- 1Natural Sciences and Science Education, National Institute of Education, Nanyang Technological University, Singapore, Singapore
- 2Department of Biological Sciences, National University of Singapore, Singapore, Singapore
The giant mudskipper, Periophthalmodon schlosseri, is an amphibious fish that builds burrows in the mudflats. It can actively excrete ammonia through its gills, and tolerate high environmental ammonia. This study aimed to examine the effects of seawater (salinity 30; SW) acclimation and/or environmental ammonia exposure on the kinetic properties of Na+/K+-ATPase (Nka) from, and mRNA expression and protein abundance of nka/Nka α–subunit isoforms in, the gills of P. schlosseri pre-acclimated to slightly brackish water (salinity 3; SBW). Our results revealed that the Nka from the gills of P. schlosseri pre-acclimated to SBW for 2 weeks had substantially higher affinity to (or lower Km for) K+ than NH+4, and its affinity to NH+4 decreased significantly after 6-days exposure to 75 mmol l−1 NH4Cl in SBW. Hence, Nka transported K+ selectively to maintain intracellular K+ homeostasis, instead of transporting NH+4 from the blood into ionocytes during active NH+4 excretion as previously suggested. Two nkaα isoforms, nkaα1 and nkaα3, were cloned and sequenced from the gills of P. schlosseri. Their deduced amino acid sequences had K+ binding sites identical to that of Nkaα1c from Anabas testudineus, indicating that they could effectively differentiate K+ from NH+4. Six days of exposure to 75 mmol l−1 NH4Cl in SBW, or to SW with or without 50 mmol l−1 NH4Cl led to significant increases in Nka activities in the gills of P. schlosseri. However, a significant increase in the comprehensive Nkaα protein abundance was observed only in the gills of fish exposed to 50 mmol l−1 NH4Cl in SW. Hence, post-translational modification could be an important activity modulator of branchial Nka in P. schlosseri. The fast modulation of Nka activity and concurrent expressions of two branchial nkaα isoforms could in part contribute to the ability of P. schlosseri to survive abrupt transfer between SBW and SW or abrupt exposure to ammonia.
Introduction
Majority of fully aquatic fishes are ammonotelic, excreting more than 50% of their nitrogenous waste as ammonia-N through their gills. Air-breathing is one of the several adaptive responses utilized by fishes dwelling in habitats with low O2 tension (Graham, 1997). With the development of air-breathing abilities, some fishes can emerge from water, make excursions on land, or even burrow into mud during droughts. Air-breathing fishes would experience difficulties in excreting ammonia during emersion or exposure to puddles of water with high concentrations of ammonia. Since ammonia is toxic, air-breathing fishes adopt various strategies to ameliorate ammonia toxicity during emersion or ammonia exposure (Ip et al., 2001a, 2004a,b; Chew et al., 2006; Ip and Chew, 2010; Chew and Ip, 2014). Theoretically, the most effective strategy to defend against ammonia toxicity is to actively excrete ammonia, which would maintain a low internal ammonia concentration and prevent the brain from ammonia intoxication. Indeed, three air-breathing tropical fishes, Periophthalmodon schlosseri (Randall et al., 1999; Chew et al., 2003, 2007), Anabas testudineus (Tay et al., 2006; Ip et al., 2012a,b; Loong et al., 2012) and Clarias gariepinus; (Ip et al., 2004c) are capable of active ammonia excretion through their gills.
The giant mudskipper, P. schlosseri, belongs to Class Actinopterygii, Order Perciformes and Family Gobiidae. It is carnivorous and is the only species of mudskippers not found outside the tropics. It inhabits muddy shores in estuaries and in the intertidal zone of rivers, where salinity fluctuates twice daily during high and low tides, in South East Asia. It is an osmoregulator and maintains its plasma osmolality at 260–280 mosmolal in a hypoosmotic or hyperosmotic external medium (Chew and Ip, unpublished results). Periophthalmodon schlosseri can survive terrestrial exposure better than other species of mudskippers (Ip et al., 1993; Kok et al., 1998) because it has branched gill filaments with intrafilamentous interlamellar fusions (Low et al., 1988, 1990; Wilson et al., 1999, 2000). The interlamellar fusions form fenestrae which trap water and prevent dehydration when the fish is on land (Low et al., 1988). Naturally, these fusions render aquatic respiration ineffective, but they would facilitate continual ammonia excretion during emersion (Chew et al., 2007). When P. schlosseri is exposed to air, ammonia is actively excreted into the small amount of water trapped in the fenestrae, and it builds up quickly therein, reaching very high concentrations (~30 mmol l−1; Chew et al., 2007). The ability to actively excrete ammonia against inwardly directed PNH3 and NH+4 concentration gradient also confers P. schlosseri the ability to tolerate high concentrations of environmental ammonia (Peng et al., 1998). Unlike many other teleosts which cannot bear 1–5 mmol l−1 NH4Cl (concentrations which can hardly be regarded as “high environmental ammonia” in the literature) for more than a few hours, P. schlosseri can survive in 100 mmol l−1 NH4Cl in water of salinity 15 for more than a week (Peng et al., 1998). As P. schlosseri can actively excrete ammonia, it can maintain low concentrations of ammonia in its plasma when exposed to environmental ammonia, (Peng et al., 1998; Randall et al., 1999) and an unchanged ammonia excretion rate when exposed to alkaline water (Chew et al., 2003).
Active ammonia excretion in P. schlosseri can be inhibited by ouabain or amiloride. Thus, Randall et al. (1999) suggested that NH+4 was transported from the blood into the ionocyte by the basolateral Na+/K+-ATPase (Nka) with NH+4 substituting for K+, and from the ionocyte to the external medium by the apical Na+/H+ (NH+4) exchanger. However, if NH+4 were to be actively transported into ionocytes through basolateral Nka (Weihrauch et al., 2009; Hwang et al., 2011), it must have comparable Km values and be able to effectively replace K+ to activate Nka. In that case, it would be difficult for the ionocyte to maintain high intracellular [K+] to uphold intracellular K+ homeostasis and low [NH+4] to minimize ammonia cytotoxicity. The fundamental principle is that ammonia is cytotoxic and therefore needs to be excreted instead of allowing its intracellular concentration to rise (Ip and Chew, 2010). More importantly, active excretion of NH+4 naturally involves the transport of NH+4 against an electrochemical gradient across the apical, and not the basolateral, membrane of ionocytes. With active excretion of NH+4 to the external medium through the apical membrane, the intracellular ammonia concentration would logically be maintained at low and sub-toxic levels. Moreover, the basolateral membrane generally has an electrical potential of ~75 mV (inside negative; Wright, 1991); hence unlike K+ which has a very high intracellular concentration (~120 mmol l−1), NH+4 does not need to be actively transported across the basolateral membrane through Nka.
The climbing perch, A. testudineus, is an obligate air-breather and a euryhaline freshwater teleost capable of progressive acclimation from fresh water to seawater (Chang et al., 2007), migration on land, and active excretion of ammonia during emersion or ammonia exposure (Tay et al., 2006). Exposure of A. testudineus to ammonia in fresh water results in a significant increase in the mRNA expression and protein abundance of Na+:K+:2Cl− cotransporter 1a (Nkcc1a) in its gills (Loong et al., 2012). Hence, it is probable that NH+4 can enter ionocytes through the basolateral Nkcc1a, in substitution of K+, before being actively transported across the apical membrane (Loong et al., 2012). Since increased activity of Nkcc1a would lead to an increased influx of Na+ and NH+4 into ionocytes, an up-regulation of branchial Nka activity and an increase in its selectivity for K+ over NH+4 are necessary to maintain the electrochemical potential gradients of Na+ and K+ during active ammonia excretion (Ip et al., 2012a). NKA/Nka coordinates the active transport of 3 Na+ out of and 2 K+ into, the cell, fuelled by the hydrolysis of ATP. It contains 2 major subunits, α and β, and functions as an αβ heterodimer. The α-subunit is a large (110–120 kDa) protein that contains all the functional sites and is responsible for the catalytic functioning of the enzyme (Blanco and Mercer, 1998). Four isoforms of the NKA α-subunit (α1, α2, α3, α4) have been identified in mammals (Blanco and Mercer, 1998). For A. testudineus, 3 nkaα isoforms (α1a, α1b, and α1c) have been cloned and sequenced from its gills recently (Ip et al., 2012a). The mRNA expression of nkaα1a is down-regulated in the gills of A. testudineus acclimated to seawater, indicating that it is a freshwater-isoform involving in branchial Na+ absorption in a hypoosmotic environment (Ip et al., 2012a). By contrast, seawater acclimation leads to an up-regulation of the mRNA expression of nkaα1b and to a lesser extent nkaα1c, indicating that they are the seawater-isoforms essential for ion secretion in a hyperosmotic environment. More importantly, exposure of A. testudineus to ammonia in fresh water leads to a significant increase in the mRNA expression of nkaα1c, indicating that it plays an important role in active ammonia excretion (Ip et al., 2012a). Since there is a decrease in the effectiveness of NH+4 to substitute for K+ in the activation of Nka from the gills of A. testudineus exposed to ammonia as compared to the freshwater control (Ip et al., 2012a), the up-regulation of nka α1c expression probably serves to remove excess Na+ from, and to transport K+ in preference to NH+4 into, ionocytes. Similarly, it is probable that the main function of branchial Nka in active NH+4 excretion in P. schlosseri is to maintain intracellular Na+ and K+ homeostasis, instead of transporting NH+4 directly into ionocytes as previously proposed (Randall et al., 1999; Weihrauch et al., 2009; Hwang et al., 2011).
