click stimulus (generated by a mechanical relay, Potter and Brumfield) was paired with activation of the pump, which was active for 3 s every 5–20 s over 50 min. Next, animals were shaped to depress the response lever (below the drinking tube in the first training session). Each press of the lever activated the pump for 1.5 s (volume per trial: 0.06 ml), during which time additional lever presses were not rewarded. The preferred paw for lever pressing was noted for each animal. In subsequent sessions, the lever was moved gradually to the direction of the side of the preferred paw and the pump was activated by the release of the lever instead of the press. Finally, the animals were trained to perform a “temporally conditioned” lever-press task, in which they were required to press on the lever over increasing periods of time (i.e., from 100 to 1000 ms in steps of 100 ms after every three consecutive correct responses) and to release the lever within 2 s in order to collect a liquid reward. Timeouts of 4–8 s occurred if the response duration was less than the target interval or longer than the target plus 2 s. All experimental events were terminated during the timeouts. Each session lasted 60 min and ended with all experimental stimuli being extinguished. Rats learned to make sustained lever presses on at least 60% of trials in 5–15 sessions.
Simple RT task with fixed delay period
Each trial in the simple RT task was initiated by the rat pressing the lever (Figure 3A). The period between trial initiation (lever press) and when the tone was presented was termed the delay period, and lasted 1.0 s. Correct responses occurred when the rats successfully maintained a lever press for the full delay period duration and released the lever with short response latencies (i.e., within a response window of 600 ms after the trigger stimulus). These responses were followed by sucrose solution rewards. Premature responses occurred when rats did not maintain a lever press for the full delay period (i.e., release before the presentation of the auditory stimulus). Late responses occurred when the lever was released after the response window. These latter two types of trials were followed by a time-out period, in which all experimental stimuli were terminated for 5–10 s. Lever pressing during the time-out period reset the timer controlling the time-out duration. After 3 days of steady performance (assessed by a runs test), rats were bilaterally implanted with cannulae.
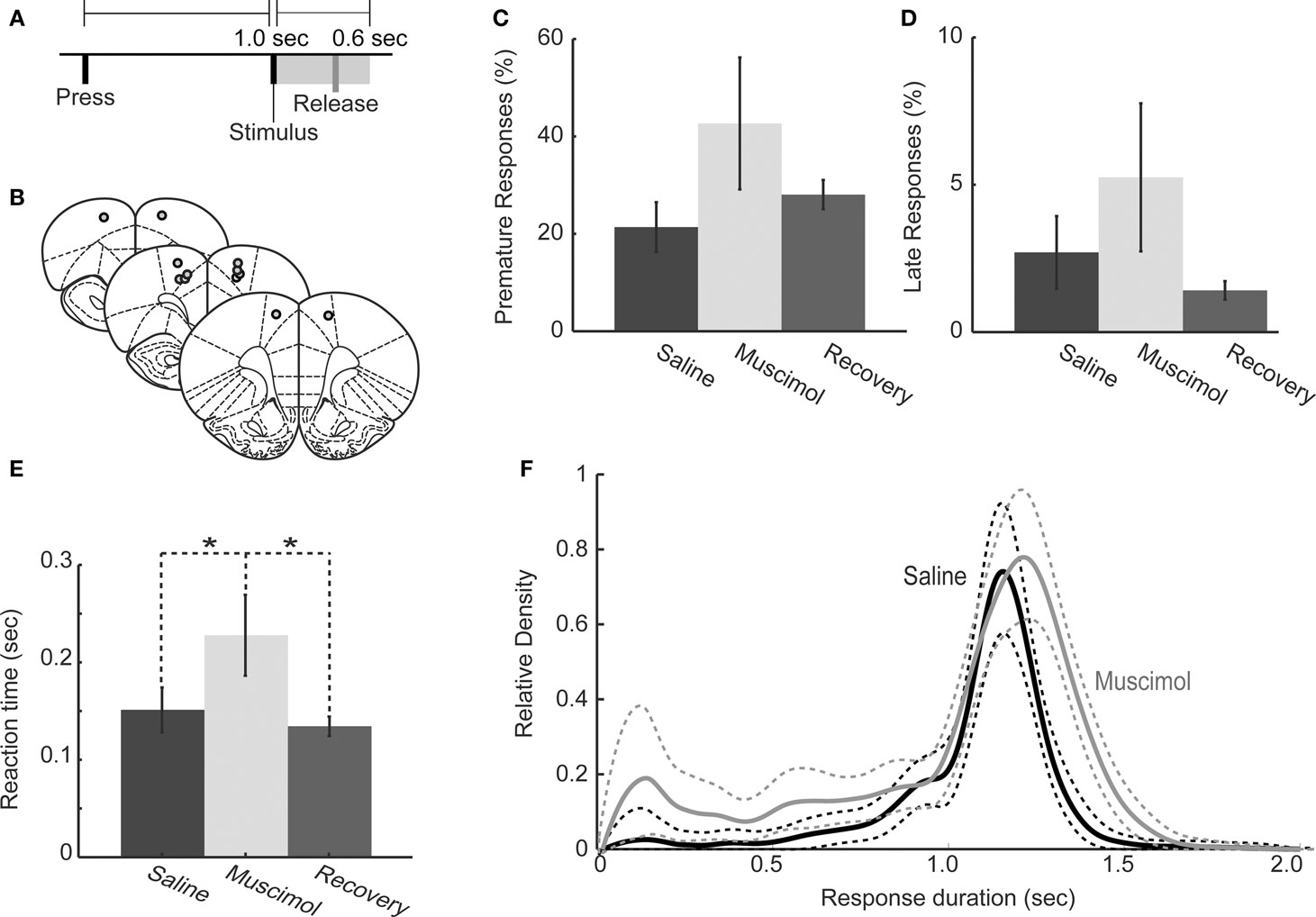
Figure 3. Behavioral performance in one-delay simple RT task following muscimol inactivation of RFA. (A) Task design: Rats pressed on a lever to initiate a trial and maintained the press until a tone was presented after a delay of 1.0 s. Lever releases within 600 ms of the tone were rewarded with 20% sucrose. Releases before the stimulus or more than 600 ms after the stimulus were punished by time-out. (B) Cannulae were implanted at locations that elicited discrete forelimb movements via intracranial microstimulation. Images were adapted from Paxinos and Watson (1998). (C,D) Rats (n = 6) with bilateral infusions of muscimol (0.5 μl of 1.0 μg/μl) did not show increased premature responding (C: P > 0.05 by rmANOVA) or late responding (D: P > 0.05 by rmANOVA). (E) Geometric mean RTs (error bars representing geometric absolute deviation) were significantly longer (paired t-test, P < 0.01) under muscimol than in saline and recovery sessions. (F) Distributions of response duration for saline and muscimol infusions showed that rats held down the lever for a longer period of time during RFA inactivation, i.e., there was a rightward shift in distribution (rmANOVA: P < 0.01).
Surgery
Anesthesia and microstimulation were performed in a similar manner to Experiment 1. Once the pulse elicited a rapid forepaw movement, the stereotaxic coordinates were recorded and used for placement of the cannulae. Twenty-six-gauge cannulae (Plastics One) with styletes cut flush to the cannulae tips were lowered slowly into bilateral RFA (coordinates from bregma: ∼ + 3.7 mm anteroposterior, ±0.5 mm mediolateral measured stereotaxically). After guide cannulae were placed, craniotomies were sealed with cyanoacrylate (SloZap, Pacer Technologies) accelerated by Zip-Kicker (Pacer Technologies), and methyl methacrylate (i.e., dental cement; AM Systems). Rats were given carprofen intramuscularly (5 mg/kg) after surgery for analgesia, and allowed to recover in their home cages. Caprofen was administered in the home cage via drinking water at concentration of 50 μg/ml (5 mg/kg/day) for 48 h after surgery. Baytril (3 ml of 22.7 mg/ml, diluted in 500 ml of water) was also provided for 1 week. Two rats died during surgery or during recovery from anesthesia, and six of the original group of eight rats were subsequently tested with muscimol infusions, as described below.
