ROS-GC interlocked Ca2+-sensor S100B protein signaling in cone photoreceptors: review
- 1Research Divisions of Biochemistry and Molecular Biology, The Unit of Regulatory and Molecular Biology, Salus University, Elkins Park, PA, USA
- 2Department of Ophthalmology, Massachusetts Eye and Ear Infirmary, Harvard Medical School, Boston, MA, USA
- 3Department of Neurobiology of Rhythms, Institute for Cellular and Integrative Neuroscience, CNRS UPR 3212, Strasbourg, France
Photoreceptor rod outer segment membrane guanylate cyclase (ROS-GC) is central to visual transduction; it generates cyclic GMP, the second messenger of the photon signal. Photoexcited rhodopsin initiates a biochemical cascade that leads to a drop in the intracellular level of cyclic GMP and closure of cyclic nucleotide gated ion channels. Recovery of the photoresponse requires resynthesis of cyclic GMP, typically by a pair of ROS-GCs, 1 and 2. In rods, ROS-GCs exist as complexes with guanylate cyclase activating proteins (GCAPs), which are Ca2+-sensing elements. There is a light-induced fall in intracellular Ca2+. As Ca2+ dissociates from GCAPs in the 20–200 nM range, ROS-GC activity rises to quicken the photoresponse recovery. GCAPs then progressively turn down ROS-GC activity as Ca2+ and cyclic GMP levels return to baseline. To date, GCAPs mediate the only known mechanism of ROS-GC regulation in the photoreceptors. However, in mammalian cone outer segments, cone synapses and ON bipolar cells, another Ca2+ sensor protein, S100B, complexes with ROS-GC1 and senses the Ca2+ signal with a K1/2 of 400 nM. Unlike GCAPs, S100B stimulates ROS-GC activity when Ca2+ is bound. Thus, the ROS-GC system in cones functions as a Ca2+ bimodal switch; with rising intracellular Ca2+, its activity is first turned down by GCAPs and then turned up by S100B. This presentation provides a historical perspective on the role of S100B in the photoreceptors, offers a pictorial model for the “bimodal” operation of the ROS-GC switch and projects future tasks that are needed to understand its operation. Some accounts of this review have been adopted from the original publications of these authors.
Introduction
A seminal observation in the field of visual transduction was that a soluble bovine rod outer segment (ROS) fraction kicked the catalytic activity of a photoreceptor guanylate cyclase into high gear in the absence of Ca2+ (Koch and Stryer, 1988). The structure of this guanylate cyclase was believed to be composed of “separate regulatory and catalytic subunits” (Stryer, 1991); however, elucidating the molecular identity of the guanylate cyclase and its regulator turned out to be quite complicated. There were reports that guanylate cyclase possessed a molecular mass of 67 kDa and was nitric oxide sensitive (Horio and Murad, 1991a,b). Yet another report claimed to have solved the molecular structure of the photoreceptor retGC, the human retinal guanylate cyclase (Shyjan et al., 1992).
Later, the true photoreceptor ROS-guanylate cyclase (ROS-GC) was purified directly from bovine outer segments (Margulis et al., 1993) and its protein-sequence-based molecular cloning, structure, and function were established (Goraczniak et al., 1994). ROS-GC had a calculated molecular mass of 120,360 Da, a value similar to 112,000 Da reported earlier for a bovine (Koch, 1991) and 110,000–115,000 Da for toad photoreceptor guanylate cyclase (Hayashi and Yamazaki, 1991). Unlike the other known membrane guanylate cyclases, atrial natriuretic factor receptor guanylate cyclase (ANF-RGC; Paul et al., 1987; Sharma, 1988; Chinkers et al., 1989; Duda et al., 1991) and type C natriuretic peptide CNP-RGC guanylate cyclase (Koller et al., 1991; Duda et al., 1993), ROS-GC was not hormonally responsive (Goraczniak et al., 1994). It thus represented a new subfamily of the membrane guanylate cyclases. Further study revealed that ROS-GC was not composed of “separate regulatory and catalytic subunits”, it was not nitric oxide sensitive nor was its structure identical to retGC. The RetGC structure was eventually revised to match that of bovine ROS-GC (Accession number M92432).
Meanwhile, groups from the U.S. and the former Soviet Union joined forces to describe a newly purified Ca2+ binding protein, recoverin, that appeared to regulate guanylate cyclase activity (Dizhoor et al., 1991) and adhere to the functional description of Koch and Stryer (Koch and Stryer, 1988). It was therefore considered a Ca2+ modulator of ROS-GC; hence, named recoverin “because it promotes recovery of the dark state” (Dizhoor et al., 1991). However, ever purer preparations of recoverin showed a disturbing decline in guanylate cyclase stimulation. It became evident that recoverin was not the sought after regulator of guanylate cyclase activity and the initial conclusion was withdrawn (Hurley et al., 1993). Instead, recoverin exerted Ca2+-dependent control over the phosphorylation of photoexcited rhodopsin by rhodopsin kinase (Chen et al., 1995; Klenchin et al., 1995; Kawamura, 1999). At almost the same time, two separate groups discovered similar but distinct guanylate cyclase activating proteins (GCAPs), GCAP1 (Palczewski et al., 1994; Subbaraya et al., 1994; Gorczyca et al., 1995; Frins et al., 1996) and GCAP2 (Dizhoor et al., 1995). These GCAPs did indeed stimulate ROS-GC activity as described by Koch and Stryer (1988).
Recombinant ROS-GC expressed in a heterologous system of COS cells responded at 10 nM [Ca2+]i to GCAP1 stimulation in a dose-dependent manner (Duda et al., 1996b). The stimulation was inhibited cooperatively by free Ca2+ with a K1/2 of 100 nM. Under identical conditions, GCAP1 had no effect on recombinant ANF-RGC, the peptide hormone receptor guanylate cyclase. An important characteristic of the transduction system was that GCAP remained bound to ROS-GC at low and high Ca2+ levels, consistent with physiological observations (Koutalos et al., 1995). Similar reconstitution studies established GCAP2 as another Ca2+-sensing subunit of ROS-GC (Dizhoor et al., 1995; Gorczyca et al., 1995). When a second ROS-GC was subsequently discovered in the bovine retina, termed Ret-GC2 in human retina (Lowe et al., 1995), it was named ROS-GC2 to distinguish it from the original ROS-GC which was renamed as ROS-GC1 (Goraczniak et al., 1997). It became clear that a Ca2+-modulated system, composed of a pair of ROS-GCs and a pair of GCAPs, subserves phototransduction in the outer segments of photoreceptors. This conclusion was supported at the physiological level by the studies with the double knockout ROS-GC1/ROS-GC2 mouse model (Baehr et al., 2007). Rods and cones were non-functional, excluding the presence of a third guanylate cyclase linked with phototransduction.
Thus ROS-GCs are related to peptide hormone receptor guanylate cyclases but they do not respond to extracellular ligands. Instead, they exist in stable complexes with GCAPs that sense changes in internal [Ca2+]. The feature that incrementing [Ca2+]i progressively reduces ROS-GC1 catalytic activity was academically challenging because such a mechanism had never been observed before for any of the members of the guanylate cyclase family. All other members were solely stimulated by their effector ligands, natriuretic peptide hormones, at their extracellular domains. The molecular properties of this remarkable system and the role that the system plays in phototransduction are described in greater depth in Pugh et al. (1997), Sharma and Duda (2012).