Therefore, the first objective of this study was to determine the kinetic properties of Nka from the gills of P. schlosseri pre-acclimated to slightly brackish water (salinity 3; SBW) for 2 weeks. We hypothesized that NH+4 could not effectively substitute for K+ to induce Nka activity and therefore the Km for NH+4 would be substantially greater than that for K+. The second objective was to examine the effects of 6-days exposure to ammonia (75 mmol l−1 NH4Cl) in SBW, seawater (salinity 30; SW), or ammonia (50 mmol l−1 NH4Cl) in SW on the Km and the Vmax of branchial Nka. The hypothesis tested was that kinetic properties of branchial Nka from P. schlosseri would be altered not only by seawater acclimation but also by exposure to ammonia in SBW or SW. The third objective was to clone and sequence nkaα isoforms from the gills of P. schlosseri, so as to characterize the Na+ and K+ binding sites of the deduced Nkaα sequences and to compare their K+ binding sites with those of the 3 Nkaα isoforms from A. testudineus. Since NKA/Nka activity is known to be regulated through transcription, translation and/or covalent modification, the fourth objective was to determine the effects of 6-days exposure to ammonia (75 mmol l−1 NH4Cl) in SBW, SW, or ammonia (50 mmol l−1 NH4Cl) in SW on the mRNA expression of nkaα isoforms and the comprehensive protein abundance of Nkaα in the gills of P. schlosseri pre-acclimated to SBW. Being a euryhaline brackish water fish, P. schlosseri can survive abrupt transfer between SBW (or water of salinity as low as 1) and SW without mortality. Hence, we hypothesized that, unlike A. testudineus (Ip et al., 2012a), there might not be a clear delineation of freshwater- and seawater-types of nkaα isoforms in the gills of P. schlosseri, and the up-regulation of branchial Nka activity during exposure to SW and/or ammonia might not result from large increases in mRNA and/or protein expression, which are relatively slow processes.
Two different types of abbreviations of genes/proteins have been adopted in this report, as the standard abbreviations of genes/proteins of fishes (http://zfin.org/cgi-bin/webdriver?MIval=aa-ZDB_home.apg) are different from those of human/ non-human primates (http://www.genenames.org). For fishes, gene symbols are italicized, all in lower case, and protein designations are the same as the gene symbol, but not italicized with the first letter in upper case. The advantage of using two types of abbreviations appropriately is that it would allow immediate interpretation of the affiliation between the abbreviated gene/protein and fishes or human/non-human primates.
Materials and Methods
Fish
Specimens of P. schlosseri (90–100 g body mass) were purchased from fishermen at Benut, Malaysia, imported to Singapore, and transferred to the National Institute of Education, Nanyang Technological University. The lowest salinity during low tides and the highest salinity during high tides recorded in the natural habitat of P. schlosseri at Benut, Malaysia were salinity 3 (pH 6.8–7.4) and salinity 30 (pH 7.8–8.3), respectively. Procedures adopted in this study were approved by the Institutional Animal Care and Use Committee (IACUC) of the Nanyang Technological University (ARF SBS/NIE-A-0122 AZ).
No attempt was made to separate the sexes. Fish were maintained in SBW at salinity 3 in individual plastic aquaria (L29 × W19 × H17.5 cm) at 25–27°C under a 12 h light:12 h dark regime. Salinity was monitored using a YSI Model 30/10 FT salinometer (Yellow Springs Instrument Co. Inc, Ohio, USA). No aeration was provided because P. schlosseri is an obligate air-breather. Since specimens of P. schlosseri were obtained from the estuaries where salinity fluctuated twice daily during high and low tides, they might have high branchial nka/Nka expression. Therefore, fish were pre-acclimated to SBW for 2 weeks before being exposed to experimental conditions. SBW had an osmolality of ~80 mosmolal, and was hypoosmotic to the plasma osmolality (260–280 mosmolal) of P. schlosseri. Hence, similar to freshwater fishes, P. schlosseri would have to undergo hyperosmotic regulation to prevent water influx and ion depletion during long-term exposure to SBW. The assumption was that 2 weeks of pre-acclimation in a hypoosmotic medium would be adequate to obtain the baseline expression levels of nka/Nka in the gills of P. schlosseri. During the pre-acclimation period, P. schlosseri was fed fish meat and water was changed daily.
Experimental Conditions and Collection of Samples
After pre-acclimation to SBW for 2 weeks, fish were divided into 3 groups (total N = 24). In the first group of fish, 4 fish were placed in SBW (control) while the other 4 fish were immersed in 20 volumes (v/w) of SBW (pH 6.8–7.0) containing 75 mmol l−1 NH4Cl (pH 7.0) for 6 days. For the second group of fish, again 4 fish were placed in SBW (control) while the other 4 fish were immersed in 20 volumes (v/w) of SW (pH 8.2) for 6 days. Salinity was monitored as mentioned above. SW was made from Red Sea salt (Houston, TX, USA) and aerated for 24 h in order to obtain a stabilized pH of 8.2 before usage. In order to imitate the physiological condition in the natural habitat of P. schlosseri, no attempt was made to adjust the pH of SW. For the third group of fish, 4 fish were immersed in SW (control) while another 4 fish were immersed in 20 volumes (v/w) of SW containing 50 mmol l−1 NH4Cl (pH 8.0) for 6 days. Preliminary studies revealed that while P. schlosseri could survive in 75 mmol l−1 NH4Cl at pH 7.0 in SBW for an extended period (>2 weeks), it would succumb after 2- to 3-days exposure to 75 mmol l−1 NH4Cl at pH 8.0 in SW. Of note, the ratio of NH3 to NH+4 at pH 8.0 in SW would be greater than that at pH 7.0 in SBW. As NH3 is the predominant species of ammonia that permeate biomembranes, ammonia toxicity increases with increasing environmental pH. Therefore, the concentration of NH4Cl was lowered to a sub-lethal concentration of 50 mmol l−1 NH4Cl (pH 8.0) in SW.
Fish were fed with fish meat during the experimental period, and water was changed daily. No mortality was recorded for fish kept in all conditions. After 6 days, fish were killed with an overdose of neutralized MS-222 (0.2%) followed with a strong blow to the head. Gills were sampled and suspended in 1 ml of solution containing 0.1 mol l−1 imidazole-HCl (pH 7.2), 0.3 mol l−1 sucrose, 0.02 mol l−1 EDTA following the method of Zaugg (1982) for the Nka activity assays and western blotting studies. Tissues collected from all four gill arches constituted one sample. Samples were frozen in liquid nitrogen and stored at −80°C until analyses.
Gill filaments needed for molecular studies were collected from a separate group of fish (total N = 40). Fish that have been pre-acclimated to SBW for 2 weeks served as controls (N = 4). After 2 weeks of pre-acclimation to SBW, a group of experimental fish (N = 4 for each time point) was immersed in 20 volumes (v/w) of SBW containing 75 mmol l−1 NH4Cl (pH 7.0) for 1, 2, or 6 days with daily changes of NH4Cl solution. A second group of experimental fish (N = 4 for each time point) was immersed in 20 volumes (v/w) of SW (pH 8.2) for 1, 2, or 6 days with daily changes of SW. A third group of experimental fish (N = 4 for each time point) was immersed in 20 volumes (v/w) of SW containing 50 mmol l−1 NH4Cl (pH 8.0) for 1, 2, or 6 days with daily changes of NH4Cl solution. Fish were fed fish meat daily during the experimental period. Gill filaments from both the control and experimental fish were excised, frozen in liquid N2, and stored at −80°C until analyses.
Determination of Nka Activity
The gill sample collected by the method of Zaugg (1982) was thawed on ice and homogenized for 2 s at 7000 rpm. The homogenate was then centrifuged at 2000 × g for 7 min at 4°C to obtain the pellet. The pellet was re-suspended in 1 ml of homogenizing buffer containing 0.1 mol l−1 imidazole-HCl (pH 7.2), 0.3 mol l−1 sucrose, and 1 g l−1 of sodium deoxycholate (without EDTA, which interfered with the subsequent phosphate analysis), and homogenized twice at 13,500 rpm for 10 s each with an interval of 10 s. The homogenized sample was centrifuged for 6 min at 2000 × g and 4°C. The supernatant obtained was assayed for Nka activity on the same day. The protein content of the sample was determined according to the method of Bradford (1976). The quantities of protein used per assay were approximately 0.23, 0.1, and 0.2 mg for samples from SBW control, seawater-acclimated and ammonia-exposed fish, respectively.
