Behavioral and neurophysiological study of olfactory perception and learning in honeybees
- Evolution, Genomes and Speciation Lab, Centre National de la Recherche Scientifique, Gif-sur-Yvette, France
The honeybee Apis mellifera has been a central insect model in the study of olfactory perception and learning for more than a century, starting with pioneer work by Karl von Frisch. Research on olfaction in honeybees has greatly benefited from the advent of a range of behavioral and neurophysiological paradigms in the Lab. Here I review major findings about how the honeybee brain detects, processes, and learns odors, based on behavioral, neuroanatomical, and neurophysiological approaches. I first address the behavioral study of olfactory learning, from experiments on free-flying workers visiting artificial flowers to laboratory-based conditioning protocols on restrained individuals. I explain how the study of olfactory learning has allowed understanding the discrimination and generalization ability of the honeybee olfactory system, its capacity to grant special properties to olfactory mixtures as well as to retain individual component information. Next, based on the impressive amount of anatomical and immunochemical studies of the bee brain, I detail our knowledge of olfactory pathways. I then show how functional recordings of odor-evoked activity in the brain allow following the transformation of the olfactory message from the periphery until higher-order central structures. Data from extra- and intracellular electrophysiological approaches as well as from the most recent optical imaging developments are described. Lastly, I discuss results addressing how odor representation changes as a result of experience. This impressive ensemble of behavioral, neuroanatomical, and neurophysiological data available in the bee make it an attractive model for future research aiming to understand olfactory perception and learning in an integrative fashion.
Introduction
Chemical molecules, especially volatile ones, are the vessel of crucial information that may determine an animal’s eventual survival and reproductive success. Perhaps for this reason, the sense of chemoreception is ubiquitously represented in the animal kingdom (Ache and Young, 2005). The role of the olfactory system is to decode the complex eddies of molecules in the environment and shape them into pieces of relevant information that will allow the animal to make decisions and engage in adapted behaviors. Major tasks of the olfactory system are for instance the identification of food sources, the detection of possible dangers (such as fire or predators), the recognition of potential mates as well as allowing social interactions. How the nervous system operates this transformation from the detection of chemical molecules via the formation of neural representations until the creation of percepts has been the focus of intense research especially in vertebrates (Lledo et al., 2005; Mori et al., 2006; Leon and Johnson, 2009; Mandairon and Linster, 2009) and in insects (Galizia and Menzel, 2001; Laurent, 2002; Galizia, 2008; Masse et al., 2009). A general finding of these studies is that the basic rules underlying olfactory processing in these different classes of animals are highly similar (Hildebrand and Shepherd, 1997; Ache and Young, 2005). For the most part, this resemblance is thought to result from evolutionary convergence due to similar constraints (Eisthen, 2002).
Olfaction consists in a series of transformations from the chemical world of odor molecules into spatiotemporal patterns of neural activity in the animal’s brain, eventually giving rise to a perceptual odor representation. Odor molecules exist in a myriad of chemical compositions, three-dimensional shapes, and vibration properties, to name but a few of their characteristics. They cannot be easily described based on simple dimensions like the wavelength and intensity of stimulus light when studying color vision. Therefore, only multiple descriptors can adequately describe an odorant molecule. In olfaction, the first transformation is thus the detection of particular features of the molecules by dedicated receptor (and associated) proteins, leading through a transduction of the signal to the activation of a subset of receptor cells (Touhara and Vosshall, 2009). This combinatorial code will then be conveyed to a series of structures in the brain and will undergo intense processing leading to a reformatting of the odor representation that will allow the extraction of the most relevant information for the system (Laurent, 2002; Kay and Stopfer, 2006). This processing will then give rise to a perceptual representation used for behavioral decision, and may link odor quality with hedonic value and learned relationships between odor and probable outcomes.
For a century now, the honeybee Apis mellifera L. has been a key insect model in which behavioral, neuroanatomical, and neurophysiological approaches have been performed to unravel the basis of olfaction and olfactory learning. Honeybees are social insects which present a wide range of behaviors relying on olfaction both within and outside of the colony (Winston, 1987; Seeley, 1995). Moreover, the study of olfaction is easily amenable to the laboratory, since dedicated protocols have been developed in which bees show rapid and robust odor learning abilities (Menzel, 1999; Giurfa, 2007). In addition, the olfactory pathway of the honeybee brain has been extensively described (Kenyon, 1896; Mobbs, 1982; Strausfeld, 2002; Kirschner et al., 2006) and the bee brain is easily accessible to neurophysiological experiments like electrophysiological or optical imaging recordings (Galizia and Menzel, 2001; Sandoz et al., 2007). We will discuss in turn these different aspects.
Olfactory Behavior in the Honeybee
Role of Pheromones in Social Life
Honeybees employ a rich repertoire of pheromones to ensure intraspecific communication in many behavioral contexts (Free, 1987; Slessor et al., 2005; Sandoz et al., 2007). The social organization of a honeybee colony is determined by chemical signals produced by the queen, but also by workers. Most honeybee pheromones are complex blends of many substances which are most effective when all components are present in appropriate ratios in the blend. The most important pheromonal components, which were sometimes used in olfactory learning experiments, are detailed below.
The queen, the only fertile female in the colony, communicates her presence mostly by means of a mixture of substances released from her mandibular glands. The queen mandibular pheromone (QMP) was originally considered to be a unique substance, 9-oxo-(E)-2-decenoic acid (9-ODA) (Barbier and Lederer, 1960; Butler et al., 1962), but later studies revealed that the actual pheromone contains several additional components (Slessor et al., 1988; Keeling et al., 2003). The queen pheromone reinforces social cohesion, by attracting workers and enticing them to groom the queen. It also has a physiological effect on workers, inhibiting their ovarian development (Hoover et al., 2003) and modifying gene expression (Grozinger et al., 2003). An interesting aspect of this pheromone is that it acts on different receivers. The queen component 9-ODA thus also acts on males (drones) and plays a crucial role for in-flight mating, attracting them from as far as 60 m (Free, 1987).
The second major source of pheromones is workers, who perform different tasks depending on their age (Winston, 1987). Aggregation pheromones are used by workers to mark and elicit attraction of other workers to important locations (profitable food source, potential nest site, etc.). This pheromone is a complex blend comprising many volatiles among which geraniol and citral are principal components (Pickett et al., 1980). On the other hand, alarm pheromones are released when confronting potential enemies (Breed et al., 2004). The main alarm pheromone is released near the sting and consists of more than 40 highly volatile compounds, among which the major component isopentyl acetate (IPA; Boch et al., 1962; Collins and Blum, 1982; Pickett et al., 1982). Release of this pheromone attracts other bees and causes them to sting and attack. Another alarm pheromone, 2-heptanone, is released by workers’ mandibular glands (Shearer and Boch, 1965) and exerts a repellent action on potential intruders and robbers from other hives. Additionally, it is used by foragers to mark recently depleted flowers to avoid immediate revisit (Giurfa and Núñez, 1992).
Role of Floral Odors in Food Search
When reaching 2–3 weeks of age, workers engage in foraging for nectar or pollen outside the hive (Seeley, 1982). Honeybees are generalist pollinators and are not bound to a limited number of plants for gathering food. However, at the individual level, they are “flower constant,” memorizing the features of a given floral species, and exploiting it as long as profitable (Grant, 1950; Chittka et al., 1999). Floral cues include color, odor, shape, and texture, but among those, odors play the most prominent role, being most readily associated with nectar or pollen reward (von Frisch, 1967; Menzel et al., 1993). The scent of a flower is a mixture of many volatile compounds that varies with respect to genotype, stage of development, and local environmental conditions (Pham-Delègue et al., 1989; Dobson, 1994; Dudareva and Pichersky, 2000). Flowers of the same plant may show differences in volatile compounds according to the time of day and with respect to their pollination status (Tollsten and Bergström, 1989; Schiestl et al., 1997). To maximize their profit from foraging, honeybees have to show good olfactory discrimination capacity. In other words, they have to be able to distinguish between fine differences in the volatile emissions of the visited flowers, to choose flowers whose volatile blend indicates good forage (Menzel, 1985). Indeed, honeybees are able to differentiate between very subtle differences in odor blends, as for instance between two genotypes of the same species or between flowering stages (Pham-Delègue et al., 1989; Wright et al., 2002). On the other hand, many of the variations in volatile emissions displayed by flowers are not indicative of any difference in reward quality, and therefore, another key ability is olfactory generalization. This ability corresponds to extending a behavior learned for a given stimulus to other, novel, stimuli, which are perceived as different, but sufficiently similar, to the learned one (Shepard, 1987). As for many lines of work about honeybee behavior and sensory capacities, both of these abilities were first recognized experimentally by Karl von Frisch. In a pioneering investigation, von Frisch (1919) trained free-flying bees to visit an artificial feeder presenting several essential oils (odor mixtures). Using a set of 32 such odors, von Frisch observed that after learning that one odor was associated with sucrose solution, bees tended to prefer this odor over others, clearly discriminating among odors, although they also sometimes visited other odors that were, to the human nose, similar to the rewarded one, thus displaying clear generalization behavior. This work laid the ground to a plethora of experimental studies on the olfactory detection, perception, and learning capacity of honeybees with odors.
Olfactory Learning Protocols in Freely Flying and Restrained Bees
Many experiments have been performed with free-flying bees visiting scented feeders (e.g., Kriston, 1971, 1973; Pham-Delègue et al., 1993; Laska et al., 1999). These experiments have the advantage of providing an ecologically relevant context, but many variables of the experiment, like bees’ physiological status, or the time intervals between learning trials, cannot be precisely controlled. Moreover, the search for the neural basis of olfaction needs the use of neurophysiological methods to monitor the bee brain while it processes and learns odors (Menzel, 1999; Giurfa, 2007). For these reasons, an experimental protocol allowing the study of olfactory learning on restrained individuals was developed, the conditioning of the proboscis extension reflex (PER; Figure 1A). The PER was initially described by Minnich (1932) in flies and Frings (1944) in bees. When the antennae, mouthparts, or tarsi of a hungry bee are touched with sucrose solution, the animal reflexively extends its proboscis to suck the sucrose. This response was later conditioned by Kuwabara (1957) and Takeda (1961), by associating visual and olfactory stimuli respectively with a sucrose reward. Perfecting the olfactory version of this protocol, Bitterman et al. (1983) also showed that it corresponds to a case of associative Pavlovian conditioning. Odors to the antennae do not usually release a PER in naive animals. If an odor is presented immediately before the sucrose solution (forward pairing), an association is formed and the odor will subsequently trigger the PER in a following test (Figures 1A,B). Thus, the odor can be viewed as the conditioned stimulus (CS) and sucrose solution as the reinforcing unconditioned stimulus (US). This association is thought to recapitulate the final phase of the foraging behavior, when bees drink nectar from an odorous flower.
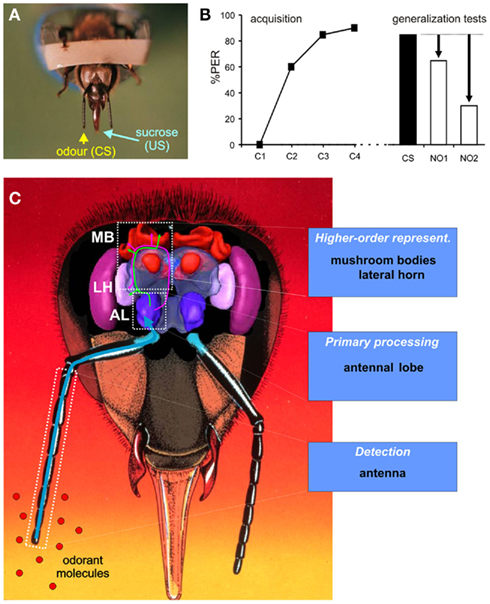
Figure 1. The study of olfactory learning and generalization in bees. (A) Conditioning of the proboscis extension reflex (PER) on restrained bees. The PER is a reflex shown by bees when their antennae, tarsi, or mouthparts are contacted with sucrose solution. During conditioning, an odor (conditioned stimulus, CS) is presented in temporal association with sucrose solution to the antennae and to the proboscis (unconditioned stimulus, US), so that the odor progressively gains control over the PER [see acquisition in (B)]. After conditioning, presentation of the odor alone triggers the PER. (B) Generalization experiment. During acquisition, bees learn to associate the CS with the sucrose reinforcement and respond with a PER to the CS. In a test phase, bees are presented in a random order with the CS and novel odorants 1 and 2 (respectively NO1 and NO2). The perceptual similarity between the CS and each novel odor can be measured as the response level to this novel odor relative to responses to the CS. In this example, NO1 would be considered as more perceptually similar to the CS for bees, than NO2. (C) Frontal view of the bee head showing a three-dimensional model (from Brandt et al., 2005) of the brain. Olfactory processing follows three main steps. First, odors are detected at the level of the antenna. Information is conveyed to the antennal lobe (AL) for primary processing. Processed information is then relayed by different pathways to higher-order centers, the mushroom bodies (MB), and the lateral horn (LH), creating multiple olfactory representations in the bee brain (see Figure 4C).
More recently, another type of Pavlovian conditioning protocol on restrained individuals was developed, which is based on aversive associations. The sting extension reflex (SER) is a defensive response of bees to potentially noxious stimuli (Breed et al., 2004), which can be elicited experimentally by delivering a mild electric shock to the thorax (Núñez et al., 1983, 1998; Balderrama et al., 2002). During conditioning, harnessed bees learn to associate an initially neutral odor (CS) with the electric shock (US; Vergoz et al., 2007; Giurfa et al., 2009; Roussel et al., 2009). While PER conditioning is appetitive and induces attraction toward the CS in a choice test (Sandoz et al., 2000; Chaffiol et al., 2005; Carcaud et al., 2009), SER conditioning is aversive and bees will accordingly avoid the CS (Carcaud et al., 2009). Hence, olfactory processing, detection and learning capacities of honeybees can now be studied and compared with respect to different reinforcement modalities.
Learning of Odors with Different Biological Meanings
The olfactory abilities and behavior of honeybees are the fruit of millions of years of co-evolution between hymenoptera and angiosperms. One could imagine that it would be beneficial to bees to not only be able to learn the features of rewarding flowers, but also to “know” in advance the sensory characteristics of a potential food source (Menzel, 1985). Do bees have an “innate search image”? It was initially expected that bees would only be able to learn floral odorants in an appetitive context, but the extreme plasticity of their olfactory learning behavior was soon recognized (von Frisch, 1919). While certain odorants are clearly attractive to bees prior to foraging (essential oils, aggregation pheromones), others are also clearly repulsive (propanol, 3-methyl indole). However, bees can still learn to associate these stimuli with sucrose reward, both in free-flying (Kriston, 1971, 1973) and in restrained conditions (Vareschi, 1971). Nevertheless, some odorants will be learned more quickly than others (above studies), and after learning, may produce stronger or longer responses (Smith and Menzel, 1989). However, it is difficult to interpret such differences as truly “innate,” since bees already learn odors within the hive (Farina et al., 2005) and may not be truly naïve when used in conditioning experiments.
Interestingly, bees can even learn to associate pheromonal odors with sucrose reinforcement. This has been proven with aggregation pheromones (citral, geraniol, Getz and Smith, 1987; Smith, 1991; Laska et al., 1999), and even more surprisingly with alarm pheromones (IPA and 2-heptanone, Smith and Menzel, 1989; Laska et al., 1999; Sandoz et al., 2001). However, even though learning does take place, social pheromones do not seem to be treated like general odors (Getz and Smith, 1991; Sandoz et al., 2001). For instance alarm pheromones (IPA and 2-heptanone) produce very high generalization to other odors (Sandoz et al., 2001). Most puzzling, although these two molecules do not have a similar structure, very high generalization was observed between them, suggesting that bees may have also associated their biological value (here alarm) with the appetitive reward and used this information to generalize.