Discovery of CD-GCAP Meant that ROS-GC1 Could Operate as a Ca2+ Bimodal Transduction Switch
Contemporaneous with the discovery of GCAPs that stimulated ROS-GC1 at low Ca2+, a post-mitochondrial 100,000 g supernatant fraction from retina was found to stimulate recombinant ROS-GC at high Ca2+ by as much as 25-fold (Pozdnyakov et al., 1995). The new factor was a peptide with an apparent molecular weight of 6–7 kDa protein that oligomerized to a functional size of 40 kDa. It stimulated native and recombinant ROS-GC with a K1/2 for Ca2+ of 2 μM with biochemical characteristics typical of a Ca2+-binding protein. To distinguish it from the just-discovered GCAPs, the new factor was named CD-GCAP (Ca2+-dependent guanylate cyclase activator protein; Pozdnyakov et al., 1995).
The capacity for ROS-GC to couple to GCAPs or to CD-GCAP allows for a cell to sculpt its response to Ca2+. Depending upon the cell’s specific needs, it could express either GCAP or CD-GCAP to stimulate cyclic GMP synthesis at low (tens of nanomolar) or at high (sub-micromolar) ranges of [Ca2+]. An even more exciting possibility exists for the simultaneous expression of both Ca2+-regulatory subunits in the same cell. ROS-GC could then operate as a Ca2+-bimodal transduction switch, stimulated at low and at high free [Ca2+] by Ca2+ -free GCAPs and by Ca2+-bound S100B, respectively. A theoretical Ca2+-BIMODAL ROS-GC transduction model embodying these features was proposed (Figure 1). Bimodal Ca2+ sensing could be an elegant general mechanism for neural transmission.
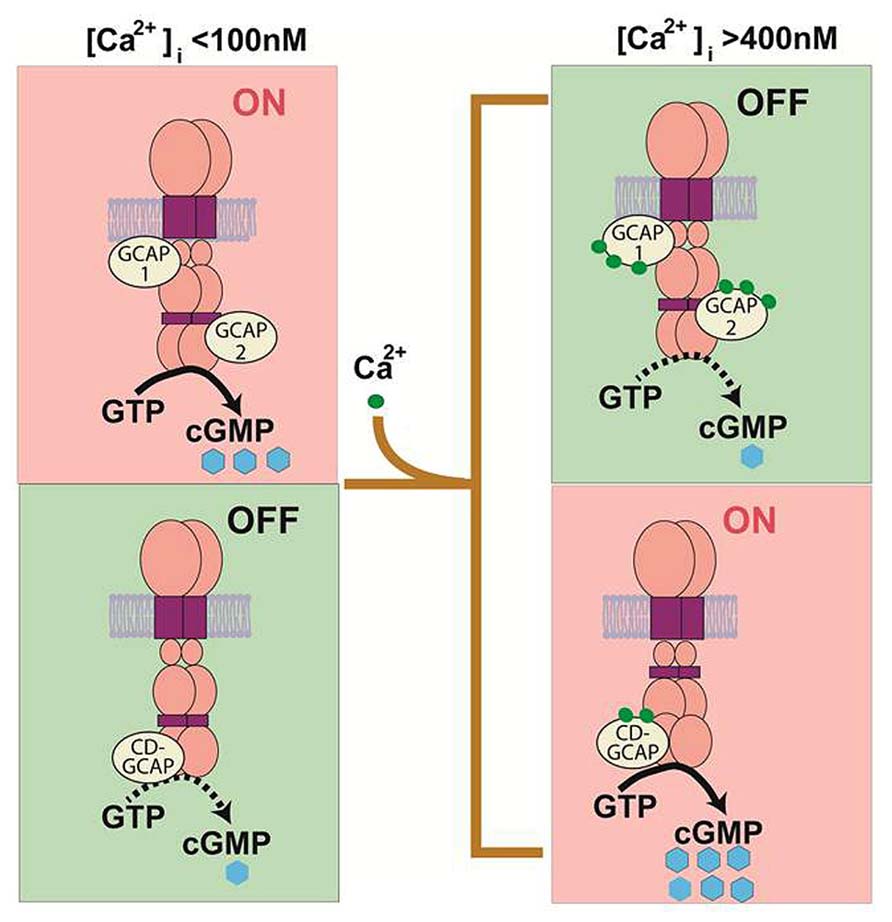
FIGURE 1. Original theoretical Ca2+ bimodal ROS-GC transduction model. In the absence of bound Ca2+, GCAPs 1 and 2 boost ROS-GC1 catalytic activity. In contrast, S100B boosts ROS-GC1 activity in the Ca2+-bound state. GCAPs sense [Ca2+]i < 200 nM while S100B senses [Ca2+]i > 200 nM. Thus in the GCAPs operational mode the S100B switch is “OFF” and in the S100B operational mode the GCAP switch is “OFF” (Upgraded with permission from Sharma, 2010; Sharma and Duda, 2012).
CD-GCAP is an S100 Protein
The first indication that CD-GCAP might be related to S100 proteins was that it appeared as a 6–7 kDA Ca2+-binding protein on SDS-PAGE (Pozdnyakov et al., 1995). S100 proteins are known to run anomalously as 6–7 kDa proteins in SDS-PAGE even though their subunit molecular weight, calculated from amino acid (aa) sequence, is about 10.5 kDa (Kligman and Marshak, 1985). Additional evidence for the structural similarity of CD-GCAP to S100 emerged from mass spectrometry analysis (Pozdnyakov et al., 1997). The fragmented molecular masses of 10,580 Da for CD-GCAP and 10,582 Da for S100B were essentially the same. Tryptic digests also yielded indistinguishable fragmentation patterns. Finally, bovine CD-GCAP and bovine S100B were cloned and their predicted primary protein structures were identical (Pozdnyakov et al., 1997).
Despite being structurally identical proteins, biochemical analyses revealed two surprising functional discrepancies. As expected, S100A1–S100B and S100ββ dimer (S100B) from commercial sources stimulated cloned recombinant and wt-photoreceptor ROS-GC (Duda et al., 1996a; Margulis et al., 1996). But the Vmax of ROS-GC was about 50% higher when activated by CD-GCAP than when activated by commercially obtained S100B. In addition, the commercial S100B required about 20-times more calcium (3.2 x 10-5 M vs. 1.5 x 10-6 M for CD-GCAP) for half-maximal stimulation of guanylate cyclase. Clearly, CD-GCAP was not quite the same as the commercially obtained S100B.
In the search for the explanation for these functional differences, the proteins were analyzed for post-translational modifications and higher order structures (Pozdnyakov et al., 1997). After treating both proteins with hydroxylamine, a deacylating agent, CD-GCAP no longer activated guanylate cyclase while S100B activation retained its ability to do so. Furthermore, hydroxylamine fragmented CD-GCAP while S100B was unaffected. These results indicated that the conformations of the two proteins were different. Such a difference could have arisen from variations in the procedures for purifying the two proteins: CD-GCAP purification entailed heating at 75°C in 5 mM Ca2+, while S100B purification included zinc affinity chromatography (commercial sources refused to provide these details but their references indicated so). To investigate, CD-GCAP was subjected to zinc affinity chromatography (CD-GCAP-to-S100B) and S100B was heated to 75°C in Ca2+ (S100B-to-CD-GCAP). Guanylate cyclase activation, calcium–sensitivity, and hydroxylamine lability measurements demonstrated that CD-GCAP-to-S100B was identical to S100B and that S100B-to-CD-GCAP was identical to CD-GCAP. Apparently zinc inhibits the native S100B potency toward stimulation of ROS-GC. An additional finding was that S100B monomer is about twice as effective as the dimer in activating the ROS-GC catalytic activity. Hence, CD-GCAP and commercially obtained S100B are conformational isomers. The native form of S100B present in the retinal neurons is CD-GCAP.