Drug infusion
After recovery from surgery (1 week), rats were food regulated and behavioral sessions were resumed. After demonstrating stable post-operative RT performance (3 days’ behavior at pre-operative levels), rats were acclimated to the infusion procedure by performing behavioral tasks 45 min after being lightly anesthetized with 4% isoflurane. Although there was some damage associated with placement of guide cannulae in RFA, there was no effect on subsequent behavior in the RT tasks. In order to reversibly inactivate brain regions, we used muscimol, a GABA-A agonist (Lomber, 1999; Martin and Ghez, 1999). Muscimol infusions were performed in the following manner: on the first day, rats were exposed to 4% isoflurane and received saline infusion into the cannulae (control session); on day 2 rats were exposed to isoflurane and received muscimol infusions into the cannulae (RFA inactivation sessions); on day 3 the rats were tested without any manipulation (recovery sessions). We observed no significant permanent changes in behavior due to infusions in the recovery sessions (see section Results). Infusions of muscimol were made with a 33-gauge injector (Plastics One) that protruded 0.2 mm from the tip of the guide cannula. Prior to injections, rats were lightly anesthetized using isoflurane (as above), injectors were inserted into the guide cannula, and 0.5 μl of infusion fluid was delivered per site at a rate of 15 μl/h (0.25 μl/min; Martin and Ghez, 1999) via a syringe infusion pump (KDS Scientific). The infusion fluid was sterile saline (0.9%) or muscimol (Sigma-Aldrich) at 1.0 μg/μl. Fluid was infused via 0.38 mm diameter polyethylene tubing joined to the injector on one end and to a 10-μl Hamilton syringe on the other end. Injections were confirmed by monitoring movement of fluid in the tubing via a small bubble. After injection was complete, the injector was left in place for 2 min to allow diffusion of fluid. Rats were then tested in the RT task 45 min after infusion. This amount of time was sufficient to allow for full recovery from the isoflurane anesthesia and for muscimol to reach its maximal effectiveness in inhibiting cortical activity, as well as to allow for stationary behavioral effects. Previous electrophysiological studies have found that the effects of muscimol begin almost immediately and are stable for many hours (Krupa et al., 1999) and that the infusions silence cortical activity near the site of the infusion (van Duuren et al., 2007). Rats were infused first in either right or left RFA (chosen randomly), and then infused in the contralateral location; consequently, the contralateral infusion was completed 4 min (2 min for infusion, and then 2 min to allow diffusion) after the initial infusion was complete. One week later, two rats were tested after unilateral infusions of muscimol (1.0 μg/μl), into the hemisphere contralateral to the paw used for lever pressing. These sessions were preceded by unilateral infusions of saline and were followed by recovery sessions.
Histology
Once experiments were complete, rats were anesthetized with 100 mg/kg sodium pentobarbital and transcardially perfused with 4% paraformaldehyde. Brains were sectioned on a freezing microtome, mounted on gelatin-subbed slides, and stained for Nissl material with thionin using standard methods.
Data analysis and statistics
Distributions of response latencies were heavily skewed. Therefore, we used the geometric mean (or “geomean,” i.e., the square root of the mean of squared response latencies) and the mean absolute deviation from the geometric mean (or “geomad,” i.e., the mean difference between each response latency and the geomean) to estimate the central tendencies of response latencies (Narayanan et al., 2006). To plot distributions of response times, kernel density estimation was used, as in Narayanan et al. (2006). We used the function ksdensity.m in the Matlab Statistics Toolbox. (see Silverman, 1986 for a review of kernel density methods and see Van Zandt, 2000 for how these methods can be applied to RT data.) The spread of these estimates was generated via bootstrapping (2000 iterations). Differences in distributions of response latencies were assessed using repeated-measures ANOVA in R. Significance values for the ANOVAs were carried out using a “permutation test” approach (Good, 2000). We pseudo-randomly permuted the independent variable of interest (e.g., saline or muscimol) 2000 times and computed F values for each permutation. An empirical probability of the results being obtained due to chance was computed as the ratio of the number of F values larger than that obtained in the actual data to the number of randomization data sets. To determine differences in behavioral measures such as percent premature and late responses in saline and muscimol sessions, Student’s t-tests with Bonferroni corrections were used (ttest.m in Matlab). If significant differences were found for RT distributions, Wilcoxon ranked-sum tests were used to determine whether the medians of distributions were significantly different.
Experiment 3: Effects of Inactivation of the Rostral Forelimb Area on the Performance of a SRT Task with Two-Delay Periods
This experiment used methods that were identical to Experiment 2, except that the task involved two-delay periods (as in Narayanan and Laubach, 2009) and used a different schedule of reinforcement (providing a higher concentration of sucrose for shorter RTs). Eight male Long–Evans rats (275–325 g) were purchased from Harlan and had regulated access to food identical to Experiment 2. Prior to behavioral training, rats were given free access to 32% sucrose for 1 day and 8% sucrose on another with the order of presentation counterbalanced across rats. We then administered a two-bottle preference test to determine the relative preference for each sucrose concentration compared with the other as well as with distilled water. Rats were presented with two bottles containing 50 ml of either 32% or 8% sucrose solution on 1 day, and 50 ml of 8% sucrose solution or distilled water on another. The order of these presentations was also counterbalanced across rats.
Rats were then trained to perform the simple RT task with a single delay period of 1.2 s identical to the training in Experiment 2. Once animals performed the task at an asymptotic level (no change in percentage of trials with lever presses sustained until the stimulus over three consecutive sessions), a second shorter (0.6 s) was introduced into the procedure. The delay duration for each trial was chosen pseudo-randomly and with an equal probability. In contrast to Experiment 1, reward delivery was contingent upon the speed of the lever release after presentation of the auditory stimulus. A custom-built multi-barrel spout (Instruments Shop at the Pierce Laboratory) was used to deliver the three fluids from a single location. The mode and the 90% percentile of the previous day’s RT distribution were calculated and used to determine the quality of reward delivered. For responses that were faster than the mode of the previous day’s RT distribution, the rats would receive 1.5 s of 32% sucrose solution. For responses that were slower than the mode and faster than the 90% percentile value of the previous day’s RT distribution, 1.5 s of 8% sucrose solution was delivered. If responses were slower than the 90% percentile of the previous day’s RT distribution, 1.5 s of distilled water was delivered.
Rats were implanted with cannulae in the region of RFA, as in Experiment 1. One rat died during surgery, and seven of the original group of eight rats were subsequently tested with muscimol infusions. Rats received infusions over a series of daily testing sessions of sterile saline, a relatively low dose of muscimol (0.1 μg/μl), and a higher dose (1.0 μg/μl) of muscimol (as in Experiment 2). Finally, they were tested in a recovery session.
Experiment 4: Effects of Inactivation of the Agranular Insular Cortex on the Performance of a SRT Task with a Single Delay Period
Three adult Long–Evans rats (275–325 g, Harlan) were trained to perform the RT task as described in Experiment 2 and using plain water rewards. After training, cannulae were implanted into the agranular insular cortex (Coordinates: 2.7 AP, 4 ML, 5 DV). Standard muscimol (0.1 and 1.0 μg/μl) was used for behavioral testing and extent of spread of drug was qualitatively assessed by infusing fluorescent muscimol (Invitrogen, as in Narayanan et al., 2006) 40 min prior to perfusion and brain removal. As the 1.0 μg/μl dose resulted in a total lack of behavior in at least one of the rats, the data reported here were based on a dose of 0.1 μg/μl.
Results
Experiment 1: Frontal Connections of the Rostral Forelimb Area
In this experiment, we used anterograde tract-tracing methods to determine if neurons in the region of the RFA project to frontal regions, such as mPFC, that are crucial for controlling RT performance. Unilateral injections of the selective anterograde tracer, biotinylated dextran amine (BDA), were successfully made into RFA in three rats (Figures 2A,B). Injection sites were made between + 3.6–4.2 mm AP. Injections spanned across cortical layers. Projections from RFA were determined by examining locations for the presence of fibers with synaptic boutons.