The optimized reaction mixture for the determination of Nka activity (Vsat) contained 0.05 ml sample, 30 mmol l−1 imidazole-HCl buffer (pH 7.2), 120 mmol l−1 NaCl, 20 mmol l−1 KCl, 5 mmol l−1 MgCl2 and 3.5 mmol l−1 ATP, with or without 2 mmol l−1 ouabain in a total volume of 1 ml. The compositions of Na+ and K+ (or NH+4 in replacement of K+) were varied in order to obtained the Km for various substrates (Na+, K+, and NH+4) and the corresponding Vmax. The reaction mixture without ATP was pre-incubated at 25°C for 10 min and the reaction was initiated by the addition of 0.05 ml of ATP. After 40 min of incubation at 25°C, the reaction was terminated by the addition of 0.05 ml of ice-cold 100% trichloroacetic acid. The mixture was centrifuged at 12,000 × g for 2 min at 4°C. Preliminary results indicate that the Nka activity increased linearly with time up to at least 45 min under all conditions. The amount of inorganic phosphate (Pi) released from ATP during the incubation period represented the activity of Nka. An aliquot (0.4 ml) of the supernatant was diluted with 4 volumes of 0.1 mol l−1 sodium acetate for Pi assay. To this diluted aliquot, 0.2 ml of 1% ascorbic acid and 0.2 ml of 1% ammonium molybdate in 0.05 mol l−1 H2SO4 were added. The solution was incubated for 30 min at 30°C and the absorbance was determined at 700 nm using a Shimadzu (Kyoto, Japan) UV160 UV-VIS spectrophotometer, and the Pi concentration calculated with reference to a standard made from K2HPO4 and assayed in the presence of trichloroacetic acid and sodium acetate. The Na+/K+-ATPase or Na+/NH+4-ATPase activity was calculated as a difference of activities assayed in the presence and absence of ouabain. The activity of Nka is expressed as μmol Pi released min−1 mg−1 protein.
To obtain Km and Vmax for Na+, effects of different concentrations of Na+ (5, 10, 20, and 120 mmol l−1) on Nka activity were determined in the presence of 20 mmol l−1 K+ for all samples. To obtain Km and Vmax for K+ or NH+4, effects of varying K+ or NH+4 concentrations (1, 2.5, 5, and 20 mmol l−1) on the Na+/K+-ATPase or Na+/NH+4-ATPase activity were determined at 120 mmol l−1 Na+. The Km and Vmax values were obtained from the Lineweaver-Burk plot. Since Lineweaver-Burk plots are very susceptible to biases at low and high substrate concentrations, results were further analyzed by the Woolf–Augustinsson plot, which also produced comparable Km and Vmax values, and confirmed the differences observed between experimental conditions. Since three Vmax values were generated for each samples based on varied Na+, K+, or NH+4 concentrations, and since they were generally in agreement with the Vsat value obtained under optimized assay conditions, Vsat values were reported instead of Vmax.
Total RNA Extraction and cDNA Synthesis
Total RNA was extracted from the gill samples collected using Tri Reagent™ (Sigma-Aldrich Co., St. Louis, MO, USA) and further purified using the RNeasy Plus Mini Kit (Qiagen GmbH, Hilden, Germany). The RNA obtained was quantified using a NanoDrop ND-1000 spectrophotometer (Nanodrop Technologies Inc., Wilmington, DE, USA) and its integrity was verified by electrophoresis. The total RNA (1 μg) isolated was reverse transcribed into first strand cDNA using oligo (dT)18 primers and the RevertAid™ First Strand cDNA synthesis kit (Thermo Fisher Scientific Inc. Waltham, MA, USA).
Polymerase Chain Reaction (PCR), Cloning, and RACE-PCR
The partial nka α-subunit sequences were obtained using primers (Forward: 5′-CACTTCATCCACATCATCAC-3′ and Reverse: 5′-ATGGCGGGAACCATGTC-3′) designed according to conserved regions of Anguilla anguilla nkaα (X76108), Fundulus heteroclitus nkaα1 (AY057072), Oncorhynchus mykiss nkaα1a (AY319391), Oreochromis mossambicus nkaα1 (TMU82549), F. heteroclitus nkaα2 (AY057073), O. mykiss nkaα2 (NM_001124458), O. mykiss nkaα3 (NM_001124630) and O. mossambicus nkaα3 (AF109409). Notably, using the same pair of primers, 3 nkaα1 isoforms (α1a, α1b, and α1c) have been cloned from the gills of A. testudineus (Ip et al., 2012a), and 3 nkaα isoforms (α1, α2, and α3) have been cloned from the gills of the euryhaline freshwater stingray, Himantura signifer (Ip et al., 2013), and the brain of the African lungfish, Protopterus annectens (Hiong et al., 2014). In addition, this pair of primers was able to amplify nkaα1, nkaα3a and nkaα3b from the brain of the swamp eel, Monopterus albus (Chen et al., 2013).
The PCR was performed in a 9902 Veriti 96-well thermal cycler (Applied Biosystems, Carlsbad, CA, USA) using DreamTaq™ polymerase (Thermo Fisher Scientific Inc.). The cycling conditions were 94°C (3 min), followed by 35 cycles at 94°C (30 s), 50°C (30 s), and 72°C (2 min), and one cycle of final extension at 72°C (7 min). The PCR products obtained were separated by electrophoresis in 1% agarose gel. Bands of the estimated size (1500–1600 bp) were extracted from the gels using Promega Wizard SV gel and PCR cleanup system (Promega Corporation, Madison, WI, USA). The PCR products were cloned into pGEM®-T Easy vector (Promega Corporation). The ligated vector was transformed into JM109 competent cells and plated onto Luria-Bertani (LB) agar with 100 μg ml−1 ampicillin, 50 μg ml−1 X-gal and 0.5 mmol l−1 IPTG. Selected white colonies were grown overnight in LB with ampicillin. The plasmids were extracted using the resin-based plasmid miniprep kit (Axygen Biosciences, Union city, CA, USA).
The plasmids were sequenced in both directions using the BigDye® Terminator v3.1 Cycle Sequencing Kit (Applied Biosystems) with a 3130XL Genetic Analyzer (Applied Biosystems). The sequence data generated was viewed and analyzed using BioEdit version 7.0.9 (Hall, 1999). Analysis of multiple clones of nkaα fragment with the nucleotide database of National Center for Biotechnology Information (http://blast.ncbi.nlm.nih.gov/Blast.cgi) revealed the presence of two nka α-subunit isoforms in the gills of P. schlosseri.
The complete cDNA sequences of the two nka α-subunit isoforms were obtained using SMART™ RACE cDNA amplification kit (Clontech Laboratories, Mountain View, CA, USA) and 5′ and 3′ RACE primers (Table 1) designed. The cDNA sequences of nka α-subunit isoforms obtained were assembled and analysed using BioEdit and the complete sequences were deposited into GenBank.
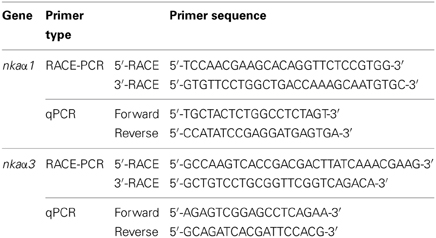
Table 1. Primers used for RACE and quantitative real-time PCR (qPCR) of Na+/K+-ATPase (nka) α-subunit isoforms from gills of Periophthalmodon schlosseri.
Deduced Amino acid Sequence and Phylogenetic Analysis
The amino acid sequences of the two Nka α-subunits were translated from the nucleotide sequences of nka α-subunits using ExPASy Proteomic server (http://web.expasy.org/translate/) (Gasteiger et al., 2003). The transmembrane domains of the translated amino acid sequences of both Nka α-subunit isoforms were identified using MEMSAT3 & MEMSAT-SVM provided by PSIPRED protein structure prediction server (http://bioinf.cs.ucl.ac.uk/psipred/) (McGuffin et al., 2000).
The identity of amino acid sequences of both Nkaα isoforms from P. schlosseri was confirmed by comparing with various Nkaα sequences for other animals species available in the Genbank and obtaining percentage similarities based on ClustalX2 and Bioedit. Furthermore, a Phylip analysis was performed using the neighbor-joining method (NEIGHBOR) in PHYLIP phylogeny package (version 3.67), with the inclusion of 100 bootstraps (Felsentein, 1989), to generate a phenogram of amino acid sequence similarities to confirm the identities of the deduced amino acid sequences of Nkaα isoforms from the gills of P. schlosseri. The phylogenetic tree were generated with CONSENSE using 50% majority rule and plotted using the TREEVIEW program version 1.6.6. (http://taxonomy.zoology.gla.ac.uk/rod/treeview.html) (Page, 1996).