Odor Discrimination and Generalization
As explained above, stimulus discrimination and generalization are two crucial abilities for bees. To study discrimination, researchers use differential conditioning procedures: bees are repeatedly presented with two odors, one (CS+) that is associated with reinforcement, while the other (CS−) is presented without reinforcement. If bees respond significantly more to the CS+ than to the CS−, it can then be concluded that they can discriminate between them. To study generalization, bees are simply conditioned to one odorant (CS) and are then presented with novel odorants without reinforcement (Figure 1B). The perceived similarity between the CS and each novel odorant is measured as the level of response to this odorant relative to the CS (amount of generalization, Figure 1B).
An important question in sensory neuroscience is along which dimensions animals measure similarity among stimuli (Shepard, 1987). Vareschi (1971) was the first to use PER conditioning to study the discrimination capacities of honeybees with a wide range of odors. He used a kind of differential conditioning, with one rewarded odor (CS) and 27 non-rewarded odors presented in-between CS trials. Bees were found to differentiate the odors from >95% of the 1816 tested odor pairs. The same high discrimination ability is also found in free-flying bees (97% of 1848 tested odor pairs, Laska et al., 1999).
In the bee, as in vertebrates (Mori et al., 2006; Johnson and Leon, 2007), aliphatic odor molecules have attracted the interest of researchers because they can be described by two main characteristics: their chemical group and the length of their carbon chain. Bees generalize more often between odors with similar carbon chain lengths or belonging to the same functional group, as found with restrained (Smith and Menzel, 1989) and with free-flying bees (Getz and Smith, 1990; Laska et al., 1999). Recently, Guerrieri et al. (2005b) systematically studied the generalization behavior of bees with 16 odorants presenting all combinations of four possible functional groups (primary and secondary alcohols, aldehydes, ketones) and chain lengths (six to nine carbons). These authors found that generalization is not always symmetrical, so that generalization from odor A to odor B is not always the same as from B to A. Strikingly, learning an aldehyde induced low generalization to other odors, while bees often responded to aldehydes after learning other odorants. In this study, the first factor determining honeybees’ generalization behavior was a molecule’s chain length, followed by the chemical group. This was the demonstration on a simple set of odor molecules that chemical dimensions are somehow encoded in the brain of honeybees and determine their behavior (Guerrieri et al., 2005b). However the bees’ natural environment provides an incredible wealth of possible odor molecules and we are still far from knowing the encoding dimensions for all these molecules.
Odor Concentration
The fact that honeybees are able to learn absolute odor concentrations was recognized by Kramer (1976), who trained individual workers in simulated odor gradients using a locomotion compensator with feedback control of odor concentration. The bees were reinforced with sucrose solution at a particular concentration of an odor, and were then placed at different concentrations. They showed a typical upwind walk when placed in a range of concentrations relatively close to the learned one (20–180%), but walked downwind when placed outside of these boundaries. Moreover, bees showed a particular alerting behavior at about 85–90% of the learned concentration (Kramer, 1976). Similarly, free-flying bees visiting a vertical odor array choose the right odor at the right concentration and reject higher or lower concentrations (Ditzen et al., 2003). In contrast to the freely moving situation, differential conditioning with two concentrations of the same odor is difficult in harnessed bees (Bhagavan and Smith, 1997; Pelz et al., 1997). Honeybees’ sensory capacity and motivation may be different in these two situations. In the visual modality, for instance, honeybees easily associate colors or patterns with sucrose reward when flying freely, but show much lower performance when restrained (especially when the antennae are not cut, Hori et al., 2006; Mota et al., 2011).
Concentration strongly influences the salience of olfactory stimuli. Generally, odors are learned more quickly at higher concentration (Bhagavan and Smith, 1997; Wright et al., 2009), and support better memory consolidation (Pelz et al., 1997). Moreover, conditioned responses to a high concentration are produced more quickly, suggesting that the olfactory system needs less time to determine odor quality at high than at low concentration (Wright et al., 2009). The discrimination power between different odorants also increases with their concentration (Getz and Smith, 1991; Wright and Smith, 2004). Lastly, bees generalize more from low to high concentrations, than from high to low concentrations (Marfaing et al., 1989; Getz and Smith, 1991; Bhagavan and Smith, 1997; Pelz et al., 1997). However, in some instances bees generalize more between different odors at the same concentration, than between different concentrations of the same odor (Wright et al., 2005). To summarize, odor identity is not totally invariant as a function of concentration, so that it is both possible for bees to differentiate between concentrations of an odorant, but also to show high generalization between different concentrations of this odorant. Such versatile capacities may be crucial when foraging for identifying and locating floral sources.
The Case of Olfactory Mixtures
Natural floral odors encountered by foraging bees are not single molecules but complex mixtures (Knudsen et al., 1993). Honeybees are thus confronted to the problem of discriminating among complex blends but also of recognizing the same floral source although its blend composition varies. Some authors have attempted to understand complex mixture processing in learning experiments with whole floral extracts (Pham-Delègue et al., 1986; Le Métayer et al., 1997) or with synthetic mixtures of six to 14 components (Pham-Delègue et al., 1993; Wadhams et al., 1994; Blight et al., 1997; Reinhard et al., 2010). A general finding of these experiments is that when bees learn a mixture and are afterward tested with the individual components, they usually respond to some components more strongly than to others. Such components have been termed key-compounds (or key-components, Wadhams et al., 1994; Laloi et al., 2000; Reinhard et al., 2010). What determines that a component is a key-component? Neither relative quantity nor volatility are predictive (Wadhams et al., 1994; Le Métayer et al., 1997; Reinhard et al., 2010). Rather, the perceptual salience of a component appears to be important, as measured by the conditioning success with this odor presented alone (Laloi et al., 2000). Additionally, whether a component will be learned in a mixture depends on the identity of the other components (Laloi et al., 2000; Reinhard et al., 2010). Thus, the processing of different odorants simultaneously produces unpredictable outcomes, a phenomenon termed “mixture interaction.” Due to the apparent complexity of mixture processing, research on mixture interactions has focused on binary mixtures (Getz and Smith, 1987, 1990, 1991; Chandra and Smith, 1998; Smith, 1998; Deisig et al., 2001). Generally an odor is better learned when presented alone, than when together with a second odorant (Smith, 1998). Usually, when learning a mixture AB, bees can recognize the components (Getz and Smith, 1987, 1990). However, one component is often learned better than the other, a phenomenon called “overshadowing.” Using three odors presented in the form of binary mixtures, Smith (1998) found that overshadowing depended on which odors were in a pair, so that overshadowing was difficult to predict. Mixture interactions may also depend on the sequence of experiences the bee has had with the stimuli. In the phenomenon of “blocking,” initial learning of an odorant A blocks learning of odorant B when the mixture AB is subsequently trained. Although this effect has been observed in different studies (Smith and Cobey, 1994; Linster and Smith, 1997; Hosler and Smith, 2000), it remains controversial, as it only rarely appeared when possible confounding variables were controlled (Gerber and Ullrich, 1999; Guerrieri et al., 2005a).
On a theoretical level, concepts from psychophysical theories have been used to attempt to understand how a mixture is represented in the bee brain (Chandra and Smith, 1998; Deisig et al., 2001; Lachnit et al., 2004). Two widely differing theories have been put to the test. First, the elemental approach assumes that a compound AB will be represented in the brain as two elements, A and B, each of which can be associated with the US (Rescorla and Wagner, 1972). In other terms, “the whole equals the sum of its parts.” On the other hand, configural approaches propose a radically different view by assuming that the representation of AB is a different entity from those of A and B (“the whole is different from the sum of its parts,” Pearce, 1987, 1994). In fact both accounts were shown to be wrong using the so-called patterning experiments (Chandra and Smith, 1998; Deisig et al., 2001). In such experiments, bees have to differentiate between two single odorants A and B and the mixture AB. In negative patterning, the single elements are both reinforced when presented alone (A+, B+), while the mixture is non-reinforced (AB−). Conversely, in positive patterning, the two elements are non-reinforced when presented alone (A−, B−), while the mixture is reinforced (AB+). Honeybees can be trained to solve both tasks with odors (Deisig et al., 2001, 2002). The elemental approach can explain positive patterning but not negative patterning, because when each component is reinforced, a mixture would elicit, through elemental summation, twice as much responding as each component. On the other hand, in its principle, the configural approach could cope with both patterning tasks, as compound and elements are associated with reinforcement independently (Pearce, 1994). However, it ran into problems when analyzing response summation between elements and mixtures at the beginning of conditioning (Deisig et al., 2003). Experiments in bees thus suggested that the best model for explaining mixture learning was an expansion of elemental models, called the unique cue hypothesis (Rescorla, 1972, 1973; Whitlow and Wagner, 1972). In addition to the representations of the elements, the compound would give rise to a supplementary (internal) representation, the unique cue. During the negative patterning problem, the unique cue U would build inhibitory associations with the US, while the elements A and B would build excitatory associations. When A and B are presented alone, the excitatory association would thus trigger behavioral responses, but during AB presentations, the added inhibitory strength of the unique cue would hamper the response. Using different types of patterning tasks, it was possible to show that a version of the unique cue hypothesis best coped with all the experimental results (Deisig et al., 2001, 2002, 2003; Lachnit et al., 2004).
For simplicity, all experiments described above considered that mixture composition is stable in time, which is not the case in nature, as floral aroma changes throughout the day and the plant’s state. Honeybees seem to focus on those components which remain relatively constant in their concentration (Wright and Smith, 2004). Such ability may be beneficial for bees in order to recognize the same floral species in spite of fluctuations in the composition of its odor blend.
Neuroanatomy of the Honeybee Olfactory System
An advantage of the bee model for understanding olfaction and olfactory learning is that the neuroanatomy of its olfactory pathway is known in great detail (e.g., Kenyon, 1896; Pareto, 1972; Suzuki, 1975; Mobbs, 1982; Abel et al., 2001; Strausfeld, 2002; Kirschner et al., 2006). Olfactory processing follows different steps, from the detection of molecules at the periphery, via primary processing by antennal lobe (AL) networks, until the establishment of olfactory representations in higher-order brain centers (Figure 1C). A simplified model of the different neuron types involved in olfactory processing is provided in Figure 2.
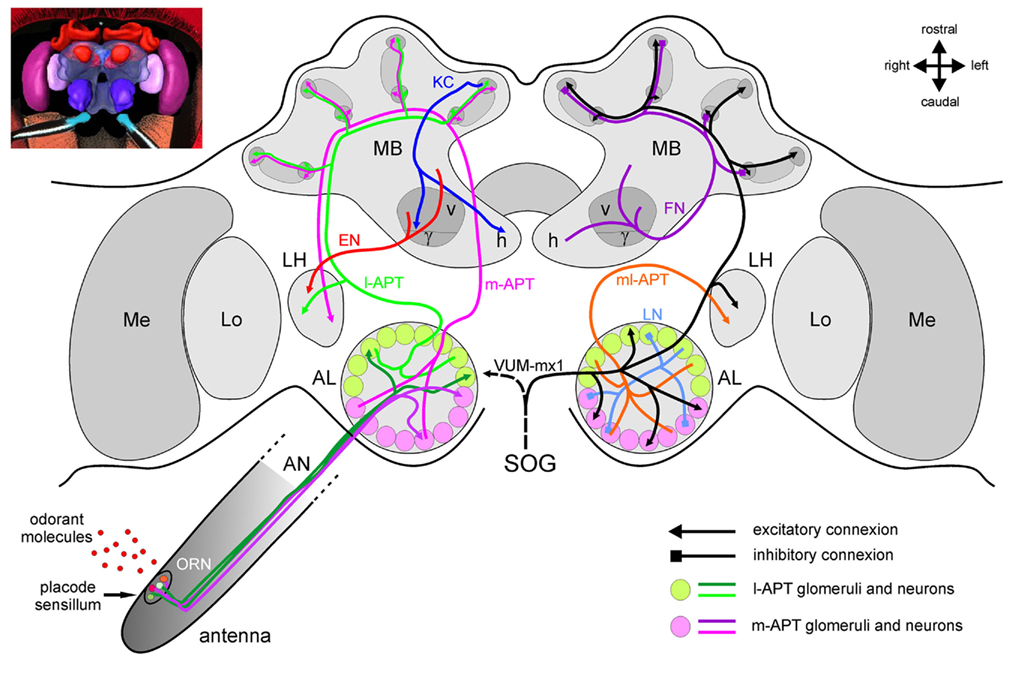
Figure 2. The honeybee brain and the olfactory pathway. For clarity, different neuron types have been presented separately in the two brain hemispheres. On the left, major excitatory pathways involved in the transmission of olfactory information in the brain are shown. On the right, mostly inhibitory connections and modulatory neurons are presented. The antennal lobe, first-order olfactory neuropil, receives input from ~60,000 olfactory receptor neurons (ORNs) which detect odorants within placode sensilla on the antenna. Within the AL’s anatomical and functional units, the 165 glomeruli, ORNs contact ~4000 inhibitory local neurons (LNs) which carry out local computations, and ~800 projection neurons which further convey processed information via different tracts. The lateral antenno-cerebralis tract (l-APT) projects first to the lateral horn (LH) and then to the mushroom body (MB) calyces (lips and basal ring), while the medial tract (m-APT) projects to the same structures, but in the reverse order. Both tracts are uniglomerular, each neuron taking information within a single glomerulus. They form two parallel, mostly independent olfactory subsystems (in green and in magenta), from the periphery until higher-order centers, where they project in non-overlapping regions. Multiglomerular projection neurons form a medio-lateral tract (ml-APT) which conveys information directly to the medial protocerebrum and to the LH. The dendrites of the Kenyon cells (KCs), the mushroom bodies’ 170,000 intrinsic neurons, form the calyces, while their axons form the pedunculus. The output regions of the MB are the vertical and horizontal lobes, formed by two collaterals of each KC axon. Within the MBs, feedback neurons (FN) project from the pedunculus and lobes back to the calyces, providing inhibitory feedback to the MB input regions. Extrinsic neurons (ENs) take information from the pedunculus and the lobes and project to different parts of the protocerebrum and most conspicuously to the LH. It is thought that descending neurons from these areas are then involved in the control of olfactory behavior. The figure also presents a single identified octopaminergic neuron, VUM-mx1, which was shown to represent reinforcement during appetitive conditioning. This neuron projects from the suboesophageal ganglion (SOG), where it gets gustatory input from sucrose receptors, to the brain and converges with the olfactory pathway in three areas, the AL, the MB calyces, and the LH.
Peripheral Odor Detection: The Antenna
Peripheral odor detection starts at the level of olfactory receptor neurons (ORNs), which are located below cuticular structures on the antennae, called sensilla (Kaissling, 1987). Different morphological types of sensilla exist on the insect antenna, but sensilla placodea (pore plate sensilla) are the main olfactory sensilla in the honeybee (Esslen and Kaissling, 1976). A sensillum placodeum is formed by an oval-shaped (9 μm × 6 μm) thin cuticular plate with numerous minute pores and is innervated by five to 35 ORNs (Schneider and Steinbrecht, 1968; Esslen and Kaissling, 1976; Kelber et al., 2006). Odorant molecules reach the dendrites of ORNs by diffusing through an extracellular fluid, called the receptor or sensillum lymph, filling the sensillum cavity (Kaissling, 1987; Masson and Mustaparta, 1990). In this fluid, odorant binding proteins (OBPs) may help transporting odorants to the ORNs but very little is known about them in bees.
When reaching the ORN membrane, the odorant molecule interacts with the olfactory receptor protein (OR). Insect ORs belong to a family of highly divergent proteins with seven-transmembrane domains, which are different from the vertebrate OR family (Benton, 2006; Touhara and Vosshall, 2009). The functional receptor is a heteromeric complex of an OR and a broadly expressed co-receptor AmOr2, which is the honeybee ortholog to the co-receptor Or83b of Drosophila (Benton et al., 2006; Robertson and Wanner, 2006). Honeybees present a remarkable expansion of the insect odorant receptor family relative to the repertoires of the fly Drosophila melanogaster and the mosquito Anopheles gambiae, which respectively possess 62 and 79 ORs, with a total of 170 OR genes including seven pseudogenes (Robertson and Wanner, 2006).