Injections into the region containing RFA resulted in anterograde projections to primary motor cortex, with terminals forming narrow “column like” bands across all cortical layers and with especially dense labeling in layers V/VI (Figure 2F). Projections were also found in posterior regions of the medial agranular cortex at + 0.5–1.0 mm AP from bregma (not shown), with terminals observed across layers (Figure 2F, center and right panels). Less dense labeling was observed in the caudal part of lateral agranular cortex (from 0 to −1 mm AP at 2.5–3.5 mm ML from bregma) and in the region of the forelimb somatosensory cortex (not shown).
All cases showed axons with synaptic terminals in the dorsal part of the mPFC, i.e., the prelimbic and rostral (pregenual) anterior cingulate regions, at all levels rostral to the genu of the corpus callosum and especially from + 2.8 to + 3.5 mm AP (Figure 2D). There was a decrease in axon density ventrally and caudally in the mPFC. Fibers were seen across most layers of rostral anterior cingulate cortex, the area bordering the medial agranular cortex; however, fibers were restricted to superficial layers of prelimbic cortex (Figure 2D, center and right panels). Fibers were sparse or not present in areas of infralimbic cortex (not shown).
Another major and distinct projection from RFA was to dorsal agranular insular cortex (Figure 2E). These projections covered the same area (in terms of the AP dimension) as the medial prefrontal projections and were most pronounced around + 2.5 to + 3.0 mm from bregma. Terminals were most apparent in superficial layers, similar to mPFC (Figure 2E, center and right panels).
Within the thalamus (not shown), the highest density of fibers and boutons were observed in the centromedian and ventroanterior nuclei. A lower density of fibers and boutons were found in the lateral mediodorsal, ventrolateral (VL), ventromedial (VM), and posterior nuclei, in accordance with previous studies investigating the thalamic connectivity to the medial agranular cortex (Reep and Corwin, 1999). This pattern of connections is typical for motor-related parts of the rodent cerebral cortex (Donoghue and Parham, 1983). The presence of fibers in both the VL and VM nuclei suggests that, while we did not observe discrete movements of the jaw, tongue, and whiskers at the sites where BDA was injected, the tracer diffused into adjacent excitable zones associated with these body regions. During microstimulation, we routinely noted that neighboring sites in the rostral motor field could activate largely distinct body regions, as has been reported previously (Neafsey, et al., 1986).
Experiment 2: Inactivation of Rostral Forelimb Area Slows Response Initiation
In this experiment, a simple RT task with a single delay period of 1 s (Figure 3A) was used to assess if inactivation of RFA impairs RT performance. Six rats were implanted bilaterally with cannulae in RFA (identified using electrical microstimulation, as in Experiment 1 above). The locations of cannula tips were confirmed in 50-μm sections of thionin-stained tissue (Figure 3B). Implantation of cannulae did not alter task performance. In pre-operative sessions, 23 ± 8.1% (mean ± standard error) of responses were premature (i.e., lever releases before the presentation of the stimulus) and 3.4 ± 1.3% were late responses (i.e., lever releases more than 600 ms after the presentation of the stimulus). In post-operative sessions, 25 ± 7.2% of trials were premature and 3.2 ± 1.3% were late, both unchanged from pre-operative sessions (t(1,5) = 0.355, P > 0.05 for premature responses; t(1,5) = −0.145, P < 0.89 for late responses). Also, the geometric mean of RTs was unchanged in pre- and post-operative sessions (pre-op 163 ± 36 ms, post-op = 160 ± 30 ms; paired t-test, t(1,5) = 0.324, P > 0.05).
In subsequent test sessions using muscimol (1.0 μg/μl), we observed no increase in premature responding (rmANOVA; F(1,5) = 0.060, P > 0.05; Figure 3C) and no increase in late responding (saline = 3.1 ± 1.2%, muscimol = 8.7 ± 2.6%: rmANOVA; F(1,5) = 2.12, P > 0.05, Figure 3D). Therefore, inactivation of RFA did not impair inhibitory control over action in the task. However, inactivation of RFA did result in significantly slower mean RTs (saline: 157 ± 25 ms, muscimol: 244 ± 42 ms; paired t-test, t(1,5) = −3.92, P = 0.0172, Figure 3E) and induced rightward shifts in the RT distributions (rmANOVA, F(1,2961) = 82.157, P ≪ 0.01; Figure 3F). Furthermore, these measures of task performance during muscimol test sessions was steady over the course of the session with accuracy (t(1,5) = −1.4350, P > 0.05), rate of responding (t(1,5) = −0.3022, P > 0.05), and geometric mean RT (t(1,5) = −0.9357, P > 0.05) not significantly different during the first and second halves of the test sessions.
Previous studies have shown that unilateral lesions in medial agranular cortex have a significant impact on behavior (Brown et al., 1991; Brasted et al., 2000). Therefore, we examined effects of unilateral inactivation of RFA by infusing the same dose of muscimol unilaterally into RFA in the hemisphere opposite to the paw used for lever pressing. While the mean RT was unaffected by the unilateral infusions of muscimol compared to saline (saline: 186 ± 9 ms, muscimol: 212 ± 10 ms, paired t-test, t(1,1) = −5.00, P = 0.12), the distributions of RTs were shifted significantly to the right (rmANOVA: F(1,804) = 16.707, P ≪ 0.01), as was found with bilateral inactivations.
Finally, we examined if other measures of task performance were affected by inactivation of RFA. Total number of trials did not differ significantly between saline and muscimol infusions (saline: 453.3 ± 22.8 trials, muscimol: 353.2 ± 33.3 trials; paired t-test: P = 0.155, t(1,5) = 1.6748), suggesting that the general motivation to perform the task was not changed by inactivation of RFA. We also investigated whether time-out responding, a generalized measure of inhibitory control, was affected by inactivation of RFA. Previous work from our lab has shown that inactivations of mPFC result in increased time-out responding (Narayanan et al., 2006). Infusions of muscimol did not cause a significant change in the number of responses per time-out session (saline: 0.61 ± 0.26 responses per time-out, muscimol: 1.19 ± 0.38 responses per time-out; paired t-test: P = 0.115, t(1,5) = −1.909), demonstrating that this measure of inhibitory control, like premature responding, was not significantly affected by RFA inactivation.
Experiment 3: Inactivation of Rostral Forelimb Area Eliminates Delay-Dependent Speeding
The previous experiment established that RTs are prolonged when RFA is inactivated. To determine if effects of RFA inactivation are sensitive to delay duration, rats were trained to perform a two-delay version of the task, with stimuli presented at either 0.6 or 1.2 s (Figure 4A). To ensure that rats responded as fast as possible at each delay, a special schedule of reinforcement was used in which a higher concentration of liquid sucrose reward (32%) was given if the RT was relatively short and lower concentrations of liquid sucrose (8% and 0%) were given after longer RTs (see section Materials and methods for details on this procedure). To ensure that rats were differentially motivated by the different levels of sucrose concentration, we performed two-bottle preference tests in the home cage prior to behavioral training. Rats strongly preferred 32–8% sucrose (32% sucrose solution consumed: 15.6 ± 1.1 ml, 8% sucrose solution consumed: 8.3 ± 0.9 ml; paired t-test: P < 0.01, t(1,6) = 3.926), and also strongly preferred 8% sucrose to deionized water (8% sucrose solution consumed: 26.4 ± 0.6 ml, deionized water consumed: 7.6 ± 1.0 ml; paired t-test: P ≪ 0.01, t(1,6) = 14.010).