Amino acid sequences of Nkaα from various animals were obtained from Genbank with the following accession numbers: A. testudineus Nkaα1a (AFK29492.1), A. testudineus Nkaα1b (AFK29493.1), A. testudineus Nkaα1c (AFK29494.1), A. anguilla Nkaα1 (CAA53714.1), Danio rerio Nkaα1 (NP_571761.1), D. rerio Nkaα1a.2 (NP_571762.1), D. rerio Nkaα1a.3 (NP_571763.1), D. rerio Nkaα1a.4 (NP_571764.1), D. rerio Nkaα1a.5, D. rerio Nkaα1b (NP_571765.1), D. rerio Nkaα2 (NP_571758.1), Salmo salar Nkaα1 (ACN10460.1), O. mykiss Nkaα1a (NP_001117933.1), O. mykiss Nkaα1b (NP_001117932.1), O. mykiss Nkaα1c (NP_001117931.1), Oncorhynchus masou Nkaα1a (BAJ13363.1), O. masou Nkaα1b (BAJ13362.1), O. mykiss Nkaα2 (NP_001117930.1), O. mykiss Nkaα3 (NP_001118102.1), F. heteroclitus Nkaα1 (AAL18002.1), F. heteroclitus Nkaα2 (AAL18003.1), Sarotherodon melanotheron Nkaα1 (ADB03120.1), O. mossambicus Nkaα1 (AAD11455.2), O. mossambicus Nkaα3 (AAF75108.1), Chanos chanos Nkaα1 (ABF58911.1), Carassius auratus Nkaα3 (BAB60722.1), Trematomus bernacchii Nkaα3 (AAY30258.1), and Saccoglossus kowalevskii Nkaα1 (XP_002737354.1; as the outgroup).
Quantitative Real-Time PCR (qPCR)
There are two types of quantification methods for qPCR (Wong and Medrano, 2005). In absolute quantification, the precise amount of the template used for the curve is known with expression levels being expressed in absolute numbers of copies. In relative quantification, the template of interest is known to be present in the sample, but its absolute amount is uncertain. However, fold change does not allow the interpretation of which isoform being the predominant one being expressed in a certain condition. Since it is essential to compare the mRNA expression of nkaα1 and nkaα3 in the gills of P. schlosseri, the method of absolute quantification with reference to a standard curve was adopted in this study.
RNA (2 μg) from gill samples of P. schlosseri were extracted as mentioned above and reverse-transcribed using random hexamer primers with RevertAid™ first strand cDNA synthesis kit (Thermo Fisher Scientific Inc.). qPCR was performed in triplicates using a StepOnePlus™ Real-Time PCR System (Applied Biosystems). The mRNA expression of the two nka α-subunits in the gills of P. schlosseri was determined using specific qPCR primers (Table 1).
In order to determine the absolute quantity of the each of the nka α-subunit transcripts in a qPCR reaction, efforts were made to produce a pure amplicon (standard) of a defined region of nka α-subunits cDNA from the gills of P. schlosseri following the method of Gerwick et al. (2007). PCR was performed with the qPCR primers (Table 1) and cDNA as a template in a final volume of 25 μl with the following cycling conditions: initial denaturation 95°C for 3 min, followed by 35 cycles of 95°C for 30 s, 60°C for 30 s and 72°C for 30 s and 1 cycle of final extension of 72°C for 10 min. The PCR product was separated in a 2% agarose gel then excised and purified using Promega Wizard SV gel and PCR cleanup system. The nka α-subunit nucleotide fragment in the purified product was cloned using pGEM-T Easy vector. The presence of the insert in the recombinant clones was confirmed by sequencing. The cloned circular plasmid was quantified using a spectrophotometer.
The standard cDNA (template) was serially diluted (from 106 to 102 specific copies per 2 μl). The PCR reactions contained 5 μl of 2× Fast SYBR® Green Master Mix (Applied Biosystems), 0.3 μmol l−1 of forward and reverse primers each (Table 1) and 1 ng of sample cDNA or various quantities of standard in a total volume of 10 μl. Cycling conditions were 95°C for 20 s (1 cycle), followed by 40 cycles of 95°C for 3 s, and 60°C for 30 s. Data (Ct values) were collected at each elongation step. A melt curve analysis was performed after each run by increasing the temperature from 60 to 95°C in 0.3°C increments to confirm the presence of a single product only. The PCR products obtained were also separated in a 2% agarose gel to verify the presence of a single band. A standard curve was obtained from plotting threshold cycle (Ct) on the Y-axis and the natural log of concentration (copies μl−1) on the X-axis. The Ct slope, PCR efficiency, Y-intercept and correlation coefficient (r2) were calculated using the default setting of StepOne™ Software v2.1. Diluted standards were stored at −20°C. The PCR amplification efficiencies for the nkaα1 and nkaα3 were 97.8 and 93.7%, respectively. The quantity of transcript in a sample was determined from the linear regression line derived from the standard curve and expressed as copy number per ng cDNA (Gerwick et al., 2007).
The specificity of each pair of qPCR primers was verified by PCR using two different plasmid clones containing fragments of nkaα1 and nkaα2 as templates. The identities of these plasmid clones had been verified through cloning and sequencing previously (see section above). The specificity of each pair of primers was demonstrated by the presence of a single band using the plasmid clones of the targeted nkaα isoform as the template and the absence of detectable band using the plasmid clones of the other isoform. Furthermore, for each pair of primers, the Ct value obtained using plasmid clones of the targeted nkaα fell between 16 and 20, but no detectable Ct values (i.e., undetermined) were obtained using the other plasmid clones.
SDS-Page and Western Blotting
The gill filaments were homogenized three times in five volumes (w/v) of ice cold buffer containing 50 mmol l−1 Tris HCl, (pH 7.4), 1 mmol l−1 EDTA, 150 mmol l−1 NaCl, 1 mmol l−1 NaF, 1 mmol l−1 Na3VO4, 1% NP-40, 1% sodium deoxycholate, 1 mmol l−1 PMSF, and 1× HALT protease inhibitor cocktail (Thermo Fisher Scientific Inc.) at 24,000 rpm for 20 s each with 10 s intervals using the Polytron PT 1300D homogenizer (Kinematica AG, Lucerne, Switzerland). The homogenate was centrifuged at 10,000 × g for 20 min at 4°C. The protein concentration in the supernatant obtained was determined according to the method of Bradford (1976) and adjusted to 2 μg μl−1 with Laemmli buffer (Laemmli, 1970). Samples were heated at 70°C for 15 min, and then kept at −80°C until analysis.
Proteins were separated by SDS-PAGE (8% acrylamide for resolving gel, 4% acrylamide for stacking gel) under conditions as described by Laemmli (1970) using a vertical mini-slab apparatus (Bio-Rad Laboratories, Hercules, CA, USA). Proteins were then electrophoretically transferred onto PVDF membranes using a transfer apparatus (Bio-Rad Laboratories). After transfer, membranes were blocked with 10% skim milk in TTBS (0.05% Tween 20 in Tris-buffered saline: 20 mmol l−1 Tris-HCl; 500 mmol l−1 NaCl, pH 7.6) for 1 h before being incubated overnight at 4°C with NKA antibody (1:800 dilution) or pan-actin antibody (1:5000 dilution). The NKA antibody, α5, was developed by Douglas M. Farmbrough (Johns Hopkins University, MD, USA) under the auspices of NICHD and maintained by The University of Iowa, Department of Biological Sciences, Iowa City, IA52242, USA. α5 is known to react comprehensively with Nka α-subunit isoforms in fish (Wilson et al., 2007; Ip et al., 2012a). Both α5 and pan-actin antibodies were diluted in 1% BSA in TTBS. The membranes were then incubated in alkaline phosphatase-conjugated secondary antibodies (anti-mouse or anti-goat; 1:10,000 dilutions) for 1 h, rinsed and then incubated for 5 min in a solution of 5-bromo-4-chloro-3-indolyl phosphate p-toluidine salt and nitro-blue tetrazolium chloride (Invitrogen, Carlsbad, CA, USA) for color development. The blots were scanned using CanonScan 4400F flatbed scanner in TIFF format at 300 dpi resolution. Densitometric quantification of band intensities were performed using ImageJ (version 1.40, NIH), calibrated with a calibrated 37 step reflection scanner scale (1″ × 8″; Stouffer #R3705-1C). Results were presented as relative protein abundance of Nka normalized with actin.
Statistical Analysis
Results are presented as means ± standard errors of the mean (S.E.M.). Results presented in Figures 1–3, 10–12 were evaluated by Student t-test while those presented in Figures 7–9 were evaluated by One-Way analysis of variance (ANOVA), followed by multiple comparisons of means by the Tukey test, because they satisfied the criteria (i.e., normal distribution and equal variances) for parametric analyses. For data presented in Table 2, arcsin transformation was applied to achieve normal distribution, before statistical analysis by ANOVA and the Tukey test. Differences with P < 0.05 were regarded as statistically significant.