The Primary Olfactory Center: The Antennal Lobe
ORN axons form the antennal nerve and project to a primary olfactory center in the brain, the AL (Figure 2). The bee AL is compartmentalized in 165 spheroidal neuropile units called glomeruli. Glomeruli are the anatomical and functional units of the AL and constitute the first site of synaptic interaction between ORNs and other neuron types. Glomeruli can be recognized based on their relative position, size, and shape, using an anatomical atlas of the AL (Flanagan and Mercer, 1989a; Galizia et al., 1999a). In Drosophila axons of ORNs expressing the same odorant receptor converge onto the same glomerulus (Vosshall et al., 2000; Dahanukar et al., 2005). Thus, the array of AL glomeruli corresponds to an array of OR types. Noticeably, the number of 163 potentially functional ORs in bees coincides with the number of glomeruli in the AL (~165). This would thus support the one-receptor/one-ORN/one-glomerulus hypothesis in bees.
Within each glomerulus, ORNs release acetylcholine (ACh), the primary excitatory transmitter of the insect brain (Bicker, 1999). Thus doing, they activate local neurons (LNs) connecting different glomeruli and projection neurons (PNs), which relay the olfactory message processed at the level of the AL to higher-order centers such as the lateral horn (LH) and the mushroom bodies (MBs).
Local neurons are neurons whose branching patterns are restricted to the AL (Figure 2). The ~4000 LNs can be classified in two main types. One type innervates most if not all glomeruli in a uniform manner, and are therefore called homogeneous LNs (homo-LNs; Flanagan and Mercer, 1989b; Fonta et al., 1993). Neurons of the second type innervate only a small subset of glomeruli and are called heterogeneous LNs (hetero-LNs). They have one dominant glomerulus with very dense innervation and a few other glomeruli with very sparse processes (Flanagan and Mercer, 1989b; Fonta et al., 1993). Hetero-LNs branch in the core of the sparsely innervated glomeruli but branch in the whole (core and cortex) of their densely innervated glomerulus (Fonta et al., 1993; Abel et al., 2001). LNs are thought to carry out the first processing of olfactory information, with two different functions of Homo-LNs and Hetero-LNs, respectively global inhibition for gain control and asymmetrical lateral inhibition between glomeruli for refining odor representation and allowing better discrimination among olfactory representations (Sachse and Galizia, 2002).
Local neurons in bees are mostly inhibitory and may use many different neurotransmitters. About 750 LNs are GABAergic (Schäfer and Bicker, 1986) and functional data indicate that GABA is indeed inhibitory in the AL (Stopfer et al., 1997; Sachse and Galizia, 2002; Dupuis et al., 2010). In addition, glutamate (for review see Bicker, 1999) and histamine (only about 35 neurons, Bornhauser and Meyer, 1997) have been identified in the AL. Several lines of evidence indicate that glutamate (Barbara et al., 2005; El Hassani et al., 2008) and histamine (Sachse et al., 2006) also act as inhibitory neurotransmitters in the bee brain. Lastly, the AL houses many, often small, subpopulations of LNs which each express characteristic peptides, including allatostatins, allatotropin, tachykinins, FMRFamide, and other RFamide peptides (Galizia, 2008; Kreissl et al., 2010).
Projection neurons connect the AL with higher-order brain areas (Figure 2), following five different pathways, called antenno-protocerebral tracts (APTs; Mobbs, 1982; Abel et al., 2001). From their morphology, PNs can also be classified in two types. Uniglomerular projection neurons (uPNs) branch in a single glomerulus within the AL and have axons that project to the MBs and to the LH using the two major APT tracts (see below). On the other hand, multiglomerular projection neurons (mPNs) branch in most glomeruli and are therefore potentially capable, in contrast to uPNs, to extract combinatorial information across glomeruli. Their axons follow the three lesser tracts, the medio-lateral (ml) APTs, leading not to the MBs, but to other areas of the protocerebrum, surrounding the α-lobe of the MB or extending toward the LH (Abel et al., 2001; Kirschner et al., 2006).
The more numerous uniglomerular PNs (~800) form two roughly equal tracts toward higher-order brain centers, the lateral (l-APT), and medial (m-APT) tract. The l-APT leaves the AL dorsally and then runs on the lateral side of the protocerebrum, forming collaterals in the LH and then continuing on to the MB calyces. The m-APT runs along the brain midline first toward the MBs where collaterals enter into the calyces, and then travels laterally to end in the LH (Abel et al., 2001; Kirschner et al., 2006). The current understanding of this anatomical organization is that the honeybee brain may arbor two parallel olfactory subsystems, as excitatory transmission of the olfactory message follows essentially two independent pathways in each brain hemisphere toward higher-order brain centers. L- and m-APT neurons take their information from two non-overlapping groups of 84 and 77 glomeruli respectively (Abel et al., 2001; Kirschner et al., 2006). Following the current hypothesis that each glomerulus is the projection center for all ORNs expressing a given OR, one may thus say that PNs from the l- and m-APT each transmit information about two independent portions of the honeybee odor detection repertoire. Moreover, the central projection areas of the two PN tracts are segregated in the MB calyces and in the LH with only partial overlap (Abel et al., 2001; Kirschner et al., 2006). How are the two subsystems connected through local networks? Several hypotheses are possible, from almost total segregation of the subsystems with hetero-LNs providing lateral inhibition only within each system, to an equal and symmetrical weight of lateral inhibition between both subsystems (Galizia and Rössler, 2010). However, no data are available yet concerning this question.
Second-Order Olfactory Centers: The Mushroom Bodies and the Lateral Horn
Olfactory information leaving the AL takes several routes to the MBs and LH (Figure 2). While the function of the LH is still unclear, the MBs are thought to be involved in a sparsening of olfactory representation, in olfactory learning and memory, as well as the integration of olfactory information with other sensory modalities (Menzel et al., 1994; Menzel, 1999; Giurfa, 2003, 2007).
MB-intrinsic neurons are the Kenyon cells (KCs; Kenyon, 1896), which form two cup-shaped regions called calyces in each hemisphere. MB calyces are anatomically and functionally subdivided into the lip, the collar, and the basal ring (Mobbs, 1982, 1984; Gronenberg, 2001; Strausfeld, 2002). The lip region and the inner half of the basal ring receive olfactory input, whereas the collar and outer half of the basal ring receive visual input (Gronenberg, 2001), in addition to input from mechanosensory and gustatory pathways (Strausfeld, 2002; Schröter and Menzel, 2003). The projections of individual PNs extend in most parts of each calyx (Müller et al., 2002). PN boutons form multisynaptic microcircuits in the MB lips, with GABAergic input and KC output connections arranged to form particular structures termed microglomeruli (Ganeshina and Menzel, 2001). KC axons project in bundles into the midbrain, forming the peduncle and the vertical and horizontal lobes (also called α and β lobes). The calyx is topologically represented in the lobes (Mobbs, 1982; Strausfeld et al., 2000; Strausfeld, 2002). About 55 GABAergic feedback neurons from the MB output lobes project back to the calyces (Bicker et al., 1985; Figure 2). Due to the parallel arrangement of intrinsic KCs, most subcompartments in the calyx receive feedback from their corresponding layer in the α lobe (Grünewald, 1999). Most KCs provide bifurcating axons to both α and β lobes. In the bee, about 800 PNs diverge onto a major proportion of the 170,000 KCs of each MB (i.e., onto olfactory KCs). Each PN contacts many KCs and each KC receives input from many PNs. If the figures calculated for the locust Schistocerca americana (Jortner et al., 2007) were to apply to the honeybee, each KC would contact about 400 PNs (i.e., 50% of the total PN count). This organization appears ideal for a combinatorial readout across PNs (Laurent, 2002).
The second major target area of both the m- and l-APT uPNs is the LH. In addition to the uPN innervation, the LH receives input from mPNs via the ml-APTs (Fonta et al., 1993). Similarly to the olfactory input of the MB calyx, the LH shows a PN tract-specific compartmentalization, with at least four subcompartments: one receives exclusively projections of m-APT uPNs, while others receive mixed input from m- and l-APT PNs, from l-APT and ml-APT PNs, or from the latter type alone (Kirschner et al., 2006). Possible local computations within this structure as well as the connectivity between PNs and other neurons are still mostly unknown.
Mushroom Body Output
A number of neuron populations project from the MBs toward other brain centers (Figure 2), with two major output regions being the α and β lobes (Mobbs, 1982). About 400 extrinsic neurons (ENs) from the α lobe have been studied in details (Rybak and Menzel, 1993). Some are unilateral neurons with projection fields restricted to the ipsilateral protocerebrum, while others are bilateral neurons connecting both α lobes, or projecting from one lobe to the contralateral protocerebrum around the α lobe (Rybak and Menzel, 1993). A single conspicuous neuron in each MB, called Pe-1, forms a major output pathway from the peduncle of the MBs (Mauelshagen, 1993). This neuron arborizes extensively in the peduncle and projects to the lateral protocerebral lobe, and more specifically to the LH where it synapses directly or via interneurons onto descending neurons involved in behavior.
Although the anatomical description of projections to the LH is good, knowledge of the neurons leaving the LH and of descending pathways involved in behavioral output is still scarce in bees. Some anatomical descriptions of descending neurons in other insects, like cockroaches, suggest that their dendrites are distributed mainly in the lateral and medial protocerebrum, which are major termination areas of MB output neurons, but not in the AL, MBs, or regions of the LH receiving PN input (Okada et al., 2003). Thus it is possible that both MB output neurons and yet unknown LH output neurons contact descending neurons and can therefore modulate behavior. Investigation of descending neurons and the neural pathways involved in behavioral control in bees may help bridge this gap (Ibbotson and Goodman, 1990; Ibbotson, 2001; Schröter et al., 2007).
Aversive and Appetitive Reinforcement Information
The olfactory pathway also receives input from different modulatory systems. Of special importance are the reinforcement systems necessary for the formation of neural associations between odors and particular outcomes. Such associations rely on the co-activation of two neural pathways, the olfactory pathway and a pathway representing the specific reinforcement. As in other insects, appetitive reinforcement in bees depends on octopamine (Hammer and Menzel, 1998; Farooqui et al., 2003) and aversive reinforcement on dopamine (Vergoz et al., 2007). A single, putatively octopaminergic, neuron in the bee brain, VUM-mx1 (Figure 2), was shown to represent a neural substrate of the sucrose US pathway (Hammer, 1993), because the forward (but not backward) pairing of an odor CS with an artificial depolarization of VUM-mx1 produces an associative memory trace. VUM-mx1, has its cell body in the suboesophageal ganglion (SOG), and converges with the olfactory pathway in both brain hemispheres at three sites, in the AL, in the MB calyces, and in the LH. Another neuron with a similar projection pattern has been found with its cell body in another neuromere of the SOG (VUM-md1, Schröter et al., 2007). On the other hand, many dopaminergic neurons are found in the bee brain (Schäfer and Rehder, 1989; Schürmann et al., 1989) but until now, none of them could be shown to provide aversive reinforcement.
Neurophysiological Study of Olfactory Processing and Learning
Peripheral Odor Detection: The Antenna
The search for the neural correlates of olfactory detection and processing has started at the periphery, using extracellular recordings of single placodes (e.g., Lacher and Schneider, 1963; Lacher, 1964; Vareschi, 1971; Akers and Getz, 1992, 1993). The first demonstration that placode sensilla were responsible for olfactory detection was provided by Lacher and Schneider (1963) with individual placode recordings showing responses to benzylacetate in workers, and caproic acid in drones, but no answers to light, sound, water vapor, or CO2. ORNs show little spontaneous activity, but respond to odors mostly with a spike frequency increase in a phasic-tonic manner. Sometimes, they also show inhibitory responses or off-responses (response at the end of the stimulus). Because a single placode houses many ORNs it is difficult to segregate the responses of individual cells based on spike amplitude. Using statistical techniques to attempt such segregation, Akers and Getz (1992) found that units with similar odor-response spectra were more likely to be found in different placodes than within the same placode. This observation fits with anatomical data showing that ORNs from a placode innervate different glomeruli (Kelber et al., 2006). The complex organization of ORNs in the honeybee antenna has strongly hindered efforts to study peripheral odor detection in this species.
Olfactory Processing in the Antennal Lobe
Thanks to the technique of in vivo calcium imaging, it was however possible to record neural activity at the glomerular level (Joerges et al., 1997, Figure 3). This recording technique uses fluorescent dyes to measure the increase of intracellular calcium (coming from the extracellular medium and/or released from internal stores) following neuronal excitation (Joerges et al., 1997; Galizia and Vetter, 2004). In the most simple form of this technique, bees are fixed in a recording chamber, and the head capsule is carefully opened (Figures 3A,B). Membranes and tracheas covering the brain are removed, and a calcium-sensitive fluorescent dye (for instance, Calcium Green 2-AM) is bath-applied onto the brain (Figures 3C,D). After about 1 h incubation, during which the dye has penetrated AL cells, the brain is rinsed with saline solution and the bee is placed under an upright fluorescence microscope in front of an odor stimulation device (Figure 3A). This bath-application of Calcium Green 2-AM allows recording a composite calcium signal which could potentially come from all cell populations of the AL: ORNs, LNs, PNs, and glial cells (Joerges et al., 1997). Due to the numerical preponderance of ORNs and because odor-induced signals have a very stereotypical time course and do not show spontaneous activity or inhibitory responses (the hallmark of LNs and PNs, see below), these recordings are thought to emphasize presynaptic calcium variations from ORNs (Galizia et al., 1998; Sachse and Galizia, 2003), with a possibly significant contribution from glial cells surrounding each glomerulus (Galizia and Vetter, 2004). The compound signal has therefore been long interpreted as representative of sensory input (Sachse and Galizia, 2003; Deisig et al., 2006, 2010; Sachse et al., 2006).