Characterization of the S100B Binding Site of ROS-GC1
Direct interaction between ROS-GC1 and S100B was verified by cross-linking experiments with bis-(sulfosuccinimidyl)suberate. A cross-linked complex, consisting of ROS-GC1 dimer and S100B, was detected by both ROS-GC1 and S100B antibodies on Western blots (Duda et al., 2002). Biochemical experiments with ROS-GC1 deletion constructs localized an interaction of S100B with the C-terminus of ROS-GC1, aa 731–1054 (Duda et al., 1996a). An EC50 of 395 nM, measured using surface plasmon spectroscopy, was comparable to the EC50 of 800 nM for stimulation of ROS-GC1 by S100B in a biochemical assay (Duda et al., 2002).
Since the aa 733–964 segment containing the putative dimerization and catalytic domains is highly conserved, the less conserved aa 965–1054 segment was subjected to further analyses. First, three ROS-GC1 deletion mutants: Δ965–1054, Δ972–1054, and Δ1016–1054 were analyzed for their responses to S100B. The first mutant was totally unresponsive, the response of the second mutant was only ~25% and that of the third mutant ~50% of the wild-type ROS-GC1 response to S100B. Therefore the entire region aa 965–1054 of ROS-GC1 is important for Ca2+-dependent stimulation by S100B, but the region aa 965–1016 appears to be the most critical (Duda et al., 2002). Second, overlapping peptides encompassing this region were screened for their effects on S100B-dependent stimulation of ROS-GC1 at high Ca2+. Two peptides covering aa regions 952–991 and 1029–1040 prevented ROS-GC1 stimulation by S100B. Finer analysis revealed that aa 962–981 formed the critical binding site of ROS-GC1 with half-maximal binding at 198 nM. A 966RIHVNRS972 motif was obligatory, however, a flanking cluster of four aa residues, R1039RQK1042 formed a low affinity binding site. While not absolutely required for binding, the secondary site was an S100B-modulated transduction site that conferred a twofold increase in maximal activation of ROS-GC1 (Duda et al., 2002).
These studies validated the unique ability of ROS-GC1 to respond not only to low Ca2+ signals mediated by GCAPs but also to high Ca2+ signals mediated by S100B. To analyze the possible interplay of these signals, the aforementioned ROS-GC1 deletion mutants were analyzed for their responses to GCAP1 (Duda et al., 2002) and GCAP2 (Duda et al., 2005). At 10 nM Ca2+, activation of all mutants was identical to that of wild-type ROS-GC1. Thus, the S100B and GCAP1 regulatory sites of ROS-GC1 are distinct and do not interfere with one other. This result was not surprising because the binding site for GCAP1 was previously mapped to the N-terminal part of the ROS-GC1 cytoplasmic domain (Duda et al., 1999; Lange et al., 1999).
The case for GCAP2 was not so clear. Initial mapping had localized the GCAP2 binding site closer to the C-terminus of ROS-GC1 (Krishnan et al., 1998). In reconstitution experiments with the ROS-GC1 deletion mutants (the same as used for mapping the S100B site: vide supra), the Δ965–1054 mutant failed to respond to GCAP2, the Δ972–1045 responded with Vmax of ~50% of that of the wild-type ROS-GC1, and the response of the Δ1016–1054 mutant matched that of the wild-type cyclase, indicating that the functional GCAP2-modulated domain resided within aa 965–1016. Detailed peptide competition assays narrowed down the GCAP2-modulated site to the sequence motif Y965-N981 (Duda et al., 2005). As expected, this site is distinct from the GCAP1 site. It, however, partially overlaps with the S100B-regulatory site. These results provide a hint that, when GCAP1 and S100B are co-expressed, a single ROS-GC1 complex could operate as a bimodal Ca2+ switch. On the other hand, when GCAP2 and S100B are co-expressed, the switch might involve separate populations of ROS-GC1 complexes. Furthermore, identification of the binding sites for the Ca2+ sensors disclosed an intriguing topographical feature of ROS-GC1; GCAP2 and S100B transmit the Ca2+ signals to the catalytic domain of ROS-GC1 from the C-terminal side while GCAP1 does so from a more distant location on the N-terminal side. These intramolecular mechanics are specific to ROS-GC1 and are different from the peptide hormone receptor subfamily, where the signal to the catalytic site migrates solely downstream from a site on the N-terminus located on the opposite side of the membrane. A schematic model of the system is depicted in Figure 2.
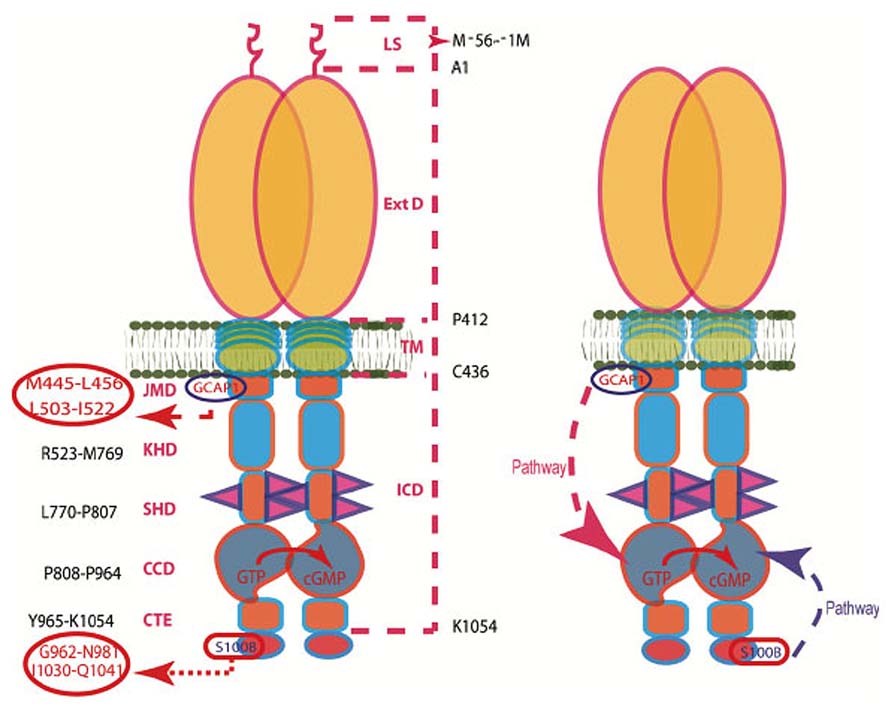
FIGURE 2. The modular nature of ROS-GC1. Left Panel. The dashed lines on the right show the domain boundaries: LS, leader sequence (note: the leader sequence is absent from the mature protein shown in the right panel); ExtD, extracellular domain; TM, transmembrane domain; ICD, intracellular domain. The designated names and the amino acid residues constituting their boundaries are indicated on the left: JMD, juxtamembrane domain housing the indicated GCAP1-binding site; KHD, kinase homology domain; SHD, signaling helix domain; CCD, core catalytic domain; CTE, C-terminal extension housing the S100B-binding site. It is noteworthy that the ICD contains all of the functional domains and that GCAP1 and S100B target sites on opposite sides of the CCD. Right panel. The GCAP1 and S100B signaling pathways within ROS-GC propagate in opposite directions. The trajectory of the GCAP1 pathway is shown with the red dashed arrow. From its origin in the JMD, it passes “downstream” through the structural KHD and SHD in its course to the CCD. The trajectory of the S100B pathway shown with the blue dashed arrow. Originating in the CTE, it directly flows “upstream” to the CCD. The CCD exists as an antiparallel homodimer. Both GCAP1 and S100B signals are translated at the CCD into faster production of cyclic GMP (Modified from Duda et al., 2012).