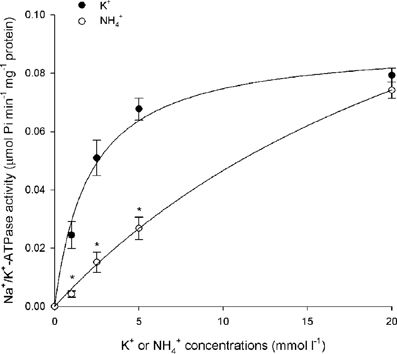
Figure 1. Effects of varying K+ or NH+4 concentrations on branchial Na+/K+-ATPase (Nka) activity. Specific activity (μmol Pi min−1 mg−1 protein) of Nka from the gills of Periophthalmodon schlosseri pre- acclimated to slightly brackish water (SBW; salinity 3) for 2 weeks (control) with varying concentrations of K+ or NH+4. Results represent mean ± s.e.m. (N = 4). *Significant difference from the corresponding K+-induced specific activity (P < 0.05).
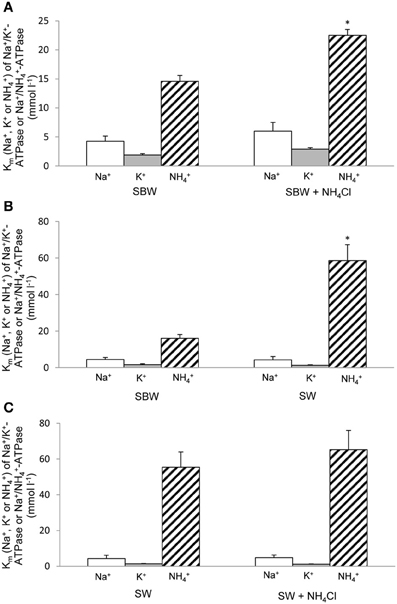
Figure 2. Km (for Na+, K+, or NH+4) of Na+/K+(NH+4)-ATPase under various experimental conditions. Effects of 6-days exposure to (A) slightly brackish water (SBW; salinity 3) containing 75 mmol l−1 NH4Cl, (B) seawater (SW; salinity 30), or (C) SW containing 50 mmol l−1 NH4Cl on Km (for Na+, K+, or NH+4) of Na+/K+(NH+4)-ATPase (mmol l−1) from gills of Periophthalmodon schlosseri which had been kept in SBW for 2 weeks. Results represent mean ± s.e.m. (N = 4). *Significant difference from the Km for NH+4 of Na+/K+(NH+4)-ATPase of the corresponding control (P < 0.05).
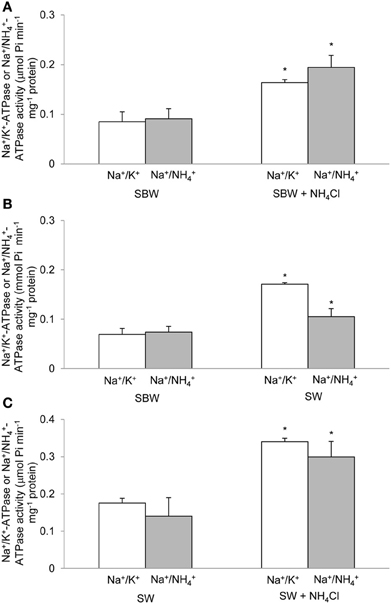
Figure 3. Specific activity of Na+/K+(NH+4)-ATPase under various experimental conditions. Effects of 6-days exposure to (A) slightly brackish water (SBW; salinity 3) containing 75 mmol l−1 NH4Cl, (B) seawater (SW; salinity 30), or (C) SW containing 50 mmol l−1 NH4Cl on the specific activity (μmol Pi min−1 mg−1 protein) of Na+/K+(NH+4)-ATPase from gills of Periophthalmodon schlosseri which had been kept in SBW for 2 weeks. Results represent mean ± s.e.m. (N = 4). *Significant difference from the corresponding control (P < 0.05).
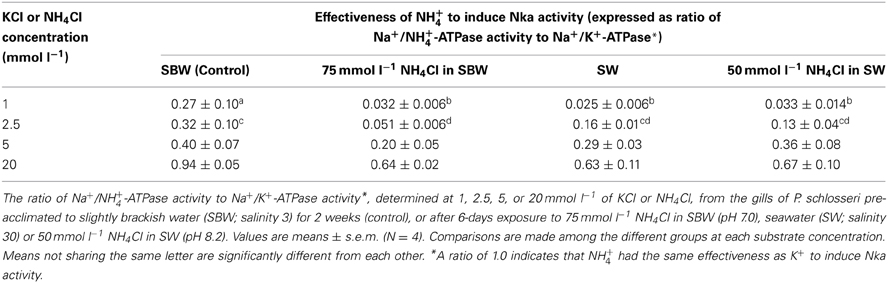
Table 2. Effectiveness of NH+4 to activate Na+/K+-ATPase (Nka) from the gills of Periophthalmodon schlosseri.
Results
Kinetic Properties of Branchial Nka after Exposure to Various Conditions
Results obtained in this study revealed that NH+4 could not effectively substitute for K+, especially at low substrate concentrations, to induce Nka activity from the gills of P. schlosseri pre-acclimated to SBW for 2 weeks (Figure 1; Table 2). For branchial Nka from these control fish in SBW, the Km for K+ (1.6 mmol l−1) was substantially lower than the Km for NH+4 (15 mmol l−1; Figure 2A), indicating that it had much greater affinity to K+ than NH+4. After 6-days exposure to 75 mmol l−1 NH4Cl in SBW, the Km of branchial Nka for K+ remained relatively unchanged but the Km for NH+4 increased significantly to 23 mmol l−1 (Figure 2A). After P. schlosseri was exposed to SW for 6 days, the Km of branchial Nka for K+ also remained unchanged but the Km for NH+4 increased significantly to 56 mmol l−1 (Figure 2B). The Km for K+ and Km for NH+4 of Nka from the gills of P. schlosseri exposed to 50 mmol l−1 NH4Cl in SW were comparable to those of fish exposed to SW (Figure 2C).
In general, the effectiveness of NH+4 to replace K+ to activate Nka (expressed as the ratio of Na+/NH+4-ATPase activity to Na+/K+-ATPase activity) from the gills of P. schlosseri was the highest at saturating substrate concentrations (0.63–0.94 at 20 mmol l−1; Table 2), and it decreased with decreasing substrate concentrations (0.03–0.27 at 1 mmol l−1; Table 2). At high concentrations (5 and 20 mmol l−1) of K+ or NH+4, exposure to 75 mmol l−1 NH4Cl in SBW, SW or 50 mmol l−1 NH4Cl in SW for 6 days had no significant effects on the effectiveness of NH+4 to substitute for K+ as compared to the SBW control (Table 2). However, the effectiveness of NH+4 to substitute for K+ to activate Nka at 1 mmol l−1 of K+ or NH+4 decreased significantly from 0.27 for the SBW control to 0.025–0.033 for fish exposed to 75 mmol l−1 NH4Cl in SBW, SW or 50 mmol l−1 NH4Cl in SW for 6 days (Table 2). Furthermore, at 2.5 mmol l−1 of K+/NH+4, the effectiveness of NH+4 to substitute for K+ to activate Nka from gills of fish exposed to 75 mmol l−1 NH4Cl in SBW for 6 days (0.051) was significantly lower than that of the SBW control (0.32; Table 2).
There were significant increases in activities (Vsat, which was determined at saturating substrate concentrations and hence close to the theoretical Vmax) of Na+/K+-ATPase and Na+/NH+4-ATPase from the gills of P. schlosseri after 6-days exposure to 75 mmol l−1 NH4Cl in SBW (Figure 3A) or SW (Figure 3B) as compared to the SBW control. The Vsat of branchial Na+/K+-ATPase and Na+/NH+4-ATPase from fish exposed to 50 mmol l−1 NH4Cl in SW for 6 days were significantly higher than those of fish exposed to SW only (Figure 3C).
Nucleotide Sequences of Branchial nka α Isoforms and their Deduced Amino Acid Sequences
Two full cDNA sequences of nka α-subunits, nkaα1 (GenBank: KF410828) and nkaα3 (GenBank: KF410829), were cloned from the gills of P. schlosseri. As previous studies demonstrated that the set of primers used to clone and sequence nkaα in this study could effectively “fish out” 3 nkaα1 isoforms, 1 nkaα2 isoform and 2 nkaα3 isoforms from the gills (Ip et al., 2012a, 2013) and brains (Chen et al., 2013; Hiong et al., 2014) of 4 other fish species, it is highly probable that the gills of P. schlosseri expressed only one isoform each of nkaα1 and nkaα3.