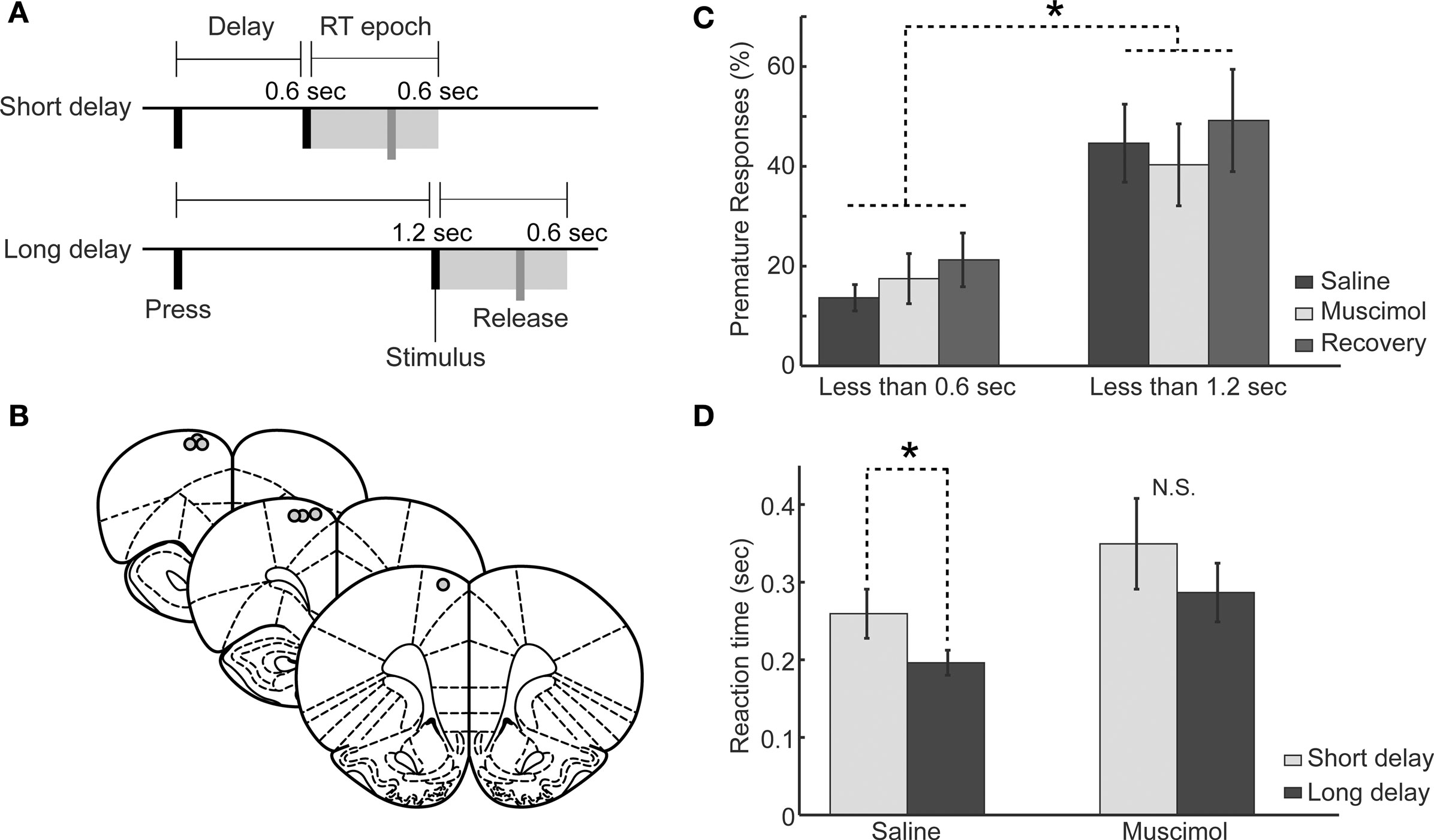
Figure 4. Behavioral performance in the two-delay version of the simple RT task following muscimol inactivation of RFA. (A) Task design: Rats pressed a lever down to initiate a trial and this initiated either a short (0.6 s) or long (1.2 s) delay period. See section “Materials and methods” for details. (B) Approximate locations of cannula tips at sites where intracranial microstimulation elicited discrete forelimb movements. Images adapted from Paxinos and Watson (1998). (C) Rats (n = 7) with unilateral infusions of muscimol (0.5 μl of 1.0 μg/μl into one hemisphere) did not make more premature responses at either the short or the long delays. There was increased premature responding at longer delays compared to shorter delays (rmANOVA: P < 0.01). (D) Geometric mean RTs (error bars representing geometric absolute deviation) were significantly longer at short compared to long delays under saline (*P < 0.05, paired t-test), but was no significant difference in RTs after the shorter and longer delays with muscimol in RFA (P > 0.05, paired t-test).
Seven rats were implanted with cannulae unilaterally into RFA as in Experiment 2. Locations of cannula are shown in Figure 4B. In pre-operative sessions, 12 ± 3.9% of responses occurred before the end of the short delay and 39 ± 8.9% of responses occurred before the end of the long delay, a significant difference in premature responding before the end of the short and long delays (paired t-test, t(1,6) = −4.461, P < 0.01). In post-operative sessions, 14 ± 3.0% of responses occurred before the end of the short delay, unchanged from pre-operative sessions (paired t-test; t(1,6) = −0.491, P > 0.05), and responses before the end of the long delay were similarly unaffected by cannula placement (44 ± 9.0% premature responses; t(1,6) = −0.838, P > 0.05). As in Experiment 2, inactivation of RFA (using muscimol at 0.1 and 1.0 μg/μl) did not increase premature responding prior to either delay (rmANOVA: F(1,6) = 0.520, P > 0.05), although there were still significantly more premature responses later in the delay period (for the 1.0 μg/μl dose, short delay: saline = 14 ± 2.7%, muscimol = 17 ± 5.0%; long delay: saline = 45 ± 7.8%, muscimol = 40 ± 8.2%; rmANOVA; F(1,6) = 22.67, P ≪ 0.01, Figure 4C). Similar to Experiment 3, other task measures such as total trials performed and time-out responding were not affected by RFA inactivation (P > 0.10).
RTs were longer at short delays compared to long delays and this delay-dependent speeding was not altered by implantation of cannula into RFA (Short delays – pre-op: 337 ± 45 ms, post-op: 297 ± 27 ms; paired t-test, t(1,6) = 0.9448, P = 0.3812; Long delays – pre-op: 214 ± 21 ms, post-op: 194 ± 15 ms; paired t-test, t(1,6) = −1.5519, P = 0.1717). The lower dose of muscimol (0.1 μg/μl) was effective in changing RT performance (rmANOVA: F(1,2891) = 14.2361, P < 0.05), but there was no significant interaction between foreperiod and drug (rmANOVA: F(1,2891) = 0.7553, P = 0.39). RTs were not significantly different at short delays (saline: 260 ± 31 ms, 0.1 μg/μl muscimol: 313 ± 45 ms, t(1,6) = −1.67, P = 0.14) and were significantly lengthened at long delays (saline: 196 ± 16 ms, 0.1 μg/μl muscimol: 241 ± 14 ms, t(1,6) = −2.6782, P < 0.03). The effects seen in the muscimol session were steady over the test session with accuracy (t(1,6) = 0.1212, P > 0.05), trials per minute (t(1,6) = 0.6378, P > 0.05), and geometric mean reaction times (t(1,6) = −1.1818, P > 0.05) not significantly different during early vs. late test session.
The dose of 1.0 μg/μl resulted in prolonged RTs at both delays (Short – saline: 378 ± 32 ms, muscimol: 409 ± 56 ms; Wilcoxon rank-sum test, W = 25570, P < 0.01; Long – saline: 282 ± 17 ms, muscimol: 385 ± 38 ms; Wilcoxon rank-sum test, W = 335470, P < 0.01; Figure 4D). The distribution of RTs was significantly shifted to the right at both delays (rmANOVA: F(1,2896) = 8.364, P < 0.05, not shown). As for the lower dose, the interaction between delay period and drug on RT was not significant (rmANOVA: F(1,2896) = 0.113, P > 0.05).