ROS-GC2 has an ~8x lower affinity for S100B and with S100B bound at high Ca2+, its maximal activation is twofold lower than that of ROS-GC1. Substitution of aa 731–1053 of ROS-GC1 into the corresponding region of ROS-GC2 and vice versa reversed the selectivity (Duda et al., 1998). These results suggest that ROS-GC2 may be an S100B partner of lesser importance than ROS-GC1.
S100B is Expressed in Cones but not in RODS
Guided by the knowledge that ROS-GC and GCAPs are present in photoreceptors, including their outer segments where they serve as critical components of phototransduction (Cuenca et al., 1998; Kachi et al., 1999; reviewed in Pugh et al., 1997; Koch et al., 2010; Sharma and Duda, 2012), and that S100B is also present in photoreceptors (Rambotti et al., 1999; reviewed in: Rambotti et al., 2002), questions were raised as to whether a ROS-GC1 bimodal Ca2+ -regulated system operates in visual transduction and whether it is present in both rods and cones. These issues are being addressed in mouse (e.g., Wen et al., 2012), for which there exist useful knockouts including S100B-/-, GCAP1-/-, GCAP2-/-, GCAP1/GCAP2-/-, ROS-GC1-/-.
Affinity purified S100B antibody recognized on Western blots of mouse photoreceptors, a protein of molecular weight corresponding to S100B. In biochemical assays, guanylate cyclase activity of mouse ROS membranes exhibited bimodal Ca2+ switch behavior; activity decreased as [Ca2+] rose from 10 to 300 nM and then increased as [Ca2+] began to exceed 300 nM. The “inhibitory” phase of the guanylate cyclase activity was consistent with the loss of ROS-GC1 activation by GCAPs upon Ca2+ binding to the latter and the stimulatory phase with Ca2+-induced activation of ROS-GC1 activity by S100B. The increase in ROS-GC activity at low [Ca2+] disappeared in ROS membranes from GCAP1/GCAP2-/- mice, whereas the increase in ROS-GC activity at high [Ca2+] was missing in ROS membranes from S100B-/- mice and from ROS-GC1-/- mice. The significance of the result from ROS-GC1-/- mice will be revealed below. Yet, regulation of ROS-GC1 by S100B was not functionally linked to phototransduction in rods because flash responses from wild-type and S100B-KO rods were indistinguishable (Wen et al., 2012). The possibility that S100B might contribute to the rod photoresponse at high free [Ca2+] outside the normal physiological range was tested and not found.
Detailed histochemical analysis of S100B expression in the mouse retina brought all these observations into accord (Figure 3). As expected, the S100B-KO retina showed no S100B immunoreactivity (Wen et al., 2012), affirming the specificity of the S100B antibody, because photoreceptor outer segments also express S100A1, another member of the S100 family (Rambotti et al., 1999). S100B immunoreactivity was present in wild-type photoreceptors, however, it was not present in all of them. The scattered S100B staining corresponded to that for cone-specific arrestin. On the other hand, in retinas of Nrl-/- mice, all outer segments labeled for S100B as well as for cone arrestin. Because Nrl-/- retina is rod-less and populated exclusively with cone photoreceptors (Mears et al., 2001) these results strongly indicate that among photoreceptors, S100B expression is restricted to cones. Unimodal switch behavior in ROS-GC1-/- outer segments (above) is now easy to understand. In ROS-GC1-/- retinas, cones degenerate but the rods remain intact (Yang et al., 1999). Rods however, do not express S100B, so the only Ca2+-regulated guanylate cyclase activity in ROS-GC1-/- retinas is the GCAPs modulated ROS-GC2 activity.
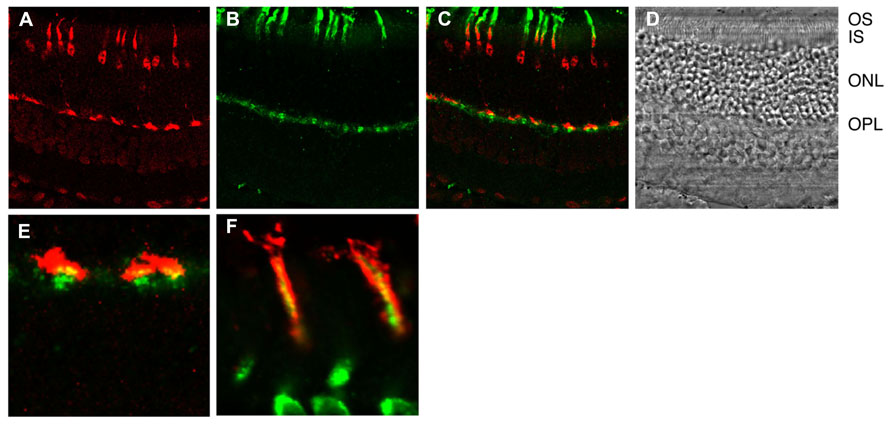
FIGURE 3. Immunhistochemical localization of S100B to cones in the murine retina. (A) Cone arrestin in red. Cone outer segment labeling is overshadowed with by strong labeling of the synapse, nucleus and inner segment. (B) S100B in green. Selected outer segments are labeled intensely. Inner segments and synapses are also labeled. (C) Composite showing that cone arrestin and S100B labeling are restricted to cones. (D) Bright field. OS, outer segments; IS, inner segments; ONL, outer nuclear layer containing nuclei of rods and cones; OPL, outer plexiform layer containing synapses of rods and cones. (E) Composite showing cone synapses at higher magnification. Overlap (yellow) between arrestin and S100B is not perfect because the former is a soluble protein whereas the latter is membrane bound. (F) Section from a different retina that was doubly labeled for cone arrestin in green and S100B in red at high magnification. Conditions were adjusted to optimize the detection of outer segment labeling Courtesy of A. Pertzev, Salus University.
Our ongoing immunohistochemical studies with the Nile Rat (Arvicanthis ansorgei) retina in which 33% of the photoreceptors are cones, in contrast to the 3% cone population in the mouse (Bobu et al., 2006) appear to corroborate the conclusion derived from analyses of murine retinas that S100B co-exists with ROS-GC1 exclusively in the cone outer segments.
At present, the question about the role of the ROS-GC1 bimodal switch in cone phototransduction is still unanswered because cones from S100B-/- mice have yet to be recorded. A model illustrating some of the difficulties in understanding the operation of the bimodal switch is shown in Figure 4. One issue is that in darkness (DARK), Ca2+ will not bind to S100B and activate the switch if [Ca2+]i never exceeds 250 nM. However, the value of 250 nM for [Ca2+]i in darkness was taken from mouse rods (Woodruff et al., 2002) because measurements from mouse cones have not yet been made. Thus the true [Ca2+]i for cones in darkness may be higher. A second issue is that without proper restraint, operation of the bimodal switch at high [Ca2+]i would ignite an explosive positive feedback: increased cyclic GMP production, followed by opening of more cyclic GMP-gated channels, which then allow a greater influx of Ca2+, etc. Cones could accumulate lethal levels of Ca2+ or experience a collapse in ion gradients (DEPOLARIZATION-S100B Mode). Indeed, genetic mutations that result in a hyperactive ROS-GC complex cause the photoreceptors to degenerate (Olshevskaya et al., 2002; Zägel and Koch, 2014).