The coding sequence of nkaα1 from the gills of P. schlosseri contained 3075 bp, which encoded for 1024 amino acids with a calculated molecular mass of 112.5 kDa (Figure 4). In comparison, the cDNA coding sequence of nkaα3 was slightly shorter and contained 3030 bp, encoding 1009 amino acids with a calculated molecular mass of 111.4 kDa (Figure 4). A hydropathy analysis of Nkaα1 revealed that both nkaα1 and nkaα3 comprised 10 transmembrane domains (Figure 4). Nkaα1 and Nkaα3 from gills of P. schlosseri have large areas of conserved regions of Nka/NKA, which include the threonine-glycine-glutamic acid-serine (TGES) motif, the proline-glutamic acid-glycine-leucine (PEGL) motif, the aspartic acid-lysine-theronine-glycine-threonine (DKTGT) motif containing the phosphorylation site, and the glycine-aspartic acid-glycine-valine-asparagine-aspartic acid-serine-proline (GDGVNDSP) motif (Figure 4).
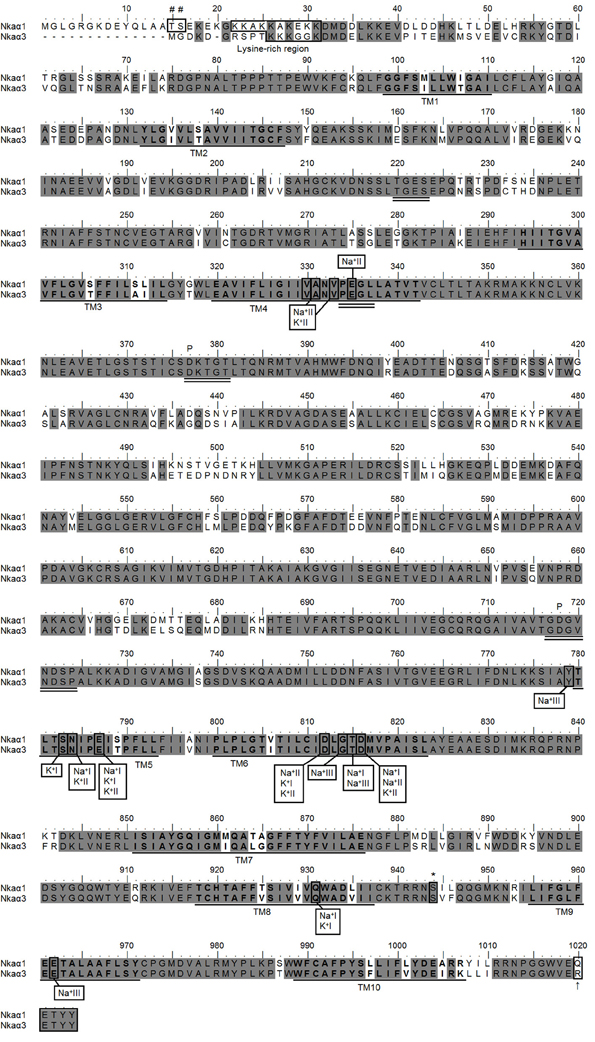
Figure 4. Molecular characterization of Na+/K+-ATPase (Nka) α1 and Nkaα3. Amino acid sequence alignment of Nkaα1 and Nkaα3 from the gills of Periophthalmodon schlosseri. Identical amino acid residues are indicated by shaded dark gray residues. The 10 predicted transmembrane regions (TM1-TM10) are underlined and in bold. Vertical boxes represent coordinating residues for Na+ or K+ binding. The conserved regions containing the TGES, PEGL, DKTGT, and GDGVNDSP sequence motifs are double underlined and the phosphorylation sites are indicated by a “P.” The asterisk and hash marks denote the amino acid residues phosphorylated by protein kinase A and protein kinase C, respectively. The lysine-rich region is indicated with a box. The KETYY motif is indicated with a box and an arrow indicating the amino acid residue that replaced arginine. The transmembrane domains were predicted using MEMSAT3 and MEMSAT-SVM provided by PSIPRED protein structure prediction server.
A comparison of Nkaα1 and Nkaα3 of P. schlosseri with Nka α-subunits of teleosts and NKA α-subunits from other animals revealed that they shared the highest amino acid sequence identity with teleost Nkaα1 (95.4–90.7%) and Nkaα3 (94.5–92.1%), respectively. Indeed, a phenogram of amino acid sequence similarities generated through phylogenetic analysis confirmed that the two Nkaα isoforms cloned from the gills of P. schlosseri were Nkaα1 and Nkaα3 (Figure 5).
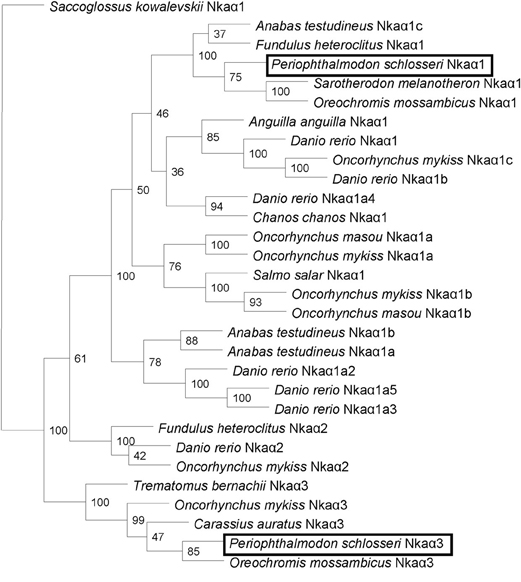
Figure 5. Phenogramic analysis of Na+/K+-ATPase (Nka) α1 and Nkaα3. A phenogram generated through phylogenetic analysis to illustrate the relationship between Nkaα1 and Nkaα3 from gills of Periophthalmodon schlosseri and Nkaα1, Nkaα2 and Nkaα3 of selected teleost species. Numbers presented at each branch point represent bootstrap values from 100 replicates. Saccoglossus kowalevskii Nka is used as an outgroup.
The percentage similarities between the deduced amino acid sequence of Nkaα1 from gills of P. schlosseri and Nkaα1a [JN180940], Nkaα1b [JN180941], and Nkaα1c [JN180942] from gills of A. testudineus were 81.1, 87.7, and 93.5%, respectively; for Nkaα3 from gills of P. schlosseri, the respective similarities were 75.5, 78.5, and 85.0%. Hence, both Nkaα1 and Nka3 of P. schlosseri had the greatest similarity with Nkaα1c of A. testudineus. A detailed analysis of the amino acid residues constituting the K+ binding sites of Nkaα1 and Nkaα3 from the gills of P. schlosseri confirmed that they are identical to those of Nkaα1c, but different from those of Nkaα1a (freshwater-isoform) and Nkaα1b (seawater-isoform), from the gills of A. testudineus (Figure 6).
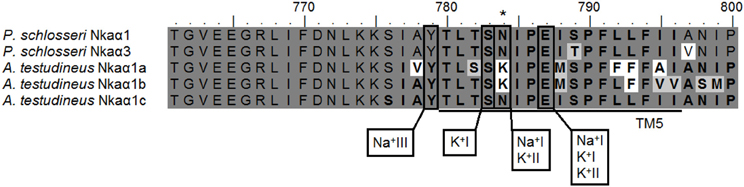
Figure 6. Analysis of Na+/K+ binding site of Na+/K+-ATPase (Nka) α1 and Nkaα3. A multiple amino acid sequence alignment of a region of Nkaα1 and Nkaα3 from gills of Periophthalmodon schlosseri, with Nkaα1a (GenBank: JN180940), Nkaα1b (GenBank: JN180941), and Nkaα1c (GenBank: JN180942) from the gills of Anabas testudineus. Identical amino acid residues are indicated by shaded black residues and similar amino acids (threshold value 60%) are indicated by shaded gray residues. Vertical boxes represent coordinating residues for Na+ or K+ binding. Asterisks indicate the amino acid residue that is similar to Nkaα1c but different from Nkaα1a and Nkaα1b.
mRNA Expression of Branchial nkaα1 and nkaα3 Exposed to Various Conditions
Six days of exposure of P. schlosseri to 75 mmol l−1 NH4Cl in SBW had no significant effects on the mRNA expression of nkaα1 in the gills; however, there was a transient but significant increase in the branchial mRNA expression of nkaα3 on day 2 (Figure 7). Similarly, 6-days exposure to SW had no significant effects on the mRNA expression of nkaα1 in the gills of P. schlosseri, but there was a significant increase in the branchial mRNA expression of nkaα3 on day 6 (Figure 8). However, upon exposure to 50 mmol l−1 NH4Cl in SW, there were significant increases in the mRNA expression of nkaα1 and nkaα3 (Figure 9) in the gills of P. schlosseri on day 2 and 6, respectively.