There were clear effects of previous outcome on RT (rmANOVA: F(3,2896) = 17.754, P ≪ 0.01). That is, rats responded more quickly on trials that were preceded by quick responses (263 ± 148 ms) that were reinforced with 32% liquid sucrose compared to trials that were preceded by slow responses (939 ± 313 ms) that were reinforced with 8% liquid sucrose (Wilcoxon rank-sum test, W = 8847, P ≪ 0.01). However, the interaction between previous outcome and drug on RT was not significant (F(1,2896) = 2.466, P = 0.0705), suggesting that RFA did not mediate this sequential effect of speed/outcome.
Experiment 4: Inactivation of Agranular Insular Cortex Does not Impair RT Performance
Experiment 1 found that RFA send major projections to the dorsal part of the medial prefrontal cortex (dmPFC) and the rostral part of the insular cortex (IC). Retrograde tracer studies on the medial agranular cortex (e.g., Hoover and Vertes, 2007), where RFA is located, suggest that RFA also receives significant input from the prefrontal and insular regions. Our lab has previously shown that dmPFC is a critical region for the control of RT performance (e.g., Narayanan et al., 2006). However, to date, no study has examined how inactivation (or lesion) of IC impacts the ability of rats to perform RT tasks. To address this issue, we implanted bilateral cannula into IC in three rats and inactivated the region using infusions of muscimol (1.0 μg/μl). As in Experiments 2 and 3, cannula tips were localized using 50-μm sections of thionin-stained tissue (Figure 5A), and found to be at the rostral border of the insular cortex in the granular and dorsal agranular areas. Infusions of fluorescent muscimol revealed that the spread of the drug was confined to ∼1 mm in the AP and DV dimensions and 0.5 mm in the ML dimension (not shown), and therefore had effects both in the ventral somatosensory cortex, the granular insula, the dorsal agranular insula, and the ventral agranular insula.
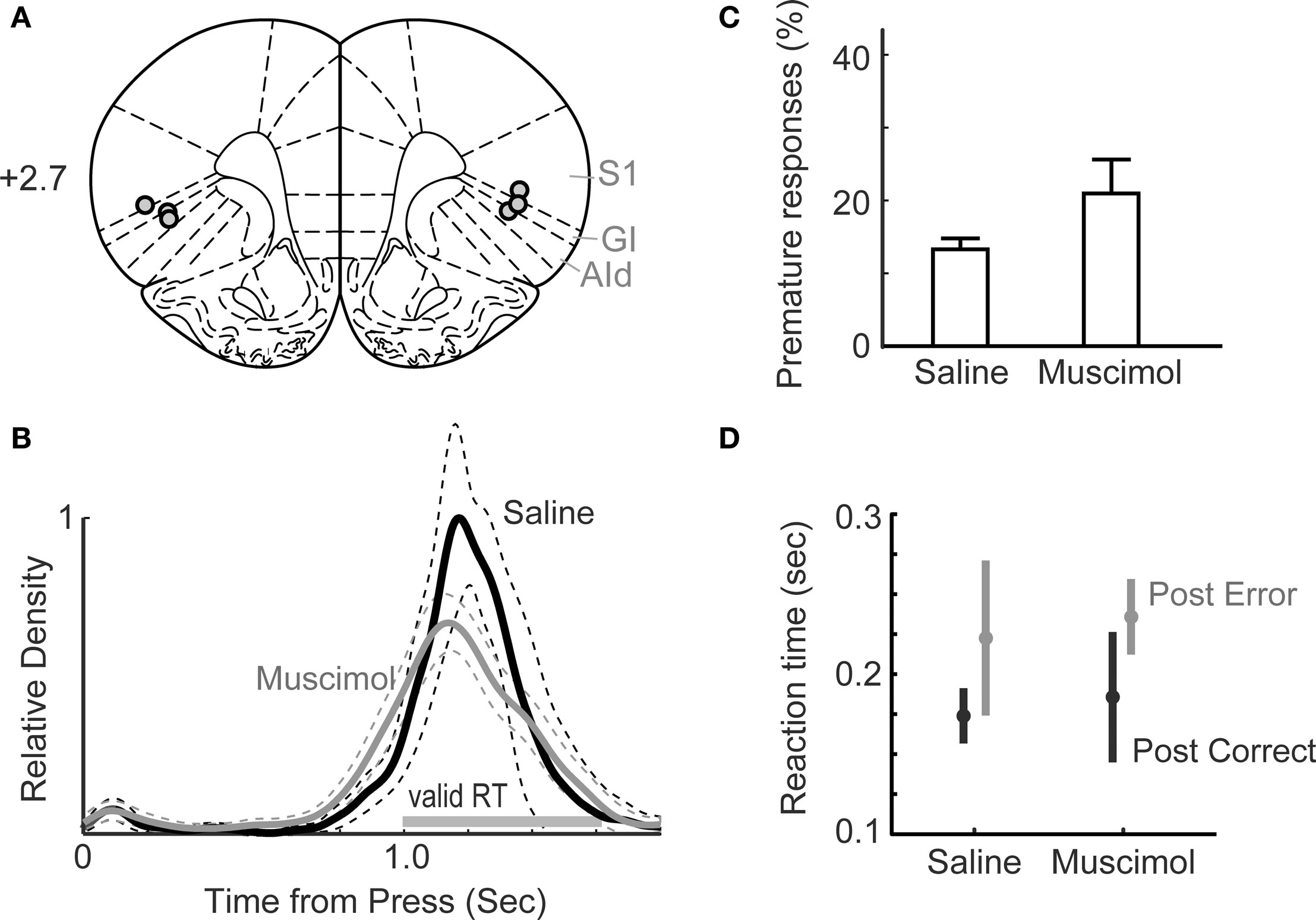
Figure 5. Behavioral performance in the one-delay version of the simple RT task following muscimol inactivation of insular cortex (IC). (A) Approximate locations of cannula tips. (B) Distributions of response duration for saline and muscimol infusions revealed no effects of IC inactivation. (rmANOVA: P > 0.05). (C) The percentage of trials with premature responses in sessions with muscimol infused into IC was elevated compared to that observed with saline in IC, but the difference was not significant (t-test: P > 0.05). (D) Mean RTs from sessions with saline or muscimol in IC and trials preceded by correct (post-correct) and premature error (post-error) responses were not significantly different (t-test: P > 0.05). In both types of sessions, RTs were longer on trials preceded by errors compared to trials preceded by correct responses (t-test: P < 0.05).
Implantation of cannula did not alter measures of task performance. In pre-operative sessions, 20.8 ± 5.9% of responses were premature (i.e., lever releases before the presentation of the stimulus) and 2.5 ± 0.5 % were late responses (i.e., lever releases more than 600 ms after the presentation of the stimulus). In post-operative sessions, 20.0 ± 3.9 % of trials were premature and 3.2 ± 0.0 % were late, both unchanged from pre-operative sessions (t(1,2) = 0.2241, P = 0.8435 for premature responses; t(1,2) = −1.1161, P = 0.3805 for late responses). Also, the geometric mean of reaction times was unchanged in pre- and post-operative sessions (pre-op: 194 ± 40 ms, post-op: 230 ± 16 ms; paired t-test, t(1,2) = −1.0057, P = 0.4204).
In sessions with insular cortex inactivated by 0.1 μg/μl muscimol, we observed no significant change in the distribution of response durations (Figure 5B) and no increase in premature (13.3 ± 1.2%; paired t-test, t(1,2) = −2.5375, P = 0.1265; Figure 5C) or late (2.0 ± 0.5%; paired t-test, t(1,2) = −1.0246, P = 0.4133; not shown) responding. The geometric mean of reaction times did not differ between saline (189 ± 13 ms) and muscimol (218 ± 32 ms) sessions (paired t-test, t(1,2) = −1.1863, P = 0.3573).