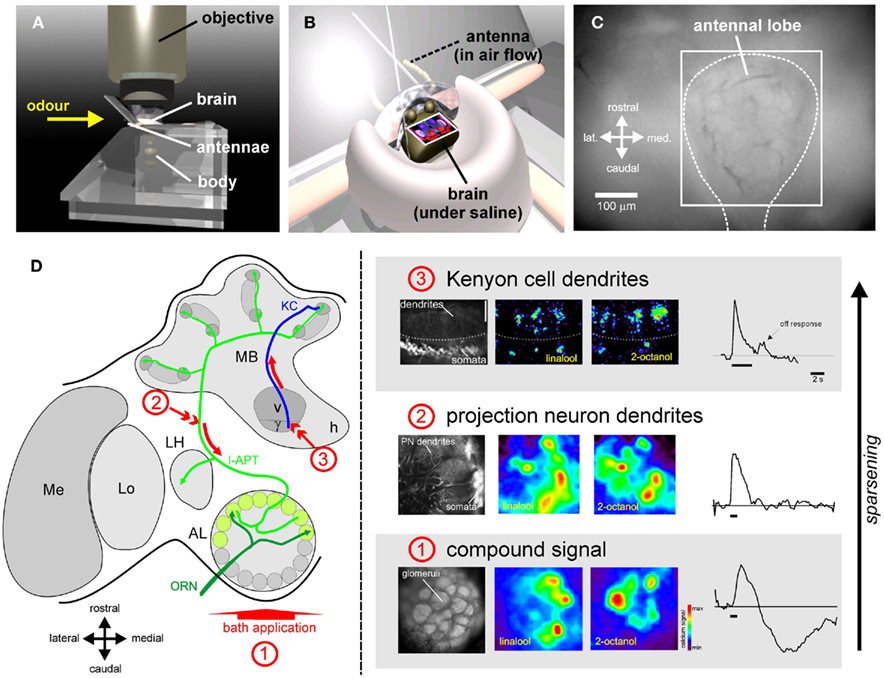
Figure 3. Optical imaging of odor representations in the bee brain. Thanks to in vivo calcium imaging, odor representation can be recorded in the bee brain. (A) Bees are placed under an epifluorescence microscope in front of an odor-delivery device delivering a permanent airflow. (B) Their head capsule is opened revealing the brain, which is then kept under saline solution at all times, while the antennae are maintained in the airflow. (C) Example view of the brain surface after bath-application of a calcium dye (method 1, below). The recording can be restrained to the region corresponding to the antennal lobe (square). (D) Using different staining techniques, odor representation was recorded at different levels of olfactory processing. On the left the staining technique and the imaged neuronal population are shown, while on the right, activity maps evoked by two sample odorants (1-hexanol and linalool) as well as an exemplary time course are presented. (1) Using bath-application of a calcium-sensitive dye (Calcium-Green 2-AM), a compound signal can be recorded in the antennal lobe in response to odors (Joerges et al., 1997). This signal is thought to represent mostly olfactory input from the ORN population (see text). Different odors induce different, but overlapping, multiglomerular activity patterns. Bath application signals are temporally slow and biphasic. (2) Using retrograde staining with a migrating dye (Fura-2 dextran), projection neurons can be selectively stained (Sachse and Galizia, 2002). A dye-coated electrode is inserted into the PNs axon tract (arrow number 2). The dye is taken up by the neurons and migrates back to their dendrites in AL glomeruli. Such staining allows the selective recording of AL output information sent to higher-order centers. Odors also induce multiglomerular activity patterns, but these are scarcer (less glomeruli are activated) and more contrasted than the compound signals. The time course is mostly phasic-tonic, but also presents some complex temporal patterns and inhibitions. (3) Inserting the dye-coated electrode into the ventral part of the vertical lobe allowed recording activity from Kenyon cell dendrites and somata (Szyszka et al., 2005). Olfactory representation becomes even sparser in the MBs as few KCs respond to each odorant. Responses are phasic and often present off-responses at stimulus offset. (Recordings 1 and 2 from Deisig et al., 2006, 2010 – Recordings 3 from Szyszka et al., 2005).
Optical imaging experiments showed that odors elicit combinatorial activity patterns across glomeruli (Joerges et al., 1997; see Figure 3D). Combining imaging recordings with anatomical staining allowed assigning activity patterns to identified glomeruli using the published anatomical AL atlas (Galizia et al., 1999a). This showed that odor quality is represented in the AL according to a specific distributed code conserved between individuals (Galizia et al., 1999b; Sachse et al., 1999). Each glomerulus – representing an ORN type expressing a given OR – shows a rather broad molecular receptive range (Galizia et al., 1999b). Because the optical imaging technique allows recording activity only at the tissue surface, only a small part of the 165 glomeruli could be accessed (up to 38 glomeruli; Sachse et al., 1999). The question therefore arose whether the signals recorded in this subpart of the AL had any significance with regards to odor representation and olfactory behavior. To answer this question, Guerrieri et al. (2005b) studied the generalization behavior of honeybees among a panel of 16 odorants for which the activity patterns in these glomeruli were known (Sachse et al., 1999). As mentioned above, these authors built a complete generalization matrix among the 16 odorants differing according to their functional group and their carbon chain length (Figure 4A). Importantly, this work demonstrated for the first time a significant correlation between the similarity among odors in the behavior and in the neurophysiological recordings (Figure 4B). Thus, calcium signals in this subpart of the AL could to some extent allow predicting bees’ generalization behavior. As shown on the figure, the data showed however some scatter and the question whether extending the neurophysiological recordings to more glomeruli, or to other parts of the brain may ameliorate this prediction remains unanswered. In theory, the bee brain contains different sets of olfactory representations in its different olfactory structures, which each can be characterized by an odor-similarity matrix based on combinatorial activity of its neuronal units (Figure 4C). It will be the goal of future research to compare the capacity of these different levels for predicting bees’ olfactory behavior.
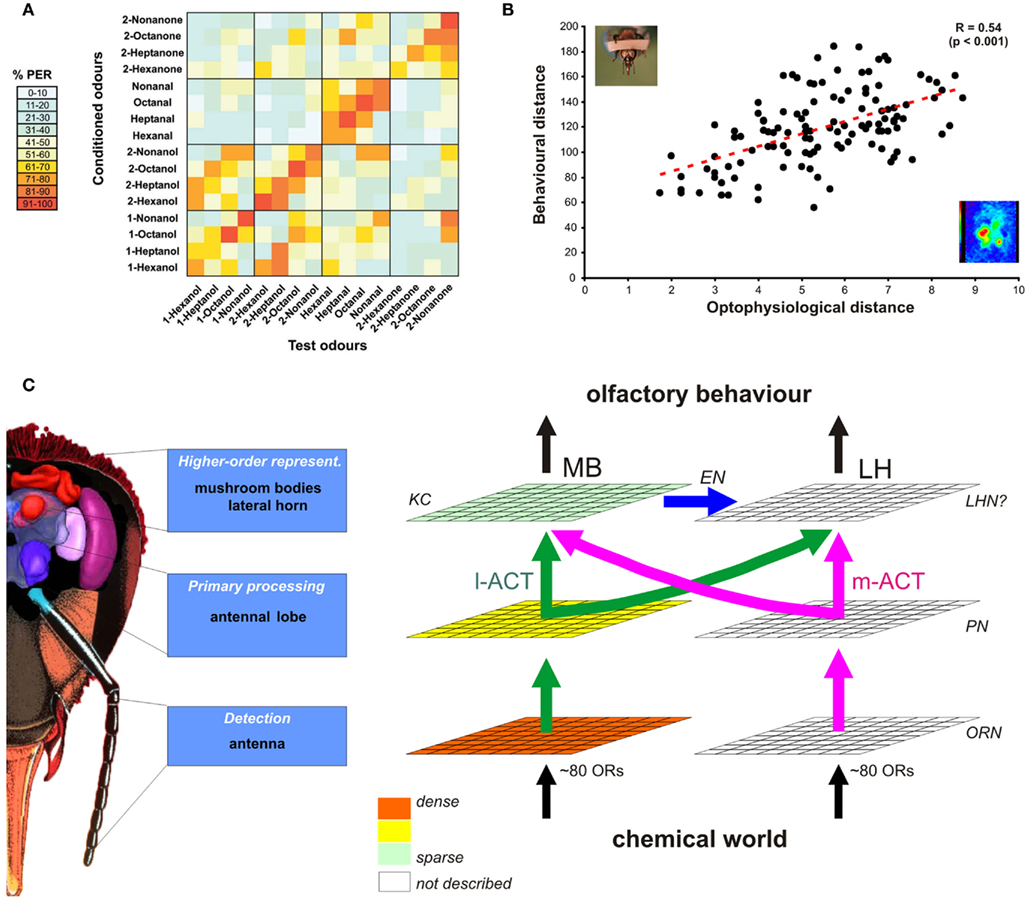
Figure 4. Neural representations of odors and olfactory behavior. (A) Using a generalization experiment as shown in Figure 1B, it was possible to measure the perceptual similarity among all possible pairs of 16 aliphatic odorants. The table presents the amount of generalization between any two of these odors. Odors used for conditioning are presented vertically, while odors used in the generalization tests are presented horizontally. Bees respond preferentially to the learned odor (main diagonal), but also to other – perceptually similar – odors. For instance, they generalize between odors sharing the same carbon chain length (smaller diagonals) or the same functional group (boxes along diagonals, see for instance the high generalization among aldehydes). (B) Similarity among odors at the neural level (measured in the AL using bath-application of the calcium dye, method 1 in Figure 3) significantly correlates with similarity at the behavioral level, as measured in (A). Thus, the AL contains a neural representation of odors which allows predicting to some extent the bees’ olfactory behavior. (C) At its different processing levels, the bee brain is thought to contain multiple odor representations, which can be characterized by different odor-similarity matrices. Sequential and/or parallel transformation of olfactory information shapes odor representations in higher-order centers that would eventually determine olfactory behavior. Thus, higher-order representations should correlate more strongly with behavioral output than more peripheral representations [like AL input, see (B)]. As shown in Figure 3, we have access to representations at the level of ORNs, PNs, and KCs corresponding to the l-APT subsystem. There, a sparsening of olfactory representation is found, but its influence on predicting behavior is still unknown. In addition, representations in the m-APT subsystem, as well as in the LH are still unknown and should be studied in future work.
AL neurons are involved in the processing of incoming odor information provided by ORNs. Intracellular recordings of LN and PN responses provided some insights about this processing (Flanagan and Mercer, 1989b; Sun et al., 1993; Stopfer et al., 1997; Abel et al., 2001; Müller et al., 2002; Krofczik et al., 2009). LNs are odor-specific, responding in a differential manner to different odors. They can show excitatory responses to some odors and inhibitory responses (i.e., a reduction of spiking activity relative to background) to others (Sun et al., 1993). Staining of hetero-LNs allows identifying the glomerulus in which this LN most intensively branches (Galizia and Kimmerle, 2004). Generally, the response profile of the recorded LN corresponded to the known response profile of the innervated glomerulus, suggesting that hetero-LNs take their input in this glomerulus. LNs tend to show a shorter latency than PNs, which allows them to rapidly and efficiently inhibit the firing of PNs (Krofczik et al., 2009).
Projection neuron responses are the product of direct excitation from ORNs, direct inhibition from LNs and possibly disinhibition from LN–LN connections and can therefore be temporally complex (Sun et al., 1993; Müller et al., 2002). PNs are usually spontaneously active and can change their responses upon odor presentations in an either excitatory or inhibitory manner (respectively increasing or decreasing firing rate; Abel et al., 2001; Müller et al., 2002). However, PNs belonging to the two anatomical tracts conveying information to MBs and LH may have different response properties. In contrast to initially thought (Müller et al., 2002), there does not seem to be very clear-cut differences between PN pathways in their propensity to respond to odorants, all PNs responding rather non-specifically to many odors (Krofczik et al., 2009). On average, the dynamic response profiles of l- and m-APT neurons were found to be similar so that in both systems odor identity would be encoded both in the pattern of response latencies and in the subset of activated PNs. However, even though responses to single odors may be similar, this work and a recent imaging study (Yamagata et al., 2009) both showed that the two subsystems may treat odor mixtures differently. Moreover, the two systems seem to respond differently to odor concentration (Yamagata et al., 2009).
While intracellular recordings precisely describe the temporal response patterns of individual AL neurons to odors, imaging methods allow recording the combinatorial responses of many PNs simultaneously. This was possible using back-tracing of PN processes with the calcium dye Fura-dextran (Figure 3D). By placing a high concentration of dye into the axonal tract of l-APT PNs on their way to the MBs, the dye is taken up by the neurons and transported retrogradely to the soma near the AL, and to the dendrites within AL glomeruli (Sachse and Galizia, 2002). In agreement with electrophysiological recordings, the imaging recordings showed that PN odor-response patterns are temporally more complex than the input activity (bath application), and can show both excitation and inhibition phases. These calcium responses seem to follow – although with a lower temporal resolution – the spiking activity of the neurons, as shown by consecutive intracellular electrophysiology and optical imaging of the same AL neurons (Galizia and Kimmerle, 2004). Imaging of PN population activity allowed comparing glomerular activity patterns between the input (Sachse et al., 1999) and PN output representation, even within the same animal (Sachse and Galizia, 2003). Such comparison showed that most glomeruli which are intermediately or weakly active in the compound signal, do not present any calcium increase in PNs (Sachse and Galizia, 2002). Thus, PN patterns are sparser than input patterns. Moreover, it was found that AL networks improve the separability of odor representations, both with single odors over a wide concentration range (Sachse and Galizia, 2003) and with mixtures (Deisig et al., 2010, see below).
From this, AL processing appears to perform mainly two operations: gain control, which quantitatively controls the overall amount of PN activity and contrast enhancement which qualitatively modifies the activity patterns. These two properties can be attributed to the action of inhibitory LN networks, in particular GABAergic ones. Indeed, application of GABA onto the brain blocks spontaneous activity and totally abolishes calcium response to odors (Sachse and Galizia, 2002). Conversely, application of a GABAA-like receptor antagonist, picrotoxin, stimulates spontaneous activity, and increases the number of activated glomeruli upon odor presentation, also modifying the time course of the signals. Picrotoxin also abolishes network oscillation dynamics (Stopfer et al., 1997). Imaging recordings confirmed the existence of (at least) two different inhibitory networks, following the anatomical features of the bee AL (Sachse and Galizia, 2002). The first one would be a global inhibitory network driven by all glomeruli and affecting all glomeruli, corresponding to homo-LNs. Based on the above results, it would be sensitive to picrotoxin and have a gain control function. The second network would be an asymmetrical inhibitory network driven by one glomerulus and affecting mainly another glomerulus, corresponding to hetero-LNs. The neurotransmitter for the hetero-LN network involved in contrast enhancement is still unknown. Glutamate (Barbara et al., 2005) or histamine (Sachse et al., 2006) may play such a role, but this has not been demonstrated to date.
Which rule underlies the inhibition relationships of hetero-LNs between individual glomeruli? Comparison of the result of computational modeling with imaging experiments established that the transformation of odor representation between AL input and output is best achieved by an interglomerular inhibition based on functional similarity between glomeruli and less so by inhibition based on anatomical neighborhood relationships or random connections (Linster et al., 2005).
Odor Representation in the Mushroom Bodies
After AL processing, l-APT and m-APT PNs convey information to the MB calyces. In honeybees, KCs, the MB-intrinsic neurons, are too small to perform intracellular recordings. Data from locusts suggest that whereas PNs respond to odors with trains of spikes, KCs often respond with a single or very few spikes (Perez-Orive et al., 2002). KCs do not show any spontaneous activity, and respond to very few odorants, i.e., representation at the KC level is highly sparse. Optical imaging recordings used Fura-dextran forward- and back-fills of PNs and KCs respectively, to study this transformation of odor representation from the AL to the MB (Szyszka et al., 2005, Figure 3D). This study showed that the proportion of cells responding to only one odor out of a four-odor panel increased at each level, respectively 55, 70, and lastly 92%. Thus, olfactory representation would follow a series of transformations with a progressive sparsening of odor representation (Figure 4C). The last step of sparsening, which takes place at the level of the MB calyx involves several mechanisms. First, the low synaptic strength between PNs and KCs would imply that coherent input from many PNs at the same time is needed to excite a KC (Perez-Orive et al., 2002). Second, KCs would detect coincidence among many PNs thanks to odor-driven inhibition produced by LH inhibitory neurons locked in anti-phase to PN oscillations (Perez-Orive et al., 2002, 2004). Third, local microcircuits involving GABA processes in the MB microglomeruli would also shape KC responses (Ganeshina and Menzel, 2001; Szyszka et al., 2005).
Odor representation at the KC level is thus highly sparse, and each KC represents a particular pattern of PN inputs, possibly for a particular concentration of an odorant (Stopfer et al., 2003) or a particular composition of a mixture (Broome et al., 2006). Therefore, they are thought to be the ideal representation of a particular odorant for storing associative memories, i.e., storing the information that one particular odor has been associated with a sucrose reward or with a noxious stimulus (Heisenberg, 2003; Gerber et al., 2004).
Mushroom Body Output and the Lateral Horn
The most studied MB output neuron is the Pe-1 neuron, which is recognizable by a characteristic firing pattern in doublets or triplets of action potentials (Mauelshagen, 1993; Rybak and Menzel, 1998; Okada et al., 2007). This wide-field neuron does not only respond to odors, but also to other sensory modalities (visual, mechanosensory). Moreover, it changes its responses during conditioning (Okada et al., 2007). At this level of the olfactory pathway, odor information is thus integrated with other modalities, and the function of neurons such as Pe-1 might not be to represent specific information about the learned odor like its identity, concentration, or multimodal context, but rather that this particular stimulus combination has been learned.