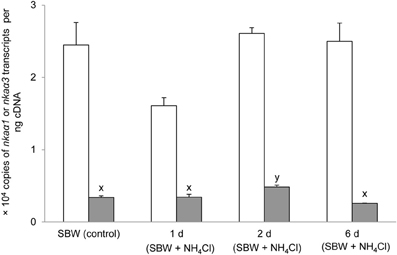
Figure 7. Effects of exposure to ammonia in slightly brackish water on Na+/K+-ATPase (nka) α1 and nkaα3 mRNA expression. Absolute quantification (copies of transcript per ng cDNA) of nkaα1 () and nkaα3 (
) in gills of Periophthalmodon schlosseri kept in slightly brackish water (SBW; salinity 3) for 2 weeks (control), or after 1, 2, or 6 days of transfer from SBW to SBW containing 75 mmol l−1 NH4Cl. Results represent mean + s.e.m. (N = 4). Means not sharing the same letter are significantly different (P < 0.05).
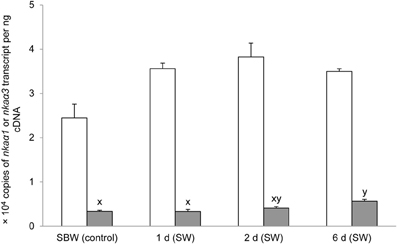
Figure 8. Effects of exposure to seawater on Na+/K+-ATPase (nka) α1 and nkaα3 mRNA expression. Absolute quantification (copies of transcript per ng cDNA) of nkaα1 () and nkaα3 (
) in gills of Periophthalmodon schlosseri kept in slightly brackish water (SBW; salinity 3) for 2 weeks (control) or after 1, 2, or 6 days of transfer from SBW to seawater (SW; salinity 30). Results represent mean + s.e.m. (N = 4). Means not sharing the same letter are significantly different (P < 0.05).
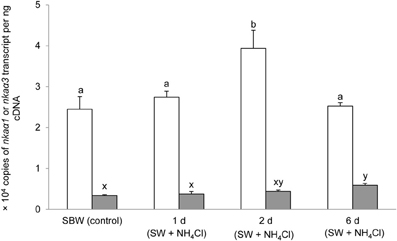
Figure 9. Effects of exposure to ammonia in seawater on Na+/K+-ATPase (nka) α1 and nkaα3 mRNA expression. Absolute quantification (copies of transcript per ng cDNA) of nkaα1 () and nkaα3 (
) in gills of Periophthalmodon schlosseri kept in slightly brackish water (SBW; salinity 3) for 2 weeks (control) or after 1, 2, or 6 days of transfer from SBW to seawater (SW; salinity 30) containing 50 mmol l−1 NH4Cl. Results represent mean + s.e.m. (N = 4). Means not sharing the same letter are significantly different (P < 0.05).
Protein Abundance of Branchial nkaα Exposed to Various Conditions
Based on Western blotting results using the α5 anti-NKA antibody, which reacted comprehensively with fish Nka α-subunit isoforms, the protein abundance of Nkaα remained unchanged in the gills of P. schlosseri after 6-days exposure to 75 mmol l−1 NH4Cl in SBW (Figure 10) or SW (Figure 11) as compared with that of the control pre-acclimated to SBW for 2 weeks. By contrast, 6-days exposure to 50 mmol l−1 NH4Cl in SW led to a slight but significant increase in the protein abundance of Nkaα in the gills of P. schlosseri (Figure 12).
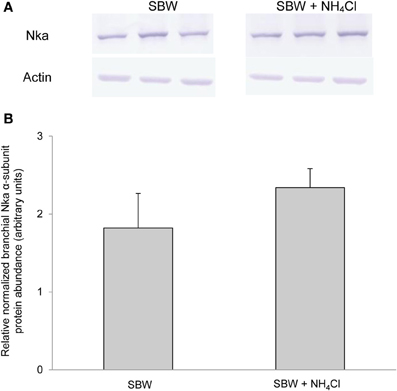
Figure 10. Effects of exposure to ammonia in slightly brackish water on Na+/K+-ATPase (Nka) protein abundance. Protein abundance of Nka based on the α5 anti-NKA antibody, which is known to react with all Nka/NKA α-isoforms, in gills of Periophthalmodon schlosseri kept in slightly brackish water (SBW; salinity 3) for 2 weeks (control) or after 6 days of transfer from SBW to SBW containing 75 mmol l−1 NH4Cl. (A) The immunoblots of Nka and actin. (B) The intensity of the Nka band was normalized with respect to that of actin. Results represent mean + s.e.m. (N = 3).
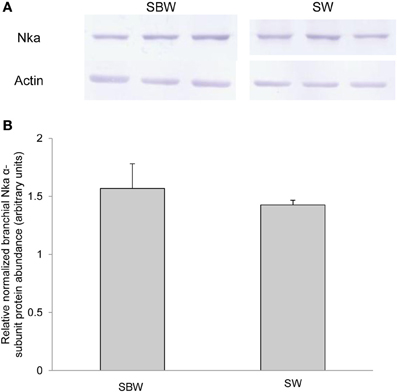
Figure 11. Effects of exposure to seawater on Na+/K+-ATPase (Nka) protein abundance. Protein abundance of Nka based on the α5 anti-NKA antibody which is known to react with all Nka/NKA α-isoforms of gills of Periophthalmodon schlosseri kept in slightly brackish water (SBW; salinity 3) for 2 weeks (control) or after 6 days of transfer from SBW to seawater (SW; salinity 30). (A) The immunoblots of Nka and actin. (B) The intensity of the Nka band was normalized with respect to that of actin. Results represent mean + s.e.m. (N = 3).
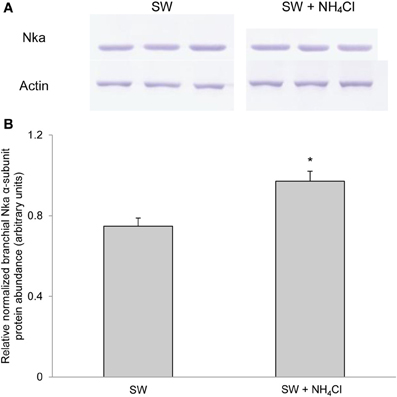
Figure 12. Effects of exposure to ammonia in seawater on Na+/K+-ATPase (Nka) protein abundance. Protein abundance of Nka based on the α5 anti-NKA antibody which is known to react with all Nka/NKA α-isoforms of gills of Periophthalmodon schlosseri after 6 days of transfer from slightly brackish water (SBW; salinity 3) to seawater (SW; salinity 30; control) or after 6 days of transfer from SBW to SW containing 50 mmol l−1 NH4Cl. (A) The immunoblots of Nka and actin. (B) The intensity of the Nka band was normalized with respect to that of actin. Results represent mean + s.e.m. (N = 3). *Significantly different from the control (P < 0.05).
Discussion
Km for NH+4 is Substantially Greater than Km for K+ Under All Experimental Conditions
Periophthalmodon schlosseri could survive abrupt transfer between SBW and SBW containing 75 mmol l−1 NH4Cl and between SBW and SW with or without 50 mmol l−1 NH4Cl without mortality. Exposure of P. schlosseri to SW or to ammonia in SBW or SW resulted in significant increases in the Vsat of branchial Nka, with Na+ and K+/NH+4 as substrates, indicating that branchial Nka was essential for salt excretion in a hyperosmotic medium and active NH+4 excretion in a medium containing high concentrations of ammonia.
For branchial Nka of P. schlosseri pre-acclimated to SBW for 2 weeks, the Km for K+ (1.6 mmol l−1) was substantially lower than the Km for NH+4 (15 mmol l−1), indicating that the K+ binding site had high affinity for K+ and low affinity for NH+4. Taking the plasma K+ and NH+4 concentrations as 10 mmol l−1 and 1 mmol l−1, respectively, it would mean that branchial Nka would be saturated with K+, and therefore it is highly improbable that branchial Nka would transport NH+4 in replacement of K+. More importantly, after 6-days exposure to 75 mmol l−1 NH4Cl in SBW, the Km for NH+4 increased significantly to 23 mmol l−1 with the Km for K+ remained relatively unchanged. These results indicate that branchial Nka could even better differentiate NH+4 from K+ when P. schlosseri was challenged with environmental ammonia. Hence, it can be concluded that the main function of Nka in active NH+4 excretion is to maintain intracellular Na+ and K+ homeostasis, and NH+4 is not directly transported into ionocytes through the basolateral Nka during active NH+4 excretion as proposed previously for P. schlosseri (Randall et al., 1999; Weihrauch et al., 2009; Hwang et al., 2011).