Rats can show post-error slowing (Narayanan and Laubach, 2008) and this process may result from a memory of preceding trial outcomes or depend on encoding of reward or an incentive contrast effect. Both of these processes depend on insular cortex (e.g., Balleine and Dickinson, 2000; Kesner and Gilbert, 2007). Therefore, we examined if there were changes in post-error adjustments following inactivation of insular cortex and found no effects of trial outcomes on subsequent reaction times (post-correct: saline = 174 ± 14 ms vs. muscimol = 186 ± 33 ms, paired t(1,2) = −0.4822, P = 0.6773; post-error: saline = 223 ± 40 vs. muscimol = 236 ± 19, paired t(1,2) = −0.4082, P = 0.7227; Figure 5D).
Discussion
In the present study, a combination of tract-tracing and reversible inactivation methods was used to examine the role of a motor-related area in the rat frontal cortex, the RFA, in the top–down control of action. Neural tracing studies involved using electrical microstimulation to identify RFA and BDAs to map out connections of RFA with other parts of the frontal cortex. Connections were found between RFA and mPFC, the agranular insular cortex, and the primary motor cortex. These connections have been reported previously for the medial agranular cortex (Reep et al., 1990; Hoover and Vertes, 2007). However, the present study is the first to identify these connections based on injections of anterograde tracers at sites that were identified as RFA based on electrical microstimulation and is the first study to establish connections between RFA and medial and orbital parts of the rodent frontal cortex. A notable finding was that connections between these areas are based in the superficial layers of cortex. These layers exhibit synaptic plasticity during learning (Rioult-Pedotti et al., 1998), control output neurons located in the deep layers of cortex (Weiler et al., 2008), and coordinate the dynamics of neural processing based on interactions between pyramidal neurons and interneurons (Isomura et al., 2009). As such, processing between mPFC, RFA, and the insular cortex could enable a coordinated control of network dynamics across the cortical regions and with regard to the descending control of subcortical systems such as the thalamus, basal ganglia, hypothalamus, amygdala, and the monoaminergic nuclei.
Furthermore, reversible inactivation methods were used to examine effects of inhibiting neural activity in RFA on RT performance. Muscimol infusions into RFA led to increased response times and eliminated delay-dependent speeding and did not lead to increased premature responding. These results are markedly different from what is obtained when muscimol is infused into mPFC, which leads to excessive premature responding and a reduction of RTs stimuli at short delays (Narayanan et al., 2006). Our results provide evidence for mPFC and RFA having distinct roles in controlling RT performance, suggesting that RFA is part of a preparatory system that is crucial for speeding performance and that competes with mPFC to control action selection, as explained below.
In addition to examining effects of RFA inactivation, we tested animals during the RT task after inactivating the insular cortex, which we found receives inputs from RFA and is known to also be interconnected with mPFC (Gabbott et al., 2003). We found no effects of IC inactivation on RT performance in terms of RT variability, premature responding, and the effects of preceding trial outcomes on RTs. We only examined effects of IC inactivation in three rats due to our finding no effect of inactivation. We are reporting this result because we have obtained positive results in 25 of 25 rats studied with infusions of muscimol into the mPFC (Narayanan et al., 2006; Narayanan and Laubach, 2006). These results suggest that top–down control arises from specific interactions between mPFC and RFA, and does not involve processing in IC. However, it is possible that IC has a role in mediating incentive properties of the liquid sucrose rewards. More impressive effects of IC manipulation might have been obtained if methods such as those used in Exp 2 (higher sucrose given for faster response) had been used to examine IC function in the simple RT task.
Based on a conceptual model for top–down control (Figure 1A), we suggest that RFA controls the threshold that is used to initiate responding and generates a prepotent excitation over responding that is crucial for temporal preparation. We propose that two processes compete during the delay period to control the timing of action. One process is called “Proactive Inhibition”, is based in mPFC, and serves to limit inappropriate responding until the stimulus is delivered, presumably by decaying over the delay period (Figure 1B). The other process is called “Prepotent Excitation”, is based in RFA, and serves to increase the level of activation in the motor system, presumably by growing over the delay period (Figure 1B). (It is important to point out that the concept of prepotent excitation is similar to “non-specific” or temporal preparation in models of human RT performance (Los and van den Heuvel, 2001). The difference between proactive inhibition (I) and prepotent excitation (E) serves as an activation function (A, defined as A = E – I + ν, where ν is a noise term) and responses are initiated if this function is greater than the Threshold (i.e., respond if A > T; wait if A < T). To date, experimental support for this model was reported in rodent studies by Narayanan et al. (2006) and Narayanan and Laubach (2009) and in human studies by Jaffard et al. (2008) and Boulinguez et al. (2008). The present study is the first to provide experimental support for a potential role of “premotor” cortex in contributing to the “Prepotent Excitation” component of the model.
A network model is proposed in Figures 6A,B that may reveal the direction of influences across these frontal regions that underlie top–down control. One triad of areas, comprised of medial frontal cortex (MFC), “premotor” cortex (PMC), and the insular cortex (IC) would integrate inhibitory (MFC) and preparatory (PMC) processes and receive information about trial outcomes from IC (taste-based feedback). A second triad of areas, comprised of the primary motor cortex (MC), PMC, and IC would allow for linking sensorimotor processing with outcome-related information and would allow for integrating bottom–up signals from the sensorimotor and taste systems with top–down (preparatory) signals in PMC. These network components would be complimented by timing signals, which initiate the top–down control circuit, from the sensorimotor cortex (e.g., proprioception) and stimulus-related inputs to RFA from the perirhinal cortex and posterior insula (Kyuhou et al., 2003). These bottom–up signals should be crucial for controlling the onset and offset of the top–down control system. An important issue with this view of RT performance is that the top–down control system functions not through an accumulation of evidence about when to act. Instead, top–down control is based on a balance of prepotent excitation and proactive inhibition based on activity that is largely segregated across two distinct parts of frontal cortex (mPFC and RFA).