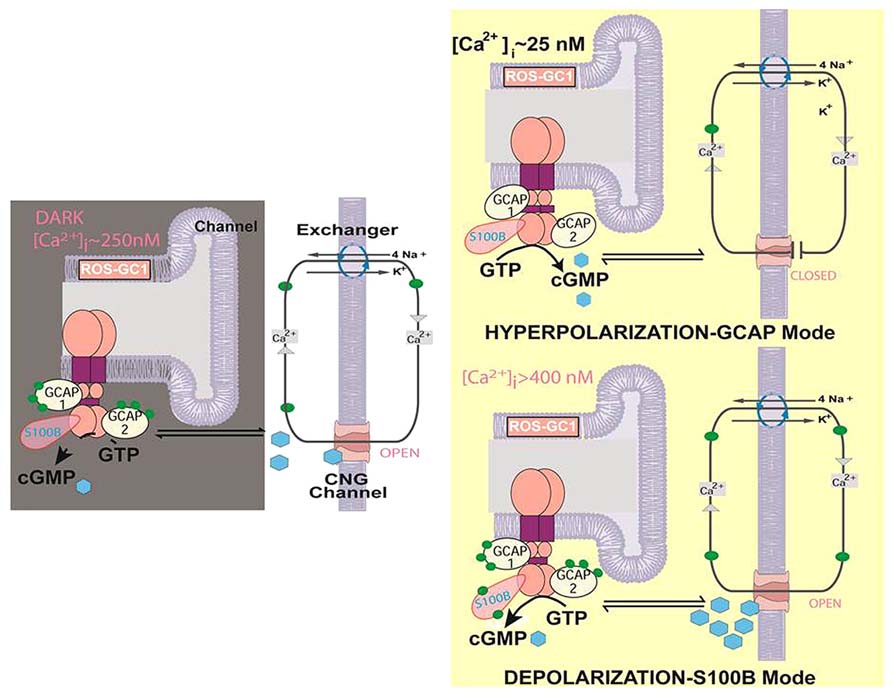
FIGURE 4. Two modes of ROS-GC1 modulation by [Ca2+]i in cones. Dark state [left]. At [Ca2+]i ~250 nM Ca2+ sensors: GCAP1, GCAP2, S100B are ROS-GC1 bound; GCAP1 and GCAP2 are [Ca2+]i bound; ROS-GC1 is in the basal state. Cyclic GMP generated keeps a fraction of CNG channels open allowing an influx of Na+ and Ca2+. In this graphical simplification, GCAP2 and S100B are shown bound to the same ROS-GC dimer but it is more likely that these two Ca2+ sensors bind separate dimers. Hyperpolarization-GCAP mode [Top right]. LIGHT triggers activation of phosphodiesterase and hydrolysis of cyclic GMP. The decrease in cyclic GMP causes CNG channels to close, preventing influx of Na+ and Ca2+ and hyperpolarizing the outer segment plasma membrane. Extrusion of Ca2+ by the Na+/Ca2+, K+ exchanger lowers [Ca2+]i from ~250 nM to about 25 nM. The decline causes GCAPs to stimulate ROS-GC1. Depolarization-S100B mode [Bottom right]. [Ca2+]i levels rise when CNG channels open. With K1/2 of 400 nM, S100B captures Ca2+, triggering activation of ROS-GC1. Cyclic GMP formed could open many more CNG channels, greatly increasing the influx of Na+ and Ca2+. [Ca2+]i levels rise still further because extrusion of Ca2+ through Na+/Ca2+, K+ exchanger slows as the ion gradients collapse. The cone outer segment may lose the ability to maintain a membrane potential (Modified from Wen et al., 2012).
The Bimodal Switch Exists at the Photoreceptor-Bipolar Cell Synapse
Immunohistochemical studies also place ROS-GC1, GCAP1 and S100B in the synaptic layers of the retina (Liu et al., 1994; Cooper et al., 1995; Duda et al., 2002; Venkataraman et al., 2003). To test whether the ROS-GC1 transduction system utilizes a Ca2+ bimodal switch at the photoreceptor-bipolar cell synapse (presumably the cone to bipolar synapse, see Figure 3), bovine retinal synaptosomes (P1) were prepared and analyzed. Purity of the preparation was ascertained by Western blotting with rhodopsin kinase antibody. No immunoreactivity for rhodopsin kinase was detected, assuring that the preparation was not contaminated with ROSs, the original residential site of the ROS-GC1. Western blots were positive for ROS-GC1, GCAP1 and S100B demonstrating that indeed the synaptosomal fraction contained all the components of the ROS-GC1 bimodal system and was suitable for functional analyses.
To be physiologically relevant, the components of the ROS-GC1 bimodal system, ROS-GC1, GCAP1 and S100B must reside in proximity and not, e.g., in different populations of synaptosomes. Because the Western analyses described above did not provide information about the proximity of ROS-GC1, GCAP1 and S100B molecules, immunohistochemical analyses (Duda et al., 2002; Venkataraman et al., 2003) and cross-linking experiments were performed.
Cryosections of bovine retina were doubly labeled with antibodies against GCAP1 or ROS-GC1 and SV2, a synaptic vesicle protein that strongly stains photoreceptor termini (Buckley and Kelly, 1985). The results revealed co-expression of ROS-GC1 and GCAP1 in cone synaptic termini (Venkataraman et al., 2003). Similarly, co-localization of ROS-GC1 and S100B was shown (Duda et al., 2002).
Cross-linking experiments with a non-cleavable imidoester confirmed the proximity of ROS-GC1 and GCAP1 in the synaptosomal fraction (Venkataraman et al., 2003). Without the cross-linker, the ROS-GC1 and GCAP1 antibodies identified separate bands at ~120 kDa and ~21 kDa, respectively, corresponding to the appropriate monomers. But in presence of the cross-linker, both GCAP1 and ROS-GC1 antibodies identified a common immunoreactive band at ~250 kDa, the approximate size for a ROS-GC1 dimer-GCAP1 complex. The results demonstrated that ROS-GC1 and GCAP1, indeed, resided in proximity. Because ROS-GC1 resides with GCAP1 in cone synaptic terminals and ROS-GC1 also resides with S100B, it was inferred that all three components of the bimodal system co-reside in the photoreceptor synaptic terminals, specifically cone synaptic terminals.
Functionality of the ROS-GC1 bimodal switch in the P1 fraction was determined by measuring guanylate cyclase activity at Ca2+ concentrations ranging from 10 nM to 10 μM. Below 200 nM, Ca2+ elicited a dose-dependent decrease in guanylate cyclase activity with an “inhibitory” value (IC50) for Ca2+ of 100 nM. But at higher concentrations, ROS-GC activity began to climb with an EC50 of 0.8 μM. Side by side analysis of photoreceptor outer segment and P1 membranes demonstrated that the cyclic GMP synthetic activities were inhibited identically by free Ca2+ with an IC50 of 100 nM.
That GCAP1 was involved in the “inhibitory” phase of ROS-GC1 activity was confirmed by peptide competition experiments. A sequence motif L503–I522 in ROS-GC1 is critical and specific for the cyclase activation by GCAP1 (Lange et al., 1999). The core motif within this domain consists of D507–R518. Analysis of the P1 and photoreceptor outer segments membranes in parallel demonstrated that the incremental concentrations of the L503–I522 and D507–R518 peptides at 10 nM Ca2+ inhibited their guanylate cyclase activities with the same dose-dependence. A control peptide of L503–I522 with a scrambled sequence had no effect on GCAP-dependent ROS–GC1 activity. Both fractions showed a reversal of the peptide (D507–R518)-dependent inhibition upon the addition of an excess of GCAP1 (Venkataraman et al., 2003). Thus Ca2+ signaling in the synapse region is modulated by GCAP1 and S100B. GCAP1 has the higher affinity for Ca2+ and upon binding, lowers ROS-GC1 activity. S100B captures the high Ca2+ signal and upon binding, stimulates ROS-GC1.
A Role in Signal Processing in the Retina?