For P. schlosseri exposed to SW, the Km of the branchial Nka for K+ remained unchanged while the Km for NH+4 increased significantly to 56 mmol l−1. Thus, SW exposure apparently led to increases in expression and/or activity of a Nkaα isoform which had even lower affinity for NH+4 as compared with the control fish. These results have important bearing on the behavior and physiology of P. schlosseri, which builds burrows in mudflats. During high tides, P. schlosseri seeks refuge into mud burrows, which may contain water with high salt and ammonia concentrations. During the breeding season, eggs are laid inside the burrows, and ammonia produced during embryonic development could further increase the ammonia concentration therein. Therefore, it would be advantages for P. schlosseri to readily increase the selectivity of its branchial Nka for K+ over NH+4 during SW exposure. Overall, these results indicate that all the Nkaα isoforms expressed in the gills of P. schlosseri in SBW, SW, or ammonia in SBW/SW would have K+ binding sites that could effectively differentiate K+ from NH+4. Thus, we proceeded to clone nkaα isoforms from the gills of P. schlosseri in order to characterize the K+ binding sites of their deduced amino acid sequences.
Molecular Characterization of nkaα Isoforms
Two nkaα isoforms, nkaα1 and nkaα3, were cloned from the gills of P. schlosseri. Similarly, Feng et al. (2002) reported the expression of two nka isoforms, nkaα1 and nkaα3 in the gills of O. mossambicus. Using isoform specific anti-Nkaα antibodies, Lee et al. (1998) demonstrated that Nkaα1 and Nkaα3 were present in branchial ionocytes of O. mossambicus, and showed that their expressions were responsive to salinity changes.
Three Na+ and two K+ binding sites are known to be present in the NKA α-subunit (Ogawa and Toyoshima, 2002). The coordinating residues present in the binding sites are arranged within the transmembrane domains such that the release of one type of cation coordinates with the binding of the other. Based on the homology modeling of human NKAα (Ogawa and Toyoshima, 2002), these coordinating residues were conserved in Nkaα1 and Nkaα3 from the gills of P. schlosseri.
Morth et al. (2007) reported a 96% reduction in Na+ affinity when five amino acid residues (KETYY) were deleted from the C-terminus of NKA. Although the KETYY motif was present in both Nka α-subunit isoforms of P. schlosseri, the lysine residue was replaced by glutamine and arginine in Nkaα1 and Nkaα3, respectively. This could indicate that the Na+ affinity of Nkaα1 could be different from that of Nkaα3. Mutations studies have shown that Asparagine-786 is critical for both Na+ and K+ binding (Pedersen et al. 1998). For A. testudineus, there was a replacement of asparagine by lysine in position 786 of Nkaα1a (freshwater-isoform) and Nkaα1b (seawater-isoform) (Ip et al., 2012a). However, similar to Nkaα1c (ammonia-isoform) of A. testudineus (Ip et al., 2012a), no replacement of the equivalent Asparagine-784 was found in both Nkaα1 and Nkaα3 of P. schlosseri.
The amino acid residues constituting the K+ binding sites of Nkaα1 and Nkaα3 from the gills of P. schlosseri were identical to those of Nkaα1c, but different from those of Nkaα1a and Nkaα1b, from the gills of A. testudineus (Ip et al., 2012a). Ip et al. (2012a) demonstrated that exposure of A. testudineus to ammonia led to significant increases in the mRNA expression of nkaα1c, the overall Nkaα protein abundance and the activity of Nka in its gills. Furthermore, there were increases in the Km of Nka for K+ and NH+4, with the increase in Km for NH+4 being much greater than that for K+, indicating a decrease in the effectiveness of NH+4 to replace K+ to activate branchial Nka in A. testudineus exposed to ammonia. Thus, Ip et al. (2012a) concluded that the up-regulation of nkaα1c expression served to maintain intracellular Na+ and K+ homeostasis by removing excess Na+ from, and transporting K+ in preference to NH+4 into, ionocytes. The similarity in the K+ binding sites between Nkaα1/Nkaα3 of P. schlosseri and Nkaα1c of A. testudineus corroborate the fact that the overall Nka activity from the gills of P. schlosseri exhibited high substrate specificity for K+, that is, low Km for K+ and high Km for NH+4.
Incidentally, basolateral Nka is also known to be involved in branchial ammonia excretion in aquatic crustaceans (Towle and Holleland, 1987; Towle et al., 2001). Masui et al. (2002, 2009) demonstrated that the branchial Nka activity from the marine blue crab, Callinectes danae, was synergistically stimulated by NH+4 and K+. Upon the addition of NH+4 to the assay medium containing an optimal concentration of K+, the activity of Nka increased by ~90%. It was speculated that K+ and NH+4 were bound to different sites of Nka in C. danae (Masui et al., 2002, 2009). Furriel et al. (2004) reported a similar phenomenon for the branchial Nka from the freshwater shrimp, Macrobrachium olfersii. They proposed that at high NH+4 concentrations, a new binding site for NH+4 was exposed in the Nka from M. olfersii. This new binding site modulated the Nka activity independently of K+ after binding to NH+4 (Furriel et al., 2004). However, to date, no molecular or structural information is available on the substrate binding sites of Nka from the gills of M. olfersii. Notably, we were unable to demonstrate a synergistic effect of K+ and NH+4 in inducing the activity of Nka from the gills of P. schlosseri (Chew and Ip, unpublished results). In addition, molecular characterization of Nkaα1 and Nkaα3 from the gills of P. schlosseri exposed to ammonia in SBW revealed, as usual, the presence of only two binding sites for K+ and three binding sites for Na+.
It is probable that Nka was regulated by phosphorylation/dephosphorylation in the gills of P. schlosseri exposed to various environmental conditions. Both cAMP-dependent protein kinase A (PKA) and protein kinase C (PKC) are known to be involved in the phosphorylation of the NKA α-subunit (Aperia et al., 1994). One possible site of cAMP-dependent PKA phosphorylation is the Serine-944 of the NKA α-subunit from kidneys of the rat and the giant toad, Bufo marinus (Beguin et al., 1994; Feschenko and Sweadner, 1994). This site was conserved in Nkaα1 and Nkaα3 of P. schlosseri. Beguin et al. (1994) identified Threonine-10 and Serine-11 as cAMP-dependent PKC phosphorylation sites in the NKA of B. marinus by site-directed mutagenesis. For P. schlosseri, these two PKC phosphorylation sites corresponded to Threonine-15 and Serine-16 in Nkaα1, but were absent from Nkaα3. Feschenko and Sweadner (1995) identified two cAMP-dependent PKC phosphorylation sites, Serine-11 and Serine-18, with different phosphorylatability in rat kidney NKAα1. For P. schlosseri, only Serine-16 was found in Nkaα1, but both serine residues were absent from Nkaα3. These differences in phosphorylation sites between Nkaα1 and Nkaα3 might have important bearing on the interpretation of the changes in mRNA expression of nkaα1 and nkaα3 and the protein abundance of Nkaα in the gills of P. schlosseri in response to various experimental conditions.
Exposure to Ammonia in SBW
Exposure of P. schlosseri to 75 mmol l−1 NH4Cl in SBW led to increases in Nka activity and mRNA expression of nkaα3, but not the overall Nkaα protein abundance in its gills. There was a significant increase (~2-fold) in the Vsat of branchial Na+/K+-ATPase and Na+/NH+4-ATPase. This corroborates the proposition that there could be an increase in the entry of Na+, NH+4, and 2Cl− into the ionocyte through the basolateral Nkcc1 during active ammonia excretion, and an increase in Nka activity was needed to pump the excess Na+ back to the blood. As such, ouabain would predictably have an inhibitory effect on active NH+4 excretion, as reported by Randall et al. (1999), not because of the direct involvement of Nka in NH+4 transport, but because a decrease in Nka activity would necessarily result in a reduction in the efficiency of Nkcc1 to transport Na+ together with K+/NH+4 and 2Cl− down the electrochemical gradient of Na+ generated by Nka.
Increases in Nka activity in fish gills can be due to an up-regulation of nkaα mRNA expression leading to an increase in Nkaα protein abundance (Singer et al., 2002; Tipsmark et al., 2002; Scott et al., 2004; Lin et al., 2006) and/or a modulation of the enzyme's hydrolytic rate (Crombie et al., 1996; Bystriansky et al., 2007). In addition, Nka activity can be rapidly modulated by several protein kinases that directly phosphorylate/dephosphorylate Nka and/or proteins involved in the trafficking of Nka between plasma membrane and intracellular endosomal pools (Blanco and Mercer, 1998). For instance, PKA (Tipsmark and Madsen, 2001) and PKC (Crombie et al., 1996) have been shown to alter branchial Nka activities in the brown trout (Salmo trutta) and the Atlantic cod (Gadus morhua), respectively. In the case of P. schlosseri exposed to ammonia in SBW, there was no significant change in the comprehensive Nkaα protein abundance after 6-days ammonia exposure despite a significant increase in the mRNA expression of nkaα3 in the gills on day 2 of exposure to ammonia. Based on the quantities of nkaα1 (2.4 × 104 copies) and nkaα3 (0.35 × 104 copies) transcripts expressed in the gills of P. schlosseri pre-acclimated