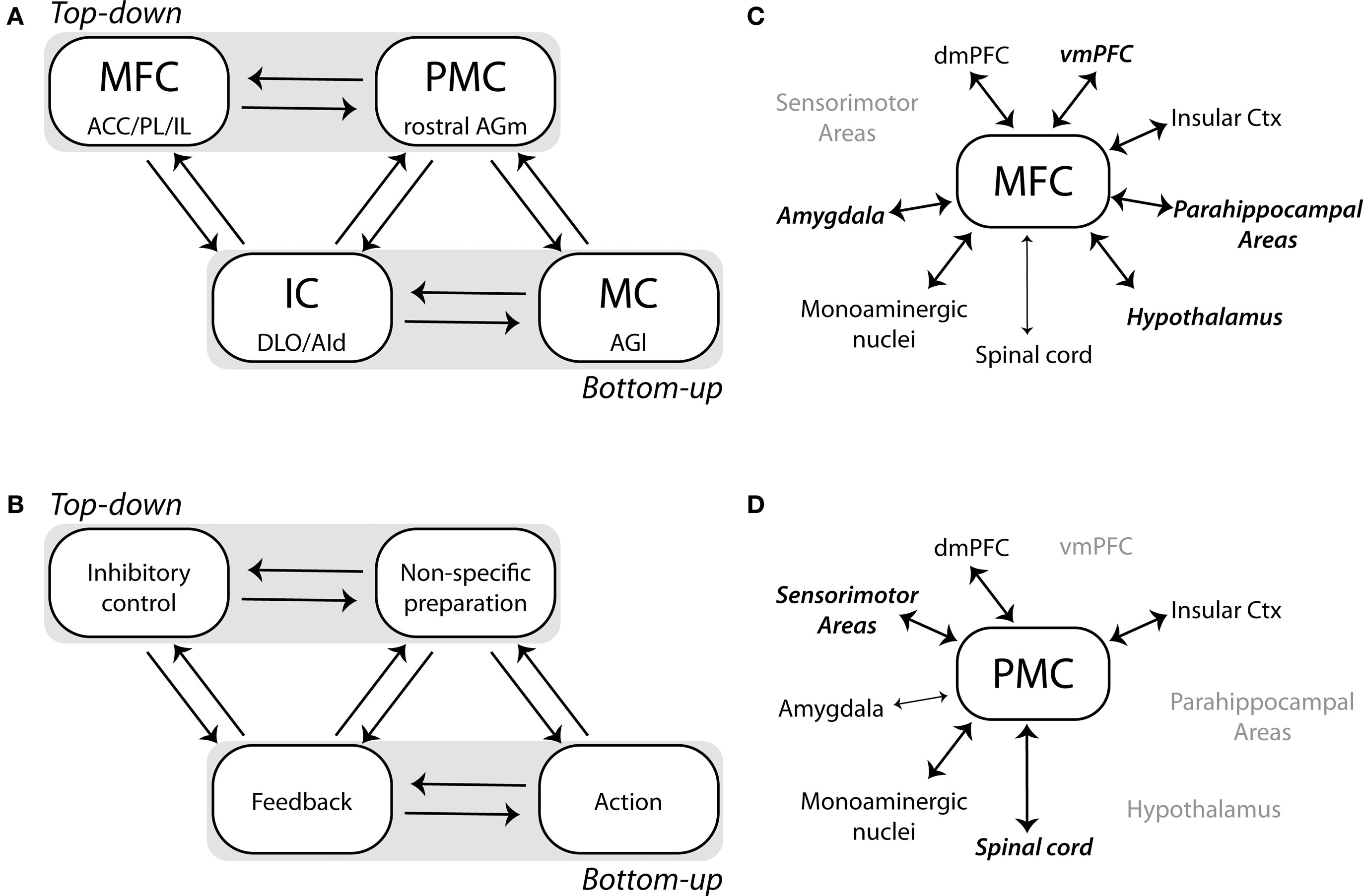
Figure 6. Network model for top–down control is proposed that is based on functional interactions between four regions of the frontal cortex. (A,B) The medial frontal cortex (MFC), comprised of the anterior cingulate (ACC), prelimbic (PL), and infralimbic (IL) areas, interacts with the “premotor” cortex (PMC, the “rostral forelimb area” located in the rostral medial agranular (AGm) region) and the insular cortex (IC, comprised of the dorsolateral orbital (DLO) and dorsal agranular insular (AId) regions). MFC and PMC have distinct roles in top–down control, inhibitory control in MFC and non-specific (i.e., temporal) preparation in PMC. The primary motor cortex (MC), located in the lateral agranular area (AGl), interacts with PMC and IC allowing for bottom–up control, with proprioceptive feedback from the motor cortex and taste-based feedback from IC. (C,D) A survey of the anatomical literature (see text) reveals several key brain regions that are selectively connected with either the MFC or PMC networks. Dorsal areas in MFC are extensively interconnected with the ventral infralimbic area, the amygdala, parahippocampal regions, and the hypothalamus. By contrast, PMC lacks connectivity with these regions and is heavily interconnected with sensory and motor brain regions, including the spinal cord.
A survey of the anatomical literature reveals several key brain regions that are selectively connected with mPFC and RFA (Figures 6C,D, where mPFC is denoted as MFC for “Medial Frontal Cortex” and RFA is denoted as PMC for “PreMotor Cortex”; these terms are used to allow for extension to studies in primates and human beings). The dorsal mPFC is extensively interconnected with the ventral mPFC (i.e., infralimbic area), the amygdala, parahippocampal regions, and the hypothalamus (Gabbott et al., 2003, 2005; Hoover and Vertes, 2007). By contrast, RFA lacks connectivity with these regions and is heavily interconnected with sensory and motor brain regions, including the spinal cord (Reep et al., 1990; Hoover and Vertes, 2007). As such, mPFC neurons are potentially influenced by brain regions associated with the limbic system and RFA by regions with clear roles in sensorimotor control. A key issue is that dopamine neurons in the midbrain project to RFA (Hoover and Vertes, 2007), but only very weakly to the primary motor cortex (Donoghue and Parham, 1983). The RFA may therefore be the place where the cortical motor system is influenced by the rewarding properties of task stimuli. As neurons in this region of cortex have been shown to exhibit altered task-related firing during initial learning (Laubach, et al., 2000), it would seem important for future studies to clarify the functional basis for the executive and motivational control of neural activity in the rat premotor cortex.
Conflict of Interest Statement
The authors declare that the research was conducted in the absence of any commercial or financial relationships that could be construed as a potential conflict of interest.
Acknowledgments
The studies were supported by funds from the National Science Foundation (Grant 194344) to ML and from Pfizer (the Pfizer Goldman-Rakic Fellowship) to Nathaniel J. Smith. Experiment 1 was carried out by Nathaniel J. Smith and Marcelo S. Caetano, and data analysis was done by Nathaniel J. Smith. Experiments 2 and 3 were carried out by Nathaniel J. Smith and Benjamine Liu, and data analysis was done by Nathaniel J. Smith. All aspects of experiment 4 were carried out by Nicole K. Horst. Figures 1 and 6 were created by Mark Laubach. Figure 2 was created by Mark Laubach and Nathaniel J. Smith. Figures 3 and 4 were created by Nathaniel J. Smith. Figure 5 was created by Nicole K. Horst. The manuscript was written by Mark Laubach, Nathaniel J. Smith, and Nicole K. Horst. We thank Linda Harenberg for helpful comments on the manuscript and thank the Instruments Shop at the John B. Pierce Laboratory, especially Tom D’Alessandro, for outstanding technical support.
References
Adams, J. C. (1981). Heavy metal intensification of DAB-based HRP reaction product. J. Histochem. Cytochem. 29, 775.
Balleine, B. W., and Dickinson, A. (2000). The effect of lesions of the insular cortex on instrumental conditioning: evidence for a role in incentive memory. J. Neurosci. 20, 8954–8964.
Boulinguez, P., Jaffard, M., Granjon, L., and Benraiss, A. (2008). Warning signals induce automatic EMG activations and proactive volitional inhibition: evidence from analysis of error distribution in simple RT. J. Neurophysiol. 99, 1572–1578.
Brasted, P. J., Dunnett, S. B., and Robbins, T. W. (2000). Unilateral lesions of the medial agranular cortex impair responding on a lateralised RT task. Behav. Brain Res. 111, 139–151.
Brown, V. J., Bowman, E. M., and Robbins, T. W. (1991). Response-related deficits following unilateral lesions of the medial agranular cortex of the rat. Behav. Neurosci. 105, 567–578.
Donoghue, J. P. (1985). Contrasting properties of neurons in two parts of the primary motor cortex of the awake rat. Brain Res. 333, 173–177.
Donoghue, J. P., and Parham, C. (1983). Afferent connections of the lateral agranular field of the rat motor cortex. J. Comp. Neurol. 217, 390–404.
Donoghue, J. P., and Wise, S. P. (1982). The motor cortex of the rat: cytoarchitecture and microstimulation mapping. J. Comp. Neurol. 212, 76–88.
Gabbott, P. L., Warner, T. A., Jays, P. R., and Bacon, S. J. (2003). Areal and synaptic interconnectivity of prelimbic (area 32), infralimbic (area 25) and insular cortices in the rat. Brain Res. 993, 59–71.
Gabbott, P. L., Warner, T. A., Jays, P. R., Salway, P., and Busby, S. J. (2005). Prefrontal cortex in the rat: Projections to subcortical autonomic, motor, and limbic centers. J. Comp. Neurol. 492, 145–177.
Good, P. I. (2000). Permutation Tests: A Practical Guide to Resampling Methods for Testing Hypotheses, Springer Series in Statistics. New York: Springer.
Hoover, W. B., and Vertes, R. P. (2007). Anatomical analysis of afferent projections to the medial prefrontal cortex in the rat. Brain Struct. Funct. 212, 149–179.
Hyland, B. (1998). Neural activity related to reaching and grasping in rostral and caudal regions of rat motor cortex. Behav. Brain Res. 94, 255–269.
Isomura, Y., Harukuni, R., Takekawa, T., Aizawa, H., and Fukai, T. (2009). Microcircuitry coordination of cortical motor information in self-initiation of voluntary movements. Nat. Neurosci. 12, 1586–1593.