Practically nothing is known about odor processing and representation in the honeybee LH. In Drosophila, recent neuroanatomical work could reconstruct putative maps of olfactory input to the LH (Jefferis et al., 2007). In this species, the response spectra of individual ORNs to odors are known (Hallem et al., 2004; Hallem and Carlson, 2006; Galizia et al., 2010) and glomeruli receiving input from ORNs carrying each receptor have been mapped (Couto et al., 2005; Fishilevich and Vosshall, 2005). Moreover, the projection patterns of uniglomerular PNs from identified glomeruli have been retraced to the higher-order centers. The putative olfactory maps at the level of the LH predict a clear segregation between candidate pheromone responsive PNs and fruit odor responsive PNs (Jefferis et al., 2007). Such functional segregation was not apparent in the MBs, although PNs from different glomeruli also project there in at least 17 different areas (Jefferis et al., 2007). Thus, in Drosophila, particular subregions of the LH may code the biological nature of olfactory stimuli. If a similar organization of the olfactory circuit exists in the honeybee, one could expect the honeybee LH to exhibit pheromone processing regionalization. Anatomical and electrophysiological work in ants also confirms this idea (Yamagata and Mizunami, 2010). Moreover, because the LH receives input from associative neurons like Pe-1, it was proposed that it may represent a pre-motor center for both innate biological behavior (pheromones) and acquired behavior (associative learning). Future research should invest more efforts in anatomical and physiological experiments for addressing this question.
Concentration Coding
Odor concentration strongly affects the odor map in the AL as the number of activated glomeruli increases with increasing concentrations of the odor (Sachse and Galizia, 2003). Thus, neurons integrating the overall excitation over many glomeruli, like multiglomerular PNs, may be adequate for monitoring absolute stimulus concentration. But how can odor-specific concentration coding as well as concentration invariance be achieved given the changing nature of the odor representation with concentration? The identity of an odorant is combinatorial and resides more in the relative activation of different glomeruli (or PNs) than in the absolute activation of individual glomeruli (Galizia and Szyszka, 2008). Therefore, neurons recognizing a particular pattern of inputs, such as KCs, could perform both operations, as was shown in locusts (Stopfer et al., 2003): while some KCs were found to be tuned to a narrow concentration range of one particular odorant, other KCs recognized the same odorant on a wide concentration scale. Some concentration invariance can be achieved earlier in the olfactory pathway, mainly through gain control mechanisms. Imaging experiments showed that processing in the AL makes odor representation more reliable over a broader concentration range (Sachse and Galizia, 2003). Moreover, the two PN subsystems may provide differential information to higher-order centers. Imaging recordings of PN boutons in the MB lips showed that while l-APT neurons display low concentration dependency (i.e., concentration invariant representation), m-APT neurons show a clear concentration effect and change their response quickly with concentration (Yamagata et al., 2009). Thus, concentration coding and concentration invariance may be extracted by differential processing at the level of PNs, and/or differential readout by KCs.
Mixture Processing
In vivo calcium imaging at the AL input showed that usually a glomerulus is activated by a mixture when at least one of its components activates this glomerulus (Joerges et al., 1997; Deisig et al., 2006). A putative presynaptic inhibition process induces a gain control at the system’s input, so that complex mixtures do not saturate the capacity of the olfactory system. The more components a mixture contains, the more suppression phenomena were observed (Deisig et al., 2006), i.e., cases in which the response to a mixture was lower than to the components (Duchamp-Viret et al., 2003). Taking into account all measured glomeruli, the whole mixture representation follows essentially an elemental rule, because it can be predicted linearly from the responses to the components: the more a component activates the AL when presented alone (in number of activated glomeruli, for instance), the more present it is in the mixture representation (Deisig et al., 2006). The situation was slightly different at the PN level, as AL processing via LN networks increased the number of suppression cases, allowing the emergence of synthetic properties, i.e., the appearance of a representation that cannot be predicted based only on component information (Deisig et al., 2010). Indeed, similarity relationships between mixtures and their components were more homogeneous than at the input with a more equal representation of weak- and strong-components in the mixture. These recordings showed that reformatting by LNs in the AL increases separability among odor mixture representations, probably facilitating olfactory mixture discrimination by bees (Deisig et al., 2010).
How mixture representation further transforms along the olfactory pathway is mostly unknown. Recordings at the level of PN boutons in the MB lips confirmed an important proportion of suppression effects in l-APT PNs, but showed that such mixture non-linearities are mostly absent in m-APT PNs, providing an additional hypothesis for the functional role of this dichotomous system: one system would be involved in synthetic processing, while the other would conserve component information (Yamagata et al., 2009). The strong sparsening of odor representation from PNs to KCs and their coincidence detection properties could be the basis for mixture-specific units.
Olfactory Plasticity
In bees, olfactory processing is not a static phenomenon, but is subject to plasticity as a function of both age and experience. This plasticity is manifested by structural and functional changes of olfactory circuits.
Developmental Plasticity
The olfactory system of bees goes through intensive remodeling during the pupal stage and metamorphosis. The compartmentations of AL and MB calyces first take place during the beginning of pupal development (Menzel et al., 1994; Hähnlein and Bicker, 1997). At pupal stage 1, the AL neuropil is still homogeneous without any trace of the first spherical neuropil regions, called “preglomeruli” which appear starting at pupal stage 3 (Masson and Arnold, 1984). During subsequent stages the number of preglomeruli progressively increases, so that at pupal stage 7, all glomeruli appear adult-like (Gascuel and Masson, 1991). At the MB level, a small, homogeneously structured neuropil that is not yet divided into subcompartments appears at the prepupal stage (Menzel et al., 1994). Then, starting at pupal stage 3, the calyces gradually become separated from each other, with the lip, collar, and basal ring regions being clearly developed at pupal stage 6 (Hähnlein and Bicker, 1997). Interestingly, PNs achieve their adult arborization pattern within their main output region (MB lip) earlier during development (pupal stage 1) than their dendritic processes within their input region, the AL (pupal stage 2; Schröter and Malun, 2000). The olfactory system remains highly plastic throughout adulthood: an age-dependent increase in neuropil volume is observed for most of the MB, but the lip (olfactory), and collar (visual) regions show both age-related and experience-dependent volume increases (Withers et al., 1993; Durst et al., 1994; Fahrbach et al., 1998; Ismail et al., 2006). Moreover, the density of microglomerular complexes in the lips also undergoes changes with both age and experience (Groh et al., 2006; Krofczik et al., 2008).
The hive is a highly odorous environment, and bees at all ages are subject to constant olfactory stimulation from honey and pollen stores and from pheromones produced by the queen, workers, and brood (Winston, 1987). This olfactory environment can have a significant effect on the maturation of the olfactory system of young bees. A number of experiments attempted to understand the effect of a passive olfactory exposure on honeybees’ behavior. Some studies showed an increase in orientation toward a prior passively exposed odor, both in bees walking in an olfactometer (Pham-Delègue et al., 1990) and in free-flying bees visiting an artificial feeder (Jakobsen et al., 1995). In contrast, in the PER conditioning procedure, no effect of passive exposure was found, or if it was found, exposed bees tended to learn the exposure odor less efficiently than naïve bees (Getz and Smith, 1991; Gerber et al., 1996; Sandoz et al., 2000). At the same time exposed bees were found to spend more time than controls in this odor in a four-armed olfactometer (Sandoz et al., 2000). As control bees tended to avoid the odor, this increased time spent in the exposure odor field was interpreted as a reduced sensitivity of bees after passive exposure. Several processes may explain this effect. For example, constant passive exposure could have induced sensory adaptation of the bees’ olfactory system. This would decrease a spontaneous avoidance by bees of the pure compound in the olfactometer, and make it a less salient compound to be learnt in a PER conditioning procedure. As sensory neurons continue to mature until 8 days after emergence (Masson and Arnold, 1984; Allan et al., 1987), exposure at an early age may permanently alter bees’ olfactory sensitivity.
In another series of experiments, odors were provided mixed with a sucrose solution for different periods during young adulthood (Arenas and Farina, 2008). Bees clearly associated the odor with the sucrose reward and showed long-term memory performance in a PER test at a later stage (17 days). Interestingly, the odor associated with sucrose reward when bees are 5–8 days old resulted in better olfactory retention at the adult stage than when the same exposure was performed before (1–4 days old) or – more surprising – after this critical period (9–12 days old; Arenas and Farina, 2008). In vivo calcium imaging showed that precocious olfactory experience increased general odor-induced activity as well as the number of glomeruli activated by the learned odor in the adult AL, but also affected qualitative odor representations (Arenas et al., 2009b). Thus early olfactory experiences inside the hive may have long-lasting effects, reflected in behavioral responses to odorants and concomitant neural activity in the adult olfactory system. Fitting with the idea of developmental plasticity, bees were found to memorize novel odor-sucrose associations more efficiently after such early experience than controls (Arenas et al., 2009a).
Neural Correlates of Olfactory Learning
During the adult stage, honeybee foragers experience odors in the context of food search, and learn to associate floral odorants with sucrose reward (see above). A number of studies have searched for possible structural and functional plasticity of the olfactory pathway during or at different moments after the formation of an odor-sucrose association. Usually, in such experiments, differential conditioning is used so that changes in neural responses to a reinforced odorant (CS+) can be compared to changes observed to a non-reinforced odorant (CS−). Doing so, several studies found learning-correlated changes in odor-evoked patterns in the AL, taking place either shortly (10–30 min) after differential conditioning (Faber et al., 1999) or later (2–5 h, Rath et al., 2011; 24 h, Sandoz et al., 2003; Fernandez et al., 2009). At short-term, the amplitude of calcium responses to the CS+ were found to increase (Faber et al., 1999). Electrophysiologically, increases and decreases in PN spike rates were found in response to odors after conditioning, with a strongest effect for the CS+ (Denker et al., 2010). Later, between 2 and 6 h after training, differential increases and decreases in the responses of individual glomeruli were found (Rath et al., 2011), which was not the case at shorter-term (Peele et al., 2006). Lastly, at 24 h, PN calcium signals were found to increase to the CS+ (Fernandez et al., 2009). A general observation of these studies was that the similarity between the patterns of the CS+ and of the CS− was decreased after learning, suggesting that olfactory learning improves the discrimination of the learned odorant from other ones (Faber et al., 1999; Fernandez et al., 2009; Rath et al., 2011).
On a structural level, olfactory experience during foraging was shown to induce glomerular volume and structure changes (Sigg et al., 1997; Brown et al., 2002). It was long unclear whether such changes were actually due to olfactory experience per se. Recently, however, a specific glomerular volume increase was demonstrated in a subset of glomeruli as a result of the formation of a long-term appetitive olfactory memory after 72 h (Figures 5A,B; Hourcade et al., 2009). It thus seems that in the AL, learning-induced plasticity takes different forms at different moments after the associative event.
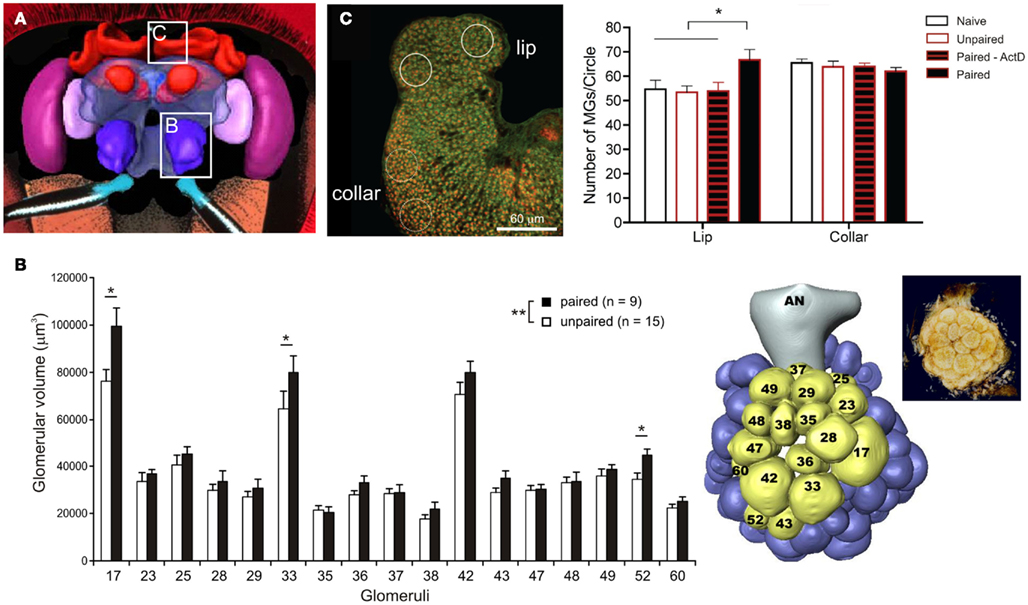
Figure 5. Structural plasticity related to olfactory long-term memory. (A) Structural changes in olfactory circuits of the bee brain were evaluated as a result of the formation of a long-term appetitive olfactory memory. Bees conditioned to an odor CS (paired bees) are compared to bees subjected to pseudoconditioning in which CS and US are presented explicitly without temporal association (unpaired bees). After 72 h, paired but not unpaired bees show strong behavioral olfactory long-term memory. At that time, the brains were prepared and either the volume of olfactory glomeruli in the antennal lobe (B) or the number of microglomeruli in the mushroom body calyx (C) were measured. (B) Volumetric analysis of 17 identified glomeruli in the antennal lobe, based on neutral red staining and 3D reconstruction. A global increase in glomerular volume was found in paired bees relative to unpaired bees. For each of the learned odors (here 1-hexanol), three glomeruli showed a significant volume increase (data from Hourcade et al., 2009). (C) Counts of microglomeruli numbers in the MB calyx, based on synapsin/phalloidin double staining. Olfactory long-term memory induced an increase in microglomeruli numbers in the lip region (olfactory) compared to unpaired or naive bees. This long-term plasticity relies on transcription as injection of Actinomycin D blocked the effect. This structural plasticity related to olfactory long-term memory was logically found only in the calyx lip (olfactory input region) and not in the collar (visual input region). (Data from Hourcade et al., 2010).
Likewise, modified odor-evoked responses to a learned odor were found in the MB calyces shortly after conditioning (10–30 min, Faber and Menzel, 2001; Szyszka et al., 2008). In particular, specific imaging of KC activity showed that repeated presentation of an odor induces a reduction of the evoked response (interpreted as habituation), while appetitive training induced a recovery from this decrease (Szyszka et al., 2008). On a structural level, a long-term olfactory memory trace 72 h after training was revealed as an increase in the density of microglomeruli in the MB lips (Figures 5A,C, Hourcade et al., 2010). MB output neurons are also subject to changes through associative learning, as exemplified by the Pe-1 neuron (Okada et al., 2007), by recurrent PCT neurons (Hähnel and Menzel, 2010) or by other ENs (Strube-Bloss et al., 2011). In some cases too, specific changes are found in responses to the CS+ and response differences between CS+ and CS− were increased (Strube-Bloss et al., 2011).
Thus many electrophysiological, functional imaging, or neuroanatomical studies find strong neural plasticity within olfactory circuits, especially after associative conditioning. However, it is often difficult to relate such neural plasticity to its exact function. Are the observed changes related to modifications of odor processing, modulating the neural representation of the learned odors so that it can be better distinguished from environmental background? Or are they related to an “engram,” revealing the storage of odor-reinforcement associations in the brain? Currently we think that the AL is mostly responsible for the former, while the MB would be crucial for the latter, but considerable work is still needed to confirm this hypothesis. Future neurobiological studies will need a combination of approaches, asking in particular whether the observed cells (and their plasticity) are necessary and/or sufficient for the expression of olfactory plasticity at the behavioral level (Gerber et al., 2004).