ERG recordings from wild-type and S100B-/- mice provided the first clues as to the physiological significance of S100B in retinal function (Wen et al., 2012). The ERG is a field potential across the retina generated by the electrical activity of all cells, including that of rods and cones (reviewed in: Peachey and Ball, 2003; Weymouth and Vingrys, 2008). The corneal negative a-wave, which sums the photocurrent responses of rods, is normal in the S100B-KO mice because S100B is not present in rods. The corneal positive b-wave, generated mainly by ON-bipolar cells, displays slower kinetics in S100B-/- mice; it peaks later and takes longer to recover. Oscillatory potentials that arise from the activity of inner retinal neurons are smaller and there are disturbances in their timing. Gaps in our knowledge about what S100B is doing in the cone outer segment, the cone synapses and for that matter, in other retinal neurons preclude any attempt to interpret the changes in the ERG at this time.
Future Directions
The composition and the operational principles of cones and rods differ in ways that support their specialized visual functions: extremely high sensitivity in rods, rapid responsiveness over a wide dynamic range in cones. In addition to the GCAPs, S100B serves as an additional Ca2+ sensor component of ROS-GC1 in cones. Future tasks will be to decode this signal transduction system at the molecular and physiological levels, to link it with cone-related dystrophies and to design therapeutic options. Progress would be facilitated if several important objectives were to be met. (1) A suitable mammalian model with abundant cones needs to be selected. No such mouse model is available at present. Through genetic manipulation, development of the rods has been suppressed in Nrl-/- mice and their retinas are cone-exclusive. But these cones may not be exact copies of the natural, although biochemically and physiologically, they appear to be very close (Nikonov et al., 2005, 2006). One alternative is the Nile rat (A. ansorgei), whose retina contains about 30% cones in contrast to the 3% population in the mouse (Bobu et al., 2006). (2) The molecular architecture of the ROS-GC complex needs to be understood. Which combinations of Ca2+ sensor proteins are possible in the complex? Which actually occur? How are their relative levels within different cell types regulated? (3) Ca2+-modulated signal transduction by the ROS-GC complex needs to be defined at a molecular level. For the S100B native to cones, what are the kinetic parameters of the S100B transduction switch? By what mechanism does S100B sense Ca2+ and how does it effect a change in the catalytic domain of ROS-GC? (4) The physiological role of S100B in the cone photoresponse needs to be assessed. Does it accelerate the recovery of cone response and increase the dark current? What limits S100B stimulated ROS-GC activity at high Ca2+? Does S100B increase the rate of vesicular release of neurotransmitter at the synapse? S100A1 is also expressed in outer segments, what is its function? (5) S100B is expressed in inner retinal neurons but nothing is known about what it does there. (6) S100B protein does not fit in the presently classified NCS protein family, sharing only 15% sequence identity and containing only two EF-hands. Therefore, the NCS family needs to be expanded to include S100B as a branch.
Conflict of Interest Statement
The authors declare that the research was conducted in the absence of any commercial or financial relationships that could be construed as a potential conflict of interest.
Acknowledgments
The authors gratefully acknowledge the continuous past support by the numerous USPHS award from the National Institutes of Health, the beginning award from the National Science Foundation and the Damon Runyon Walter Winchell Cancer Fund and the Howe Laboratory Endowment of the Massachusetts Eye and Ear Infirmary.
References
Baehr, W., Karan, S., Maeda, T., Luo, D. G., Li, S., Bronson, J. D., et al. (2007). The function of guanylate cyclase 1 and guanylate cyclase 2 in rod and cone photoreceptors. J. Biol. Chem. 282, 8837–8847. doi: 10.1074/jbc.M610369200
Bobu, C., Craft, C. M., Masson-Pevet, M., and Hicks, D. (2006). Photoreceptor organization and rhythmic phagocytosis in the nile rat Arvicanthis ansorgei: a novel diurnal rodent model for the study of cone pathophysiology. Invest. Ophthalmol. Vis. Sci. 47, 3109–3118. doi: 10.1167/iovs.05-1397
Buckley, K., and Kelly, R. B. (1985). Identification of a transmembrane glycoprotein specific for secretory vesicles of neural and endocrine cells. J. Cell Biol. 100, 1284–1294. doi: 10.1083/jcb.100.4.1284
Chen, C. K., Inglese, J., Lefkowitz, R. J., and Hurley, J. B. (1995). Ca(2+)-dependent interaction of recoverin with rhodopsin kinase. J. Biol. Chem. 270, 18060–18066. doi: 10.1074/jbc.270.30.18060
Chinkers, M., Garbers, D. L., Chang, M. S., Lowe, D. G., Chin, H. M., Goeddel, D. V., et al. (1989). A membrane form of guanylate cyclase is an atrial natriuretic peptide receptor. Nature 338, 78–83. doi: 10.1038/338078a0
Cooper, N., Liu, L., Yoshida, A., Pozdnyakov, N., Margulis, A., and Sitaramayya A. (1995). The bovine rod outer segment guanylate cyclase, ROS-GC, is present in both outer segment and synaptic layers of the retina. J. Mol. Neurosci. 6, 211–222. doi: 10.1007/BF02736766
Cuenca, N., Lopez, S., Howes, K., and Kolb, H. (1998). The localization of guanylyl cyclase-activating proteins in the mammalian retina. Invest. Ophthalmol. Vis. Sci. 39, 1243–1250.
Dizhoor, A. M., Olshevskaya, E. V., Henzel, W. J., Wong, S. C., Stults, J. T., Ankoudinova, I., et al. (1995). Cloning, sequencing, and expression of a 24-kDa Ca(2+)-binding protein activating photoreceptor guanylyl cyclase. J. Biol. Chem. 270, 25200–25206. doi: 10.1074/jbc.270.42.25200
Dizhoor, A. M., Ray, S., Kumar, S., Niemi, G., Spencer, M., Brolley, D., et al. (1991). Recoverin: a calcium sensitive activator of retinal rod guanylate cyclase. Science 251, 915–918. doi: 10.1126/science.1672047
Duda, T., Fik-Rymarkiewicz, E., Venkataraman, V., Krishnan, R., Koch, K. W., and Sharma, R. K. (2005). The calcium-sensor guanylate cyclase activating protein type 2 specific site in rod outer segment membrane guanylate cyclase type 1. Biochemistry 44, 7336–7345. doi: 10.1021/bi050068x
Duda, T., Goraczniak, R. M., and Sharma, R. K. (1996a). Molecular characterization of S100A1-S100B protein in retina and its activation mechanism of bovine photoreceptor guanylate cyclase. Biochemistry 35, 6263–6266. doi: 10.1021/bi960007m
Duda, T., Goraczniak, R., Surgucheva, I., Rudnicka-Nawrot, M., Gorczyca, W. A., Palczewski, K., et al. (1996b). Calcium modulation of bovine photoreceptor guanylate cyclase. Biochemistry 35, 8478–8482. doi: 10.1021/bi960752z
Duda, T., Goraczniak, R. M., Pozdnyakov, N., Sitaramayya, A., and Sharma, R. K. (1998). Differential activation of rod outer segment membrane guanylate cyclases, ROS-C1 and ROS-GC2, by CD-GCAP and indentification of signaling domain. Biochem. Biophys. Res. Commun. 242, 118–122. doi: 10.1006/bbrc.1997.7921
Duda, T., Goraczniak, R. M., and Sharma, R. K. (1991). Site-directed mutational analysis of a membrane guanylate cyclase cDNA reveals the atrial natriuretic factor signaling site. Proc. Natl. Acad. Sci. U.S.A. 88, 7882–7886. doi: 10.1073/pnas.88.17.7882
Duda, T., Goraczniak, R. M., Sitaramayya, A., and Sharma, R. K. (1993). Cloning and expression of an ATP-regulated human retina C-type natriuretic factor receptor guanylate cyclase. Biochemistry 32, 1391–1395. doi: 10.1021/bi00057a001
Duda, T., Koch, K. W., Venkataraman, V., Lange, C., Beyermann, M., and Sharma, R. K. (2002). Ca2+ sensor S100beta-modulated sites of membrane guanylate cyclase in the photoreceptor-bipolar synapse. EMBO J. 21, 2547–2556. doi: 10.1093/emboj/21.11.2547
Duda, T., Pertzev, A., and Sharma, R. K. (2012). Differential Ca2+ sensor guanylate cyclase activating protein modes of photoreceptor rod outer segment membrane guanylate cyclase signaling. Biochemistry 51, 4650–4657. doi: 10.1021/bi300572w
Duda, T., Venkataraman, V., Goraczniak, R., Lange, C., Koch, K. W., and Sharma, R. K. (1999). Functional consequences of a rod outer segment membrane guanylate cyclase (ROS-GC1) gene mutation linked with Leber’s congenital amaurosis. Biochemistry 38, 509–515. doi: 10.1021/bi9824137
Frins, S., Bönigk, W., Müller, F., Kellner, R., and Koch, K. W. (1996). Functional characterization of a guanylyl cyclase-activating protein from vertebrate rods. Cloning, heterologous expression, and localization. J. Biol. Chem. 271, 8022–8027. doi: 10.1074/jbc.271.14.8022
Goraczniak, R. M., Duda, T., and Sharma, R. K. (1997). Structural and functional characterization of a second subfamily member of the calcium-modulated bovine rod outer segment membrane guanylate cyclase, ROS-GC2. Biochem. Biophys. Res. Commun. 234, 666–670. doi: 10.1006/bbrc.1997.6579
Goraczniak, R. M., Duda, T., Sitaramayya, A., and Sharma, R. K. (1994). Structural and functional characterization of the rod outer segment membrane guanylate cyclase. Biochem. J. 302, 455–461.