Jaffard, M., Longcamp, M., Velay, J. L., Anton, J. L., Roth, M., Nazarian, B., and Boulinguez, P. (2008). Proactive inhibitory control of movement assessed by event-related fMRI. Neuroimage 42, 1196–1206.
Kesner, R. P., and Gilbert, P. E. (2007). The role of the agranular insular cortex in anticipation of reward contrast. Neurobiol. Learn Mem. 88, 82–86.
Krupa, D. J., Ghazanfar, A. A., and Nicolelis, M. A. (1999). Immediate thalamic sensory plasticity depends on corticothalamic feedback. Proc. Natl. Acad. Sci. U.S.A. 96, 8200–8205.
Kyuhou, S., Matsuzaki, R., and Gemba, H. (2003). Perirhinal cortex relays auditory information to the frontal motor cortices in the rat. Neurosci. Lett. 353, 181–184.
Laubach, M., Wessberg, J., and Nicolelis, M. A. (2000). Cortical ensemble activity increasingly predicts behaviour outcomes during learning of a motor task. Nature 405, 567–571.
Lomber, S. G. (1999). The advantages and limitations of permanent or reversible deactivation techniques in the assessment of neural function. J. Neurosci. Methods 86, 109–117.
Los, S. A., and van den Heuvel, C. E. (2001). Intentional and unintentional contributions to nonspecific preparation during RT foreperiods. J. Exp. Psychol. Hum. Percept. Perform. 27, 370–386.
Martin, J. H., and Ghez, C. (1999). Pharmacological inactivation in the analysis of the central control of movement. J. Neurosci. Methods 86, 145–159.
Narayanan, N. S., Horst, N. K., and Laubach, M. (2006). Reversible inactivations of rat medial prefrontal cortex impair the ability to wait for a stimulus. Neuroscience 139, 865–876.
Narayanan, N. S., and Laubach, M. (2006). Top-down control of motor cortex ensembles by dorsomedial prefrontal cortex. Neuron 52, 921–931.
Narayanan, N. S., and Laubach, M. (2008). Neuronal correlates of post-error slowing in the rat dorsomedial prefrontal cortex. J. Neurophysiol. 100, 520–525.
Narayanan, N. S., and Laubach, M. (2009). Delay activity in rodent frontal cortex during a simple RT task. J. Neurophysiol. 101, 2859–2871.
Neafsey, E. J., Bold, E. L., Haas, G., Hurley-Gius, K. M., Quirk, G., Sievert, C. F., and Terreberry, R. R. (1986). The organization of the rat motor cortex: a microstimulation mapping study. Brain Res. 396, 77–96.
Neafsey, E. J., and Sievert, C. (1982). A second forelimb motor area exists in rat frontal cortex. Brain Res. 232, 151–156.
Niki, H., and Watanabe, M. (1979). Prefrontal and cingulate unit activity during timing behavior in the monkey. Brain Res. 171, 213–224.
Ostlund, S. B., Winterbauer, N. E., and Balleine, B. W. (2009). Evidence of action sequence chunking in goal-directed instrumental conditioning and its dependence on the dorsomedial prefrontal cortex. J. Neurosci. 29, 8280–8287.
Passetti, F., Chudasama, Y., and Robbins, T. W. (2002). The frontal cortex of the rat and visual attentional performance: dissociable functions of distinct medial prefrontal subregions. Cereb. Cortex 12, 1254–1268.
Paxinos, G., and Watson, C. (1998). The Rat Brain in Stereotaxic Coordinates, 4th Edn. New York: Academic Press.
Picard, N., and Strick, P. L. (1996). Motor areas of the medial wall: a review of their location and functional activation. Cereb. Cortex 6, 342–353.
Preuss, T. M. (1995). Do rats have prefrontal cortex? The Rose-Woolsey-Akert program reconsidered. J. Cogn. Neurosci. 7, 1–24.
Reep, R. L., and Corwin, J. V. (1999). Topographic organization of the striatal and thalamic connections of rat medial agranular cortex. Brain Res. 841, 43–52.
Reep, R. L., Goodwin, G. S., and Corwin, J. V. (1990). Topographic organization in the corticocortical connections of medial agranular cortex in rats. J. Comp. Neurol. 294, 262–280.
Reiner, A., Veenman, C. L., Medina, L., Jiao, Y., Del Mar, N., and Honig, M. G. (2000). Pathway tracing using biotinylated dextran amines. J. Neurosci. Methods 103, 23–37.
Rioult-Pedotti, M. S., Friedman, D., Hess, G., and Donoghue, J. P. (1998). Strengthening of horizontal cortical connections following skill learning. Nat. Neurosci. 1, 230–234.
Risterucci, C., Terramorsi, D., Nieoullon, A., and Amalric, M. (2003). Excitotoxic lesions of the prelimbic-infralimbic areas of the rodent prefrontal cortex disrupt motor preparatory processes. Eur. J. Neurosci. 17, 1498–1508.
Sesack, S. R., Deutch, A. Y., Roth, R. H., and Bunney, B. S. (1989). Topographical organization of the efferent projections of the medial prefrontal cortex in the rat: an anterograde tract-tracing study with Phaseolus vulgaris leucoagglutinin. J. Comp. Neurol. 290, 213–242.
Sievert, C. F., and Neafsey, E. J. (1986). A chronic unit study of the sensory properties of neurons in the forelimb areas of rat sensorimotor cortex. Brain Res. 381, 15–23.
Silverman, B. W. (1986). Density Estimation for Statistics and Data Analysis. London, UK: Chapman and Hall.
Stuss, D. T., Alexander, M. P., Shallice, T., Picton, T. W., Binns, M. A., Macdonald, R., Borowiec, A., and Katz, D. I. (2005). Multiple frontal systems controlling response speed. Neuropsychologia 43, 396–417.
van Duuren, E., van der Plasse, G., van der Blom, R., Joosten, R. N., Mulder, A. B., Pennartz, C. M., and Feenstra, M. G. (2007). Pharmacological manipulation of neuronal ensemble activity by reverse microdialysis in freely moving rats: a comparative study of the effects of tetrodotoxin, lidocaine, and muscimol. J. Pharmacol. Exp. Ther. 323, 61–69.
Vertes, R. P. (2004). Differential projections of the infralimbic and prelimbic cortex in the rat. Synapse 51, 32–58.
Wang, Y., and Kurata, K. (1998). Quantitative analyses of thalamic and cortical origins of neurons projecting to the rostral and caudal forelimb motor areas in the cerebral cortex of rats. Brain Res. 781, 135–147.
Wang, Y., Matsuzaka, Y., Mushiake, H., and Shima, K. (2008). Spatial distribution of cingulate cortical cells projecting to the primary motor cortex in the rat. Neurosci. Res. 60, 406–411.
Keywords: reaction time, executive control, sensorimotor, frontal cortex, muscimol, tract tracing
Citation: Smith NJ, Horst NK, Liu B, Caetano MS and Laubach M (2010) Reversible inactivation of rat premotor cortex impairs temporal preparation, but not inhibitory control, during simple reaction-time performance. Front. Integr. Neurosci. 4:124. doi:10.3389/fnint.2010.00124
Received: 06 August 2010;
Paper pending published: 23 August 2010;
Accepted: 06 September 2010;
Published online: 08 October 2010
Edited by:
Sidney A. Simon, Duke University, USAReviewed by:
Marcos G. Frank, University of Pennsylvania, USAPatricia H. Janak, University of California at San Francisco, USA
Copyright: © 2010 Smith, Horst, Liu, Caetano and Laubach. This is an open-access article subject to an exclusive license agreement between the authors and the Frontiers Research Foundation, which permits unrestricted use, distribution, and reproduction in any medium, provided the original authors and source are credited.
*Correspondence: Mark Laubach, The John B. Pierce Laboratory, New Haven, CT, USA. e-mail: mlaubach@jbpierce.org