Conclusion
One century of experiments have provided extensive data on the olfactory behavior of honeybees, on the neuroanatomical organization of their olfactory pathway as well as on the neural representation of odors within these circuits. All these experiments concur to show that the honeybee olfactory system is tuned for performing a number of operations that are crucial for meeting the demands of social life, food search, and mating. This system thus allows to (1) detect and identify odor stimuli, allowing graded responses to increasingly similar odors; (2) measure stimulus concentration allowing both concentration invariant and concentration-specific odor recognition; (3) detect components within a mixture as well as extract mixture-unique properties; (4) constantly adapt to the odorous environment; and (5) learn relationships between almost any odor and appetitive or aversive outcomes. Although our understanding of odor representation at the different levels of the bee brain has greatly improved in the last years thanks to state-of-the-art recording techniques, entire brain regions have yet to be explored. The most prominent are the m-APT dependent parts of AL and MBs, as well as the utterly unstudied LH. Thanks to optical imaging, our understanding of the spatial representation of odors has greatly improved, but temporal aspects are still poorly understood. Even in such a simple system, as compared to vertebrates, olfactory coding involves complex interactions between different neuron types, so that only computational approaches feeding on comprehensive sets of experimental data may help understanding the dynamics and processing rules of the olfactory system. Lastly, plasticity appears in multiple regions of the olfactory pathway, but their respective implications for tuning the olfactory system or for storing outcome-related memories is still unknown. It shall be the goal of future research to progress in these questions, so that a comprehensive model of olfactory detection, processing, and learning in the honeybee can be constructed, the ultimate goal of sensory neuroscience.
Conflict of Interest Statement
The author declares that the research was conducted in the absence of any commercial or financial relationships that could be construed as a potential conflict of interest.
Acknowledgments
I would like to thank M. H. Pham-Delègue, R. Menzel, M. Giurfa, and C. G. Galizia for their intellectual contribution to the ideas developed in this review. Tilman Franke produced the pictures in Figures 2A,B. This research is financed by the CNRS and by grants from the National Research Agency (ANR-NEURO-2007 and ANR-BLANC-2010) as well as from NERF and R2DS Ile-de-France Programs.
References
Abel, R., Rybak, J., and Menzel, R. (2001). Structure and response patterns of olfactory interneurons in the honeybee, Apis mellifera. J. Comp. Neurol. 437, 363–383.
Ache, B. W., and Young, J. M. (2005). Olfaction: diverse species, conserved principles. Neuron 48, 417–430.
Akers, R. P., and Getz, W. M. (1992). A test of identified response classes among olfactory receptor neurons in the honey-bee worker. Chem. Senses 17, 191–209.
Akers, R. P., and Getz, W. M. (1993). Response of olfactory receptor neurons in honeybees to odorants and their binary mixtures. J. Comp. Physiol. A 173, 169–185.
Allan, S. A., Slessor, K. N., Winston, M. L., and King, G. G. S. (1987). The influence of age and task specialization on the production and perception of honey bee pheromones. J. Insect Physiol. 33, 917–922.
Arenas, A., and Farina, W. M. (2008). Age and rearing environment interact in the retention of early olfactory memories in honeybees. J. Comp. Physiol. A 194, 629–640.
Arenas, A., Fernandez, V. M., and Farina, W. M. (2009a). Associative learning during early adulthood enhances later memory retention in honeybees. PLoS ONE 4, e8046. doi:10.1371/journal.pone.0008046
Arenas, A., Giurfa, M., Farina, W. M., and Sandoz, J. C. (2009b). Early olfactory experience modifies neural activity in the antennal lobe of a social insect at the adult stage. Eur. J. Neurosci. 30, 1498–1508.
Balderrama, N., Núñez, J., Guerrieri, F., and Giurfa, M. (2002). Different functions of two alarm substances in the honeybee. J. Comp. Physiol. A 188, 485–491.
Barbara, G. S., Zube, C., Rybak, J., Gauthier, M., and Grunewald, B. (2005). Acetylcholine, GABA and glutamate induce ionic currents in cultured antennal lobe neurons of the honeybee, Apis mellifera. J. Comp. Physiol. A 191, 823–836.
Barbier, M., and Lederer, E. (1960). Structure chimique de la substance royale de la reine d’abeille Apis mellifera L. C. R. Acad. Sci. Paris 251, 1131–1135.
Benton, R. (2006). On the origin of smell: odorant receptors in insects. Cell. Mol. Life Sci. 63, 1579–1585.
Benton, R., Sachse, S., Michnick, S. W., and Vosshall, L. B. (2006). Atypical membrane topology and heteromeric function of Drosophila odorant receptors in vivo. PLoS Biol. 4, e20. doi:10.1371/journal.pbio.0040020
Bhagavan, S., and Smith, B. H. (1997). Olfactory conditioning in the honey bee Apis mellifera: effects of odor intensity. Physiol. Behav. 61, 107–117.
Bicker, G. (1999). Histochemistry of classical neurotransmitters in antennal lobes and mushroom bodies of the honeybee. Microsc. Res. Tech. 45, 174–183.
Bicker, G., Schäfer, S., and Kingan, T. G. (1985). Mushroom body feedback interneurones in the honeybee show GABA-like immunoreactivity. Brain Res. 360, 394–397.
Bitterman, M. E., Menzel, R., Fietz, A., and Schäfer, S. (1983). Classical conditioning of proboscis extension in honeybees. J. Comp. Psychol. 97, 107–119.
Blight, M. M., Métayer, M. L., Pham-Delègue, M. H., Pickett, J. A., Marion-Poll, F., and Wadhams, L. J. (1997). Identification of floral volatiles involved in recognition of oilseed rape flowers, Brassica napus by honeybees, Apis mellifera. J. Chem. Ecol. 23, 1715–1727.
Boch, R., Shearer, D. A., and Stone, B. C. (1962). Identification of isoamyl acetate as an active component in the sting pheromone of the honey bee. Nature 195, 1018–1020.
Bornhauser, B. C., and Meyer, E. P. (1997). Histamine-like immunoreactivity in the visual system and brain of an orthopteran and a hymenopteran insect. Cell Tissue Res. 287, 211–221.
Brandt, R., Rohlfing, T., Rybak, J., Krofczik, S., Maye, A., Westerhoff, M., Hege, H. C., and Menzel, R. (2005). Three-dimensional average-shape atlas of the honeybee brain and its applications. J. Comp. Neurol. 492, 1–19.
Breed, M. D., Guzman-Novoa, E., and Hunt, G. J. (2004). Defensive behavior of honey bees: organization, genetics, and comparisons with other bees. Annu. Rev. Entomol. 49, 271–298.
Broome, B. M., Jayaraman, V., and Laurent, G. (2006). Encoding and decoding of overlapping odor sequences. Neuron 51, 467–482.
Brown, S. M., Napper, R. M., Thompson, C. M., and Mercer, A. R. (2002). Stereological analysis reveals striking differences in the structural plasticity of two readily identifiable glomeruli in the antennal lobes of the adult worker honeybee. J. Neurosci. 22, 8514–8522.
Butler, C. G., Callow, R. K., and Johnston, N. C. (1962). The isolation and synthesis of queen substance, 9-oxodec-trans-2-enoic acid, a honeybee pheromone. Proc. R. Soc. Lond. B Biol. Sci. 155, 417–432.
Carcaud, J., Roussel, E., Giurfa, M., and Sandoz, J. C. (2009). Odour aversion after olfactory conditioning of the sting extension reflex in honeybees. J. Exp. Biol. 212, 620–626.
Chaffiol, A., Laloi, D., and Pham-Delegue, M. H. (2005). Prior classical olfactory conditioning improves odour-cued flight orientation of honey bees in a wind tunnel. J. Exp. Biol. 208, 3731–3737.
Chandra, S., and Smith, B. H. (1998). An analysis of synthetic processing of odor mixtures in the honeybee (Apis mellifera). J. Exp. Biol. 201, 3113–3121.
Chittka, L., Thomson, J. D., and Waser, N. M. (1999). Flower constancy, insect psychology, and plant evolution. Naturwissenschaften 86, 361–377.
Collins, A. M., and Blum, M. S. (1982). Bioassay of compounds derived from the honeybee sting. J. Chem. Ecol. 8, 463–470.
Couto, A., Alenius, M., and Dickson, B. J. (2005). Molecular, anatomical, and functional organization of the Drosophila olfactory system. Curr. Biol. 15, 1535–1547.
Dahanukar, A., Hallem, E. A., and Carlson, J. R. (2005). Insect chemoreception. Curr. Opin. Neurobiol. 15, 423–430.
Deisig, N., Giurfa, M., Lachnit, H., and Sandoz, J. C. (2006). Neural representation of olfactory mixtures in the honeybee antennal lobe. Eur. J. Neurosci. 24, 1161–1174.
Deisig, N., Giurfa, M., and Sandoz, J. C. (2010). Antennal lobe processing increases separability of odor mixture representations in the honeybee. J. Neurophysiol. 103, 2185–2194.
Deisig, N., Lachnit, H., and Giurfa, M. (2002). The effect of similarity between elemental stimuli and compounds in olfactory patterning discriminations. Learn. Mem. 9, 112–121.
Deisig, N., Lachnit, H., Hellstern, F., and Giurfa, M. (2001). Configural olfactory learning in honeybees: negative and positive patterning discrimination. Learn. Mem. 8, 70–78.
Deisig, N., Lachnit, H., Sandoz, J. C., Lober, K., and Giurfa, M. (2003). A modified version of the unique cue theory accounts for olfactory compound processing in honeybees. Learn. Mem. 10, 199–208.
Denker, M., Finke, R., Schaupp, F., Grun, S., and Menzel, R. (2010). Neural correlates of odor learning in the honeybee antennal lobe. Eur. J. Neurosci. 31, 119–133.
Ditzen, M., Evers, J. F., and Galizia, C. G. (2003). Odor similarity does not influence the time needed for odor processing. Chem. Senses 28, 781–789.
Dobson, H. E. M. (1994) “Floral volatiles in insect biology,” in Insect-Plant Interactions, ed. E. A. Bernays (Boca Raton: CRC Press), 47–81.
Duchamp-Viret, P., Duchamp, A., and Chaput, M. A. (2003). Single olfactory sensory neurons simultaneously integrate the components of an odour mixture. Eur. J. Neurosci. 18, 2690–2696.
Dudareva, N., and Pichersky, E. (2000). Biochemical and molecular genetic aspects of floral scents. Plant Physiol. 122, 627–633.
Dupuis, J. P., Bazelot, M., Barbara, G. S., Paute, S., Gauthier, M., and Raymond-Delpech, V. (2010). Homomeric RDL and heteromeric RDL/LCCH3 GABA receptors in the honeybee antennal lobes: two candidates for inhibitory transmission in olfactory processing. J. Neurophysiol. 103, 458–468.
Durst, C., Eichmuller, S., and Menzel, R. (1994). Development and experience lead to increased volume of subcompartments of the honeybee mushroom body. Behav. Neural Biol. 62, 259–263.
Eisthen, H. L. (2002). Why are olfactory systems of different animals so similar? Brain Behav. Evol. 59, 273–293.
El Hassani, A. K., Giurfa, M., Gauthier, M., and Armengaud, C. (2008). Inhibitory neurotransmission and olfactory memory in honeybees. Neurobiol. Learn. Mem. 90, 589–595.
Esslen, J., and Kaissling, K. E. (1976). Zahl und Verteilung antennaler Sensillen bei der Honigbiene (Apis mellifera L.). Zoomorphologie 83, 227–251.
Faber, T., Joerges, J., and Menzel, R. (1999). Associative learning modifies neural representations of odors in the insect brain. Nat. Neurosci. 2, 74–78.
Faber, T., and Menzel, R. (2001). Visualizing a mushroom body response to a conditioned odor in honeybees. Naturwissenschaften 88, 472–476.
Fahrbach, S. E., Moore, D., Capaldi, E. A., Farris, S. M., and Robinson, G. E. (1998). Experience-expectant plasticity in the mushroom bodies of the honeybee. Learn. Mem. 5, 115–123.
Farina, W. M., Grüter, C., and Diaz, P. C. (2005). Social learning of floral odours inside the honeybee hive. Proc. Biol. Sci. 272, 1923–1928.
Farooqui, T., Robinson, K., Vaessin, H., and Smith, B. H. (2003). Modulation of early olfactory processing by an octopaminergic reinforcement pathway in the honeybee. J. Neurosci. 23, 5370–5380.
Fernandez, P. C., Locatelli, F. F., Person-Rennell, N., Deleo, G., and Smith, B. H. (2009). Associative conditioning tunes transient dynamics of early olfactory processing. J. Neurosci. 29, 10191–10202.
Fishilevich, E., and Vosshall, L. B. (2005). Genetic and functional subdivision of the Drosophila antennal lobe. Curr. Biol. 15, 1548–1553.
Flanagan, D., and Mercer, A. R. (1989a). An atlas and 3-D reconstruction of the antennal lobes in the worker honey bee, Apis mellifera L. (Hymenoptera: Apidae). Int. J. Insect Morphol. Embryol. 18, 145–159.
Flanagan, D., and Mercer, A. R. (1989b). Morphology and response characteristics of neurones in the deutocerebrum of the brain in the honeybee Apis mellifera. J. Comp. Physiol. A 164, 483–494.
Fonta, C., Sun, X. J., and Masson, C. (1993). Morphology and spatial distribution of bee antennal lobe interneurones responsive to odours. Chem. Senses 18, 101–119.
Frings, H. (1944). The loci of olfactory end-organs in the honey-bee, Apis mellifera Linn. J. Exp. Zool. 97, 123–134.
Galizia, C. G. (2008). “Insect olfaction,” in The Senses, A Comprehensive Reference, eds D. V. Smith, S. Firestein, and G. K. Beauchamp (London: Elsevier), 725–769.
Galizia, C. G., and Kimmerle, B. (2004). Physiological and morphological characterization of honeybee olfactory neurons combining electrophysiology, calcium imaging and confocal microscopy. J. Comp. Physiol. A 190, 21–38.
Galizia, C. G., McIlwrath, S. L., and Menzel, R. (1999a). A digital three-dimensional atlas of the honeybee antennal lobe based on optical sections aquired using confocal microscopy. Cell Tissue Res. 295, 383–394.
Galizia, C. G., Sachse, S., Rappert, A., and Menzel, R. (1999b). The Glomerular code for odor representation is species specific in the honeybee Apis mellifera. Nat. Neurosci. 2, 473–478.
Galizia, C. G., and Menzel, R. (2001). The role of glomeruli in the neural representation of odours: results from optical recording studies. J. Insect Physiol. 47, 115–129.
Galizia, C. G., Munch, D., Strauch, M., Nissler, A., and Ma, S. W. (2010). Integrating heterogeneous odor response data into a common response model: A DoOR to the complete olfactome. Chem. Senses 35, 551–563.
Galizia, C. G., Nägler, K., Hölldobler, B., and Menzel, R. (1998). Odour coding is bilaterally symmetrical in the antennal lobe of honeybees (Apis mellifera). Eur. J. Neurosci. 10, 2964–2974.
Galizia, C. G., and Rössler, W. (2010). Parallel olfactory systems in insects: anatomy and function. Annu. Rev. Entomol. 55, 399–420.
Galizia, C. G., and Szyszka, P. (2008). Olfactory coding in the insect brain: molecular receptive ranges, spatial and temporal coding. Entomol. Exp. Appl. 128, 81–92.
Galizia, C. G., and Vetter, R. S. (2004). “Optical methods for analyzing odor-evoked activity in the insect brain,” in Methods in Insect Sensory Neuroscience, ed. T. A. Christensen (Boca Raton: CRC Press), 345–392.
Ganeshina, O., and Menzel, R. (2001). GABA-immunoreactive neurons in the mushroom bodies of the honeybee: an electron microscopic study. J. Comp. Neurol. 437, 335–349.
Gascuel, J., and Masson, C. (1991). Developmental study of afferented and deafferented bee antennal lobes. J. Neurobiol. 22, 795–810.
Gerber, B., Geberzahn, N., Hellstern, F., Klein, J., Kowalksy, O., Wüstenberg, D., and Menzel, R. (1996). Honey bees transfer olfactory memories established during flower visits to a proboscis extension paradigm in the laboratory. Anim. Behav. 52, 1079–1085.