Gorczyca, W. A., Polans, A. S., Surgucheva, I. G., Subbaraya, I., Baehr, W., and Palczewski, K. (1995). Guanylyl cyclase activating protein. A calcium-sensitive regulator of phototransduction. J. Biol. Chem. 270, 22029–22036. doi: 10.1074/jbc.270.37.22029
Hayashi, F., and Yamazaki, A. (1991). Polymorphism in purified guanylate cyclase from vertebrate rod photoreceptors. Proc. Natl. Acad. Sci. U.S.A. 88, 4746–4750. doi: 10.1073/pnas.88.11.4746
Horio, Y., and Murad, F. (1991a). Solubilization of guanylyl cyclase from bovine rod outer segments and effects of lowering Ca2+ and nitro compounds. J. Biol. Chem. 266, 3411–3415.
Horio, Y., and Murad, F. (1991b). Purification of guanylyl cyclase from rod outer segments. Biochim. Biophys. Acta 1133, 81–88. doi: 10.1016/0167-4889(91)90244-R
Hurley, J. B., Dizhoor, A. M., Ray, S., and Stryer, L. (1993). Recoverin’s role: conclusion withdrawn. Science 260, 740. doi: 10.1126/science.8097896
Kachi, S., Nishizawa, Y., Olshevskaya, E., Yamazaki, A., Miyake, Y., Wakabayashi, T., et al. (1999). Detailed localization of photoreceptor guanylate cyclase activating protein-1 and -2 in mammalian retinas using light and electron microscopy. Exp. Eye Res. 68, 465–473. doi: 10.1006/exer.1998.0629
Kawamura, S. (1999). Calcium-dependent regulation of rhodopsin phosphorylation. Novartis Found. Symp. 224, 208–218; discussion 218–224.
Klenchin, V. A., Calvert, P. D., and Bownds, M. D. (1995). Inhibition of rhodopsin kinase by recoverin. Further evidence for a negative feedback system in phototransduction. J. Biol. Chem. 270, 16147–16152. doi: 10.1074/jbc.270.41.24127
Kligman, D., and Marshak, D. R. (1985). Purification and characterization of a neurite extension factor from bovine brain. Proc. Natl. Acad. Sci. U.S.A. 82, 7136–7139. doi: 10.1073/pnas.82.20.7136
Koch, K.-W. (1991). Purification and identification of photoreceptor guanylate cyclase. J. Biol. Chem. 266, 8634–8637.
Koch, K. W., Duda, T., and Sharma, R. K. (2010). Ca2+-modulated vision-linked ROS-GC guanylate cyclase transduction machinery. Mol. Cell. Biochem. 334, 105–115. doi: 10.1007/s11010-009-0330-z
Koch, K. W., and Stryer, L. (1988). Highly cooperative feedback control of retinal rod guanylate cyclase by calcium ions. Nature 334, 64–66. doi: 10.1038/334064a0
Koller, K. J., Lowe, D. G., Bennett, G. L., Minamino, N., Kangawa, K., Matsuo, H., et al. (1991). Selective activation of the B natriuretic peptide receptor by C-type natriuretic peptide (CNP). Science 252, 120–123. doi: 10.1126/science.1672777
Koutalos, Y., Nakatani, K., Tamura, T., and Yau, K. W. (1995). Characterization of guanylate cyclase activity in single retinal rod outer segments. J. Gen. Physiol. 106, 863–890. doi: 10.1085/jgp.106.5.863
Krishnan, A., Goraczniak, R. M., Duda, T., and Sharma, R. K. (1998). Third calcium-modulated rod outer segment membrane guanylate cyclase transduction mechanism. Mol. Cell. Biochem. 178, 251–259. doi: 10.1023/A:1006860018300
Lange, C., Duda, T., Beyermann, M., Sharma, R. K., and Koch, K. W. (1999). Regions in vertebrate photoreceptor guanylyl cyclase ROS-GC1 involved in Ca(2+)-dependent regulation by guanylyl cyclase-activating protein GCAP-1. FEBS Lett. 460, 27–31. doi: 10.1016/S0014-5793(99)01312-5
Liu, X., Seno, K., Nishizawa, Y., Hayashi, F., Yamazaki, A., Matsumoto, H., et al. (1994). Ultrastructural localization of retinal guanylate cyclase in human and monkey retinas. Exp. Eye Res. 59, 761–768. doi: 10.1006/exer.1994.1162
Lowe, D. G., Dizhoor, A. M., Liu, K., Gu, Q., Spencer, M., Laura, R., et al. (1995). Cloning and expression of a second photoreceptor-specific membrane retina guanylyl cyclase (RetGC), RetGC-2. Proc. Natl. Acad. Sci. U.S.A. 92, 5535–5539.