Gerber, B., Tanimoto, H., and Heisenberg, M. (2004). An engram found? Evaluating the evidence from fruit flies. Curr. Opin. Neurobiol. 14, 737–744.
Gerber, B., and Ullrich, J. (1999). No evidence for olfactory blocking in honeybee classical conditioning. J. Exp. Biol. 202, 1839–1854.
Getz, W. M., and Smith, K. B. (1987). Olfactory sensitivity and discrimination of mixtures in the honeybee Apis mellifera. J. Comp. Physiol. A 160, 239–245.
Getz, W. M., and Smith, K. B. (1990). Odorant moiety and odor mixture perception in free-flying honey bees (Apis mellifera). Chem. Senses 15, 111–128.
Getz, W. M., and Smith, K. B. (1991). Olfactory perception in honeybees: concatenated and mixed odorant stimuli, concentration, and exposure effects. J. Comp. Physiol. A 169, 215–230.
Giurfa, M. (2003). Cognitive neuroethology: dissecting non-elemental learning in a honeybee brain. Curr. Opin. Neurobiol. 13, 726–735.
Giurfa, M. (2007). Behavioral and neural analysis of associative learning in the honeybee: a taste from the magic well. J. Comp. Physiol. A 193, 801–824.
Giurfa, M., Fabre, E., Flaven-Pouchon, J., Groll, H., Oberwallner, B., Vergoz, V., Roussel, E., and Sandoz, J. C. (2009). Olfactory conditioning of the sting extension reflex in honeybees: memory dependence on trial number, interstimulus interval, intertrial interval, and protein synthesis. Learn. Mem. 16, 761–765.
Giurfa, M., and Núñez, J. A. (1992). Honeybees mark with scent and reject recently visited flowers. Oecologia 89, 113–117.
Groh, C., Ahrens, D., and Rössler, W. (2006). Environment- and age-dependent plasticity of synaptic complexes in the mushroom bodies of honeybee queens. Brain Behav. Evol. 68, 1–14.
Gronenberg, W. (2001). Subdivision of hymenopteran mushroom body calyces by their afferent supply. J. Comp. Neurol. 436, 474–489.
Grozinger, C. M., Sharabash, N. M., Whitfield, C. W., and Robinson, G. E. (2003). Pheromone-mediated gene expression in the honey bee brain. Proc. Natl. Acad. Sci. U.S.A. 100, 14519–14525.
Grünewald, B. (1999). Morphology of feedback neurons in the mushroom body of the honeybee, Apis mellifera. J. Comp. Neurol. 404, 114–126.
Guerrieri, F., Lachnit, H., Gerber, B., and Giurfa, M. (2005a). Olfactory blocking and odorant similarity in the honeybee. Learn. Mem. 12, 86–95.
Guerrieri, F., Schubert, M., Sandoz, J. C., and Giurfa, M. (2005b). Perceptual and neural olfactory similarity in honeybees. PLoS Biol. 3, e60. doi:10.1371/journal.pbio.0030060
Hähnel, M., and Menzel, R. (2010). Sensory representation and learning-related plasticity in mushroom body extrinsic feedback neurons of the protocerebral tract. Front. Syst. Neurosci. 4:161. doi:10.3389/fnsys.2010.00161
Hähnlein, I., and Bicker, G. (1997). Glial patterning during postembryonic development of central neuropiles in the brain of the honeybee. Dev. Genes Evol. 207, 29–41.
Hallem, E. A., and Carlson, J. R. (2006). Coding of odors by a receptor repertoire. Cell 125, 143–160.
Hallem, E. A., Ho, M. G., and Carlson, J. R. (2004). The molecular basis of odor coding in the Drosophila antenna. Cell 117, 965–979.
Hammer, M. (1993). An identified neuron mediates the unconditioned stimulus in associative olfactory learning in honeybees. Nature 366, 59–63.
Hammer, M., and Menzel, R. (1998). Multiple sites of associative odor learning as revealed by local brain microinjections of octopamine in honeybees. Learn. Mem. 5, 146–156.
Hildebrand, J. G., and Shepherd, G. M. (1997). Mechanisms of olfactory discrimination: converging evidence for common principles across phyla. Annu. Rev. Neurosci. 20, 595–631.
Hoover, S. E., Keeling, C. I., Winston, M. L., and Slessor, K. N. (2003). The effect of queen pheromones on worker honey bee ovary development. Naturwissenschaften 90, 477–480.
Hori, S., Takeuchi, H., Arikawa, K., Kinoshita, M., Ichikawa, N., Sasaki, M., and Kubo, T. (2006). Associative visual learning, color discrimination, and chromatic adaptation in the harnessed honeybee Apis mellifera L. J. Comp. Physiol. A 192, 691–700.
Hosler, J. S., and Smith, B. S. (2000). Blocking and the detection of odor components in blends. J. Exp. Biol. 203, 2797–2806.
Hourcade, B., Muenz, T. S., Sandoz, J. C., Rössler, W., and Devaud, J. M. (2010). Long-term memory leads to synaptic reorganization in the mushroom bodies: a memory trace in the insect brain? J. Neurosci. 30, 6461–6465.
Hourcade, B., Perisse, E., Devaud, J. M., and Sandoz, J. C. (2009). Long-term memory shapes the primary olfactory center of an insect brain. Learn. Mem. 16, 607–615.
Ibbotson, M. R. (2001). Evidence for velocity-tuned motion-sensitive descending neurons in the honeybee. Proc. Biol. Sci. 268, 2195–2201.
Ibbotson, M. R., and Goodman, L. J. (1990). Response characteristics of four wide-field motion-sensitive descending interneurones in Apis mellifera. J. Exp. Biol. 148, 255–279.
Ismail, N., Robinson, G. E., and Fahrbach, S. E. (2006). Stimulation of muscarinic receptors mimics experience-dependent plasticity in the honey bee brain. Proc. Natl. Acad. Sci. U.S.A. 103, 207–211.
Jakobsen, H. B., Kristjansson, K., Rohde, B., Terkildsen, M., and Olsen, C. E. (1995). Can social bees be influenced to choose a specific feeding station by adding the scent of the station to the hive air? J. Chem. Ecol. 21, 1635–1648.
Jefferis, G. S., Potter, C. J., Chan, A. M., Marin, E. C., Rohlfing, T., Maurer, C. R. Jr., and Luo, L. (2007). Comprehensive maps of Drosophila higher olfactory centers: spatially segregated fruit and pheromone representation. Cell 128, 1187–1203.
Joerges, J., Küttner, A., Galizia, C. G., and Menzel, R. (1997). Representations of odours and odour mixtures visualized in the honeybee brain. Nature 387, 285–288.
Johnson, B. A., and Leon, M. (2007). Chemotopic odorant coding in a mammalian olfactory system. J. Comp. Neurol. 503, 1–34.
Jortner, R. A., Farivar, S. S., and Laurent, G. (2007). A simple connectivity scheme for sparse coding in an olfactory system. J. Neurosci. 27, 1659–1669.
Kaissling, K. E. (1987). R. H. Wright Lectures on Insect Olfaction. Burnaby, BC: Simon Fraser University.
Kay, L. M., and Stopfer, M. (2006). Information processing in the olfactory systems of insects and vertebrates. Semin. Cell Dev. Biol. 17, 433–442.
Keeling, C. I., Slessor, K. N., Higo, H. A., and Winston, M. L. (2003). New components of the honey bee (Apis mellifera L.) queen retinue pheromone. Proc. Natl. Acad. Sci. U.S.A. 100, 4486–4491.
Kelber, C., Rössler, W., and Kleineidam, C. J. (2006). Multiple olfactory receptor neurons and their axonal projections in the antennal lobe of the honeybee Apis mellifera. J. Comp. Neurol. 496, 395–405.
Kenyon, F. C. (1896). The brain of the bee – a preliminary contribution to the morphology of the nervous system of the Arthropoda. J. Comp. Neurol. 6, 134–210.
Kirschner, S., Kleineidam, C. J., Zube, C., Rybak, J., Grunewald, B., and Rössler, W. (2006). Dual olfactory pathway in the honeybee, Apis mellifera. J. Comp. Neurol. 499, 933–952.
Knudsen, J. T., Tollsten, L., and Bergström, L. G. (1993). Floral scents – a checklist of volatile compounds isolated by head-space techniques. Phytochemistry 33, 253–280.
Kramer, E. (1976). The orientation of walking honeybees in odour fields with small concentration gradients. Physiol. Entomol. 1, 27–37.
Kreissl, S., Strasser, C., and Galizia, C. G. (2010). Allatostatin immunoreactivity in the honeybee brain. J. Comp. Neurol. 518, 1391–1417.
Kriston, I. (1971). Zum Problem des Lernverhaltens von Apis mellifica L. gegenüber verschiedenen Duftstoffen. Zeit. Vergl. Physiol. 74, 169–189.
Kriston, I. (1973). Die Bewertung von Duft und Farbsignalen als Orientierungshilfen an der Futterquelle durch Apis mellifera L. J. Comp. Physiol. 84, 77–94.
Krofczik, S., Khojasteh, U., Hempel de Ibarra, N., and Menzel, R. (2008). Adaptation of microglomerular complexes in the honeybee mushroom body lip to manipulations of behavioral maturation and sensory experience. Dev. Neurobiol. 68, 1007–1017.
Krofczik, S., Menzel, R., and Nawrot, M. P. (2009). Rapid odor processing in the honeybee antennal lobe network. Front. Comput. Neurosci. 2:9. doi:10.3389/neuro.10.009.2008
Kuwabara, M. (1957). Bildung des bedingten Reflexes von Pavlovs Typus bei der Honigbiene, Apis mellifica. J. Fac. Sci. Hokkaido Univ. Ser. VI Zool. 13, 458–464.
Lacher, V. (1964). Elektrophysiologische Untersuchungen an einzelnen Rezeptoren für Geruch, Kohlendioxyd, Luftfeuchtigkeit und Tempratur auf den Antennen der Arbeitsbiene und der Drohne (Apis mellifica L.). Zeit. Vergl. Physiol. 48, 587–623.
Lacher, V., and Schneider, D. (1963). Elektrophysiologischer Nachweis der Riechfunktion von Porenplatten (Sensilla placodea) auf den Antennen der Drohne und der Arbeitsbiene (Apis mellifera L.). Zeit. Vergl. Physiol. 47, 274–278.
Lachnit, H., Giurfa, M., and Menzel, R. (2004). Odor processing in honeybees: is the whole equal to, more than, or different from the sum of its parts? Adv. Study Behav. 34, 241–264.
Laloi, D., Bailez, O., Blight, M. M., Roger, B., Pham-Delègue, M. H., and Wadhams, L. J. (2000). Recognition of complex odors by restrained and free-flying honeybees, Apis mellifera. J. Chem. Ecol. 26, 2307–2319.
Laska, M., Galizia, C. G., Giurfa, M., and Menzel, R. (1999). Olfactory discrimination ability and odor structure-activity relationships in honeybees. Chem. Senses 24, 429–438.
Laurent, G. (2002). Olfactory network dynamics and the coding of multidimensional signals. Nat. Rev. Neurosci. 3, 884–895.
Le Métayer, M., Marion-Poll, F., Sandoz, J. C., Pham-Delègue, M. H., Blight, M. M., Wadhams, L. J., Masson, C., and Woodcock, C. M. (1997). Effect of conditioning on discrimination of oilseed rape volatiles by the honeybee: use of a combined gas chromatography-proboscis extension behavioural assay. Chem. Senses 22, 391–398.
Leon, M., and Johnson, B. A. (2009). Is there a space-time continuum in olfaction? Cell. Mol. Life Sci. 66, 2135–2150.
Linster, C., Sachse, S., and Galizia, C. G. (2005). Computational modeling suggests that response properties rather than spatial position determine connectivity between olfactory glomeruli. J. Neurophysiol. 93, 3410–3417.
Linster, C., and Smith, B. H. (1997). A computational model of the response of honey bee antennal circuitry to odor mixtures: overshadowing, blocking and unblocking can arise from lateral inhibition. Behav. Brain Res. 87, 1–14.
Lledo, P. M., Gheusi, G., and Vincent, J. D. (2005). Information processing in the mammalian olfactory system. Physiol. Rev. 85, 281–317.
Mandairon, N., and Linster, C. (2009). Odor perception and olfactory bulb plasticity in adult mammals. J. Neurophysiol. 101, 2204–2209.
Marfaing, P., Rouault, J., and Laffort, P. (1989). Effect of the concentration and nature of olfactory stimuli on the proboscis extension of conditioned honey bees Apis mellifera ligustica. J. Insect Physiol. 35, 949–955.
Masse, N. Y., Turner, G. C., and Jefferis, G. S. (2009). Olfactory information processing in Drosophila. Curr. Biol. 19, 700–713.
Masson, C., and Arnold, G. (1984). Ontogeny, maturation and plasticity of the olfactory system in the workerbee. J. Insect Physiol. 30, 7–14.
Masson, C., and Mustaparta, H. (1990). Chemical information processing in the olfactory system of insects. Physiol. Rev. 70, 199–245.
Mauelshagen, J. (1993). Neural correlates of olfactory learning paradigms in an identified neuron in the honeybee brain. J. Neurophysiol. 69, 609–625.
Menzel, R. (1985). “Learning in honey bees in an ecological and behavioral context,” in Experimental Behavioral Ecology, eds B. Hölldobler and M. Lindauer (Stuttgart: G. Fischer Verlag), 55–74.
Menzel, R., Durst, C., Erber, J., Eichmüller, S., Hammer, M., Hildebrandt, H., Mauelshagen, J., Müller, U., Rosenboom, H., Rybak, J., Schäfer, S., and Scheidler, A. (1994). “The mushroom bodies in the honeybee: From molecules to behavior,” in Neural Basis of Behavioral Adaptations. Fortschritte der Zoologie, Vol. 39, eds K. Schildberger and N. Elsner (Stuttgart: Gustav Fischer Verlag), 81–102.
Menzel, R., Greggers, U., and Hammer, M. (1993). “Functional organization of appetitive learning and memory in a generalist pollinator, the honey bee,” in Insect Learning, ed. A. C. Lewis (London: Chapman Hall), 79–125.
Minnich, D. E. (1932). The contact chemoreceptors of the honey bee Apis mellifera. J. Exp. Zool. 61, 375–393.
Mobbs, P. G. (1982). The brain of the honeybee Apis mellifera I. The connections and spatial organization of the mushroom bodies. Philos. Trans. R. Soc. Lond. B 298, 309–354.
Mobbs, P. G. (1984). Neural networks in the mushroom bodies of the honeybee. J. Insect Physiol. 30, 43–58.
Mori, K., Takahashi, Y. K., Igarashi, K. M., and Yamaguchi, M. (2006). Maps of odorant molecular features in the mammalian olfactory bulb. Physiol. Rev. 86, 409–433.
Mota, T., Giurfa, M., and Sandoz, J. C. (2011). Color modulates olfactory learning in honeybees by an occasion-setting mechanism. Learn. Mem. 18, 144–155.
Müller, D., Abel, R., Brandt, R., Zockler, M., and Menzel, R. (2002). Differential parallel processing of olfactory information in the honeybee, Apis mellifera L. J. Comp. Physiol. A 188, 359–370.
Núñez, J., Almeida, L., Balderrama, N., and Giurfa, M. (1998). Alarm pheromone induces stress analgesia via an opioid system in the honeybee. Physiol. Behav. 63, 75–80.
Núñez, J. A., Maldonado, H., Miralto, A., and Balderrama, N. (1983). The stinging response of the honey bee: effects of morphine, naloxone and some opioid peptides. Pharmacol. Biochem. Behav. 19, 921–924.
Okada, R., Rybak, J., Manz, G., and Menzel, R. (2007). Learning-related plasticity in PE1 and other mushroom body-extrinsic neurons in the honeybee brain. J. Neurosci. 27, 11736–11747.