Margulis, A., Goraczniak, R. M., Duda, T., Sharma, R. K., and Sitaramayya, A. (1993). Structural and biochemical identity of retinal rod outer segment membrane guanylate cyclase. Biochem. Biophys. Res. Commun. 194, 855–861. doi: 10.1006/bbrc.1993.1900
Margulis, A., Pozdnyakov, N., and Sitaramayya, A. (1996). Activation of bovine photoreceptor guanylate cyclase by S100 proteins. Biochem. Biophys. Res. Commun. 218, 243–247. doi: 10.1006/bbrc.1996.0043
Mears, A. J., Kondo, M., Swain, P. K., Takada, Y., Bush, R. A., Saunders, T. L., et al. (2001). Nrl is required for rod photoreceptor development. Nat. Genet. 29, 447–452. doi: 10.1038/ng774
Nikonov, S. S., Daniele, L. L., Zhu, X., Craft, C. M., Swaroop, A., and Pugh, E. N. Jr. (2005). Photoreceptors of Nrl-/- mice coexpress functional S- and M-cone opsins having distinct inactivation mechanisms. J. Gen. Physiol. 125, 287–304. doi: 10.1085/jgp.200409208
Nikonov, S. S., Kholodenko, R., Lem, J., and Pugh, E. N. Jr. (2006). Physiological features of the S- and M-cone photoreceptors of wild-type mice from single-cell recordings. J. Gen. Physiol. 127, 359–374. doi: 10.1085/jgp.200609490
Olshevskaya, E. V., Ermilov, A. N., and Dizhoor, A. M. (2002). Factors that affect regulation of cGMP synthesis in vertebrate photoreceptors and their genetic link to human retinal degeneration. Mol. Cell. Biochem. 230, 139–147. doi: 10.1023/A:1014248208584
Palczewski, K., Subbaraya, I., Gorczyca, W. A., Helekar, B. S., Ruiz, C. C., Ohguro, H., et al. (1994). Molecular cloning and characterization of retinal photoreceptor guanylyl cyclase-activating protein. Neuron 13, 395–404. doi: 10.1016/0896-6273(94)90355-7
Paul, A. K., Marala, R. B., Jaiswal, R. K., and Sharma, R. K. (1987). Coexistence of guanylate cyclase and atrial natriuretic factor receptor in a 180-kD protein. Science 235, 1224–1226. doi: 10.1126/science.2881352
Peachey, N. S., and Ball, S. L. (2003). Electrophysiological analysis of visual function in mutant mice. Doc. Ophthalmol. 107, 13–36. doi: 10.1023/A:1024448314608
Pozdnyakov, N., Goraczniak, R., Margulis, A., Duda, T., Sharma, R. K., Yoshida, A., et al. (1997). Structural and functional characterization of retinal calcium-dependent guanylate cyclase activator protein (CD-GCAP): identity with S100beta protein. Biochemistry 36, 14159–14166. doi: 10.1021/bi971792l
Pozdnyakov, N., Yoshida, A., Cooper, N. G., Margulis, A., Duda, T., Sharma, R. K., et al. (1995). A novel calcium-dependent activator of retinal rod outer segment membrane guanylate cyclase. Biochemistry 34, 14279–14283. doi: 10.1021/bi00044a002
Pugh, E. N. Jr., Duda, T., Sitaramayya, A., and Sharma, R. K. (1997). Photoreceptor guanylate cyclases: a review. Biosci. Rep. 17, 429–473. doi: 10.1023/A:1027365520442
Rambotti, M. G., Giambanco, I., Spreca, A., and Donato, R. (1999). S100B and S100A1 proteins in bovine retina:their calcium-dependent stimulation of a membrane-bound guanylate cyclase activity as investigated by ultracytochemistry. Neuroscience 92, 1089–1101. doi: 10.1016/S0306-4522(99)00074-3
Rambotti, M. G., Spreca, A., Giambanco, I., Sorci, G., and Donato, R. (2002). Ultracytochemistry as a tool for the study of the cellular and subcellular localization of membrane-bound guanylate cyclase (GC) activity. Applicability to both receptor-activated and receptor-independent GC activity. Mol. Cell. Biochem. 230, 85–96. doi: 10.1023/A:1014275520879
Sharma, R. K. (1988). Guanylate cyclase and the atrial natriuretic factor receptor. Response to Waldman, S. A., Leitman, D. C., Anderson, J., and Murad, F. Science 240, 805–806. doi: 10.1126/science.240.4853.805-a
Sharma, R. K. (2010). Membrane guanylate cyclase is a beautiful signal transduction machine: overview. Mol.Cell. Biochem. 334, 3–36. doi: 10.1007/s11010-009-0336-6
Sharma, R. K., and Duda, T. (2012). Ca(2+)-sensors and ROS-GC: interlocked sensory transduction elements: a review. Front. Mol. Neurosci. 5:42. doi: 10.3389/fnmol.2012.00042
Shyjan, A. W., de Sauvage, F. J., Gillett, N. A., Goeddel, D. V., and Lowe, D. G. (1992). Molecular cloning of a retina-specific membrane guanylyl cyclase. Neuron 9, 727–737. doi: 10.1016/0896-6273(92)90035-C
Subbaraya, I., Ruiz, C. C., Helekar, B. S., Zhao, X., Gorczyca, W. A., Pettenati, M. J., et al. (1994). Molecular characterization of human and mouse photoreceptor guanylate cyclase-activating protein (GCAP) and chromosomal localization of the human gene. J. Biol. Chem. 269, 31080–31089.
Venkataraman, V., Duda, T., Vardi, N., Koch, K. W., and Sharma, R. K. (2003). Calcium-modulated guanylate cyclase transduction machinery in the photoreceptor–bipolar synaptic region. Biochemistry 42, 5640–5648. doi: 10.1021/bi034025x
Wen, X. H., Duda, T., Pertzev, A., Venkataraman, V., Makino, C. L., and Sharma, R. K. (2012). S100B serves as a Ca(2+) sensor for ROS-GC1 guanylate cyclase in cones but not in rods of the murine retina. Cell. Physiol. Biochem. 29, 417–430. doi: 10.1159/000338496
Weymouth, A. E., and Vingrys, A. J. (2008). Rodent electroretinography: methods for extraction and interpretation of rod and cone responses. Prog. Retin. Eye Res. 27, 1–44. doi: 10.1016/j.preteyeres.2007.09.003
Woodruff, M. L., Sampath, A. P., Matthews, H. R., Krasnoperova, N. V., Lem, J., and Fain, G. L. (2002). Measurement of cytoplasmic calcium concentration in the rods of wild-type and transducin knock-out mice. J. Physiol. 542(Pt 3), 843–854. doi: 10.1113/jphysiol.2001.013987
Yang, R. B., Robinson, S. W., Xiong, W. H., Yau, K. W., Birch, D. G., and Garbers, D. L. (1999). Disruption of a retinal guanylyl cyclase gene leads to cone-specific dystrophy and paradoxical rod behavior. J. Neurosci. 19, 5889–5897.
Keywords: ROS-GC guanylate cyclase, cyclic GMP, phototransduction, cones, S100B
Citation: Sharma RK, Makino CL, Hicks D and Duda T (2014) ROS-GC interlocked Ca2+-sensor S100B protein signaling in cone photoreceptors: review. Front. Mol. Neurosci. 7:21. doi: 10.3389/fnmol.2014.00021
Received: 20 December 2013; Accepted: 05 March 2014;
Published online: 26 March 2014.
Edited by:
Wolfgang Baehr, University of Utah, USAReviewed by:
Karl-Wilhelm Koch, Carl von Ossietzky University Oldenburg, GermanyWolfgang Baehr, University of Utah, USA
Copyright © 2014 Sharma, Makino, Hicks and Duda. This is an open-access article distributed under the terms of the Creative Commons Attribution License (CC BY). The use, distribution or reproduction in other forums is permitted, provided the original author(s) or licensor are credited and that the original publication in this journal is cited, in accordance with accepted academic practice. No use, distribution or reproduction is permitted which does not comply with these terms.
*Correspondence: Rameshwar K. Sharma and Teresa Duda, Research Divisions of Biochemistry and Molecular Biology, The Unit of Regulatory and Molecular Biology, Salus University, 8360 Old York Road, Elkins Park, PA 19027, USA e-mail: rsharma@salus.edu; tduda@salus.edu;
Clint L. Makino, Department of Ophthalmology, Massachusetts Eye and Ear Infirmary, Harvard Medical School, 234 Charles Street, Boston, MA 02114, USA e-mail: Clint_Makino@meei.harvard.edu