Okada, R., Sakura, M., and Mizunami, M. (2003). Distribution of dendrites of descending neurons and its implications for the basic organization of the cockroach brain. J. Comp. Neurol. 459, 158–174.
Pareto, A. (1972). Die zentrale Verteilung der Fühlerafferenz bei Arbeiterinnen der Honigbiene, Apis mellifera L. Z. Zellforsch. 131, 109–140.
Pearce, J. M. (1987). A model for stimulus generalization in pavlovian conditioning. Psychol. Rev. 94, 61–73.
Pearce, J. M. (1994). Similarity and discrimination: a selective review and a connectionist model. Psychol. Rev. 101, 587–607.
Peele, P., Ditzen, M., Menzel, R., and Galizia, C. G. (2006). Appetitive odor learning does not change olfactory coding in a subpopulation of honeybee antennal lobe neurons. J. Comp. Physiol. A 192, 1083–1103.
Pelz, C., Gerber, B., and Menzel, R. (1997). Odorant intensity as a determinant for olfactory conditioning in honeybees: roles in discrimination, overshadowing and memory consolidation. J. Exp. Biol. 200, 837–847.
Perez-Orive, J., Bazhenov, M., and Laurent, G. (2004). Intrinsic and circuit properties favor coincidence detection for decoding oscillatory input. J. Neurosci. 24, 6037–6047.
Perez-Orive, J., Mazor, O., Turner, G. C., Cassenaer, S., Wilson, R. I., and Laurent, G. (2002). Oscillations and sparsening of odor representations in the mushroom body. Science 297, 359–365.
Pham-Delègue, M. H., Bailez, O., Blight, M. M., Masson, C., Picard-Nizou, A. L., and Wadhams, L. J. (1993). Behavioral discrimination of oilseed rape volatiles by the honeybee Apis mellifera L. Chem. Senses 18, 483–494.
Pham-Delègue, M. H., Etiévant, P., Guichard, E., and Masson, C. (1989). Sunflower volatiles involved in honeybee discrimination among genotypes and flowering stages. J. Chem. Ecol. 15, 329–343.
Pham-Delègue, M. H., Masson, C., Etiévant, P., and Azar, M. (1986). Selective olfactory choices of the honeybee among sunflower aromas: a study by combined olfactory conditioning and chemical analysis. J. Chem. Ecol. 12, 781–793.
Pham-Delègue, M. H., Roger, B., Charles, R., and Masson, C. (1990). Effet d’une pré-exposition olfactive sur un comportement d’orientation en olfactomètre dynamique à quatre voies chez l’abeille (Apis mellifera L.). Insectes Soc. 37, 181–187.
Pickett, J. A., Williams, I. H., and Martin, A. P. (1982). (Z)-11-eicosen-1-ol, an important new pheromonal component from the sting of the honey bee, Apis mellifera L. (Hymenoptera, Apidae). J. Chem. Ecol. 8, 163–175.
Pickett, J. A., Williams, I. H., Martin, A. P., and Smith, M. C. (1980). Nasonov pheromone of the honeybee, Apis mellifera L. (Hymenoptera: Apidae). Part 1. Chemical characterization. J. Chem. Ecol. 6, 425–434.
Rath, L., Galizia, C. G., and Szyszka, P. (2011). Multiple memory traces after associative learning in the honey bee antennal lobe. Eur. J. Neurosci. 34, 352–360.
Reinhard, J., Sinclair, M., Srinivasan, M. V., and Claudianos, C. (2010). Honeybees learn odour mixtures via a selection of key odorants. PLoS ONE 5, e9110. doi:10.1371/journal.pone.0009110
Rescorla, R. A. (1972). “Configural” conditioning in discrete-trial bar pressing. J. Comp. Physiol. Psychol. 79, 307–317.
Rescorla, R. A. (1973). Evidence for “unique stimulus” account of configural conditioning. J. Comp. Physiol. Psychol. 85, 331–338.
Rescorla, R. A., and Wagner, A. R. (1972). “A theory of Pavlovian conditioning: variations in the effectiveness of reinforcement and non-reinforcement.,” in Classical Conditioning II, eds A. Black and W. F. Prokasy (New York: Appleton-Century-Crofts), 64–99.
Robertson, H. M., and Wanner, K. W. (2006). The chemoreceptor superfamily in the honey bee, Apis mellifera: expansion of the odorant, but not gustatory, receptor family. Genome Res. 16, 1395–1403.
Roussel, E., Carcaud, J., Sandoz, J. C., and Giurfa, M. (2009). Reappraising social insect behavior through aversive responsiveness and learning. PLoS ONE 4, e4197. doi:10.1371/journal.pone.0004197
Rybak, J., and Menzel, R. (1993). Anatomy of the mushroom bodies in the honey bee brain: the neuronal connections of the alpha-lobe. J. Comp. Neurol. 334, 444–465.
Rybak, J., and Menzel, R. (1998). integrative properties of the Pe1 neuron, a unique mushroom body output neuron. Learn. Mem. 5, 133–145.
Sachse, S., and Galizia, C. G. (2002). The Role of inhibition for temporal and spatial odor representation in olfactory output neurons: a calcium imaging study. J. Neurophysiol. 87, 1106–1117.
Sachse, S., and Galizia, C. G. (2003). The coding of odour-intensity in the honeybee antennal lobe: local computation optimizes odour representation. Eur. J. Neurosci. 18, 2119–2132.
Sachse, S., Peele, P., Silbering, A. F., Guhmann, M., and Galizia, C. G. (2006). Role of histamine as a putative inhibitory transmitter in the honeybee antennal lobe. Front. Zool. 3:22. doi:10.1186/1742-9994-3-22
Sachse, S., Rappert, A., and Galizia, C. G. (1999). The Spatial representation of chemical structures in the antennal lobe of honeybees: steps towards the olfactory code. Eur. J. Neurosci. 11, 3970–3982.
Sandoz, J. C., Deisig, N., de Brito Sanchez, M. G., and Giurfa, M. (2007). Understanding the logics of pheromone processing in the honeybee brain: from labeled-lines to across-fiber patterns. Front. Behav. Neurosci. 1:5. doi:10.3389/neuro.08.005.2007
Sandoz, J. C., Galizia, C. G., and Menzel, R. (2003). Side-specific olfactory conditioning leads to more specific odor representation between sides but not within sides in the honeybee antennal lobes. Neuroscience 120, 1137–1148.
Sandoz, J. C., Laloi, D., Odoux, J. F., and Pham-Delègue, M. H. (2000). Olfactory information transfer in the honeybee: compared efficiency of classical conditioning and early exposure. Anim. Behav. 59, 1024–1034.
Sandoz, J. C., Pham-Delegue, M. H., Renou, M., and Wadhams, L. J. (2001). Asymmetrical generalisation between pheromonal and floral odours in appetitive olfactory conditioning of the honey bee (Apis mellifera L.). J. Comp. Physiol. A 187, 559–568.
Schäfer, S., and Bicker, G. (1986). Distribution of GABA-like immunoreactivity in the brain of the honeybee. J. Comp. Neurol. 246, 287–300.
Schäfer, S., and Rehder, V. (1989). Dopamine-like immunoreactivity in the brain and suboesophageal ganglion of the honeybee. J. Comp. Neurol. 280, 43–58.
Schiestl, F. P., Ayasse, M., Paulus, H. F., Erdmann, D., and Francke, W. (1997). Variation of floral scent emission and postpollination changes in individual flowers of Ophrys sphegodes subsp. sphegodes. J. Chem. Ecol. 23, 2281–2289.
Schneider, D., and Steinbrecht, R. A. (1968). Checklist of insect olfactory sensilla. Symp. Zool. Soc. Lond. 23, 279–297.
Schröter, U., and Malun, D. (2000). Formation of antennal lobe and mushroom body neuropils during metamorphosis in the honeybee, Apis mellifera. J. Comp. Neurol. 422, 229–245.
Schröter, U., Malun, D., and Menzel, R. (2007). Innervation pattern of suboesophageal ventral unpaired median neurones in the honeybee brain. Cell Tissue Res. 327, 647–667.
Schröter, U., and Menzel, R. (2003). A new ascending sensory tract to the calyces of the honeybee mushroom body, the subesophageal-calycal tract. J. Comp. Neurol. 465, 168–178.
Schürmann, F. W., Elekes, K., and Geffard, M. (1989). Dopamine-like immunoreactivity in the bee brain. Cell Tissue Res. 256, 399–410.
Seeley, T. D. (1982). Adaptive significance of the age polyethism schedule in honeybee colonies. Behav. Ecol. Sociobiol. (Print) 11, 287–293.
Seeley, T. D. (1995). The Wisdom of the Hive – The Social Physiology of Honey Bee Colonies. London: Harvard University Press.
Shearer, D. A., and Boch, R. (1965). 2-heptanone in the mandibular gland secretion of the honey-bee. Nature 206, 530.
Shepard, R. N. (1987). Toward a universal law of generalization for psychological science. Science 237, 1317–1323.
Sigg, D., Thompson, C. M., and Mercer, A. R. (1997). Activity-dependent changes to the brain and behavior of the honey bee, Apis mellifera (L.). J. Neurosci. 17, 7148–7156.
Slessor, K. N., Kaminski, L. A., King, G. G., Borden, J. H., and Winston, M. L. (1988). Semiochemical basis of the retinue response to queen honey bees. Nature 332, 354–356.
Slessor, K. N., Winston, M. L., and Le Conte, Y. (2005). Pheromone communication in the honeybee (Apis mellifera L.). J. Chem. Ecol. 31, 2731–2745.
Smith, B. H. (1991). The olfactory memory of the honeybee Apis mellifera I. Odorant modulation of short- and intermediate-term memory after single-trial conditioning. J. Exp. Biol. 161, 367–382.
Smith, B. H. (1998). Analysis of interaction in binary odorant mixtures. Physiol. Behav. 65, 397–407.
Smith, B. H., and Cobey, S. (1994). The olfactory memory of the honeybee Apis mellifera II. Blocking between odorants in binary mixtures. J. Exp. Biol. 195, 91–108.
Smith, B. H., and Menzel, R. (1989). The use of electromyogram recordings to quantify odourant discrimination in the honey bee, Apis mellifera. J. Insect Physiol. 35, 369–375.
Stopfer, M., Bhagavan, S., Smith, B. H., and Laurent, G. (1997). Impaired odour discrimination on desynchronization of odour-encoding neural assemblies. Nature 390, 70–74.
Stopfer, M., Jayaraman, V., and Laurent, G. (2003). Intensity versus identity coding in an olfactory system. Neuron 39, 991–1004.
Strausfeld, N. J. (2002). Organization of the honey bee mushroom body: representation of the calyx within the vertical and gamma lobes. J. Comp. Neurol. 450, 4–33.
Strausfeld, N. J., Homberg, U., and Kloppenburg, P. (2000). Parallel organization in honey bee mushroom bodies by peptidergic Kenyon cells. J. Comp. Neurol. 424, 179–195.
Strube-Bloss, M. F., Nawrot, M. P., and Menzel, R. (2011). Mushroom body output neurons encode odor-reward associations. J. Neurosci. 31, 3129–3140.
Sun, X. J., Fonta, C., and Masson, C. (1993). Odour quality processing by bee antennal lobe interneurones. Chem. Senses 18, 355–377.
Suzuki, H. (1975). Convergence of olfactory inputs from both antennae in the brain of the honeybee. J. Exp. Biol. 62, 11–26.
Szyszka, P., Ditzen, M., Galkin, A., Galizia, C. G., and Menzel, R. (2005). Sparsening and temporal sharpening of olfactory representations in the honeybee mushroom bodies. J. Neurophysiol. 94, 3303–3313.
Szyszka, P., Galkin, A., and Menzel, R. (2008). Associative and non-associative plasticity in kenyon cells of the honeybee mushroom body. Front. Syst. Neurosci. 2:3. doi:10.3389/neuro.06.003.2008
Tollsten, L., and Bergström, G. (1989). Headspace volatiles of whole plants and macerated plant parts of Brassica and Sinapis. Nord. J. Bot. 9, 359–362.
Touhara, K., and Vosshall, L. B. (2009). Sensing odorants and pheromones with chemosensory receptors. Annu. Rev. Physiol. 71, 307–332.
Vareschi, E. (1971). Duftunterscheidung bei der Honigbiene – Einzelzell-Ableitungen und Verhaltensreaktionen. Zeit. Vergl. Physiol. 75, 143–173.
Vergoz, V., Roussel, E., Sandoz, J. C., and Giurfa, M. (2007). Aversive learning in honeybees revealed by the olfactory conditioning of the sting extension reflex. PLoS ONE 2, e288. doi:10.1371/journal.pone.0000288
von Frisch, K. (1919). Über den Geruchsinn der Biene und seine blütenbiologische Bedeutung. Zool. Jahrb. Physiol. 37, 1–238.
von Frisch, K. (1967). The Dance Language and Orientation of Bees. Cambridge, MA: Harvard University Press.
Vosshall, L. B., Wong, A. M., and Axel, R. (2000). An olfactory sensory map in the fly brain. Cell 102, 147–159.
Wadhams, L. J., Blight, M. M., Kerguelen, V., Métayer, M. L., Marion-Poll, F., Masson, C., Pham-Delègue, M. H., and Woodcock, C. M. (1994). Discrimination of oilseed rape volatiles by honey bee: novel combined gas chromatographic-electrophysiological behavioral assay. J. Chem. Ecol. 20, 3221–3231.
Whitlow, J. W., and Wagner, A. R. (1972). Negative patterning in classical conditioning: summation of response tendencies to isolable and configural components. Psychon. Sci. 27, 299–301.
Withers, G. S., Fahrbach, S. E., and Robinson, G. E. (1993). Selective neuroanatomical plasticity and division of labour in the honeybee. Nature 364, 238–240.
Wright, G. A., Carlton, M., and Smith, B. H. (2009). A honeybee’s ability to learn, recognize, and discriminate odors depends upon odor sampling time and concentration. Behav. Neurosci. 123, 36–43.
Wright, G. A., Skinner, B. D., and Smith, B. H. (2002). Ability of honeybee, Apis mellifera, to detect and discriminate odors of varieties of canola (Brassica rapa and Brassica napus) and snapdragon flowers (Antirrhinum majus). J. Chem. Ecol. 28, 721–740.
Wright, G. A., and Smith, B. H. (2004). Different thresholds for detection and discrimination of odors in the honey bee (Apis mellifera). Chem. Senses 29, 127–135.
Wright, G. A., Thomson, M. G., and Smith, B. H. (2005). Odour concentration affects odour identity in honeybees. Proc. Biol. Sci. 2417–2422.
Yamagata, N., and Mizunami, M. (2010). Spatial representation of alarm pheromone information in a secondary olfactory centre in the ant brain. Proc. Biol. Sci. 4, 28.
Keywords: olfaction, neural processing, perception, appetitive learning, optical imaging, electrophysiology, brain circuits, insect
Citation: Sandoz JC (2011) Behavioral and neurophysiological study of olfactory perception and learning in honeybees. Front. Syst. Neurosci. 5:98. doi: 10.3389/fnsys.2011.00098
Received: 18 August 2011;
Accepted: 16 November 2011;
Published online: 08 December 2011.
Edited by:
Milagros Gallo, University of Granada, SpainReviewed by:
Milagros Gallo, University of Granada, SpainMonique Gauthier, University Paul Sabatier Toulouse 3, France
Copyright: © 2011 Sandoz. This is an open-access article distributed under the terms of the Creative Commons Attribution Non Commercial License, which permits non-commercial use, distribution, and reproduction in other forums, provided the original authors and source are credited.
*Correspondence: Jean Christophe Sandoz, Evolution, Genomes and Speciation Lab, Centre National de la Recherche Scientifique, 1 Avenue de la Terrasse, 91198 Gif-sur-Yvette, France. e-mail: sandoz@legs.cnrs-gif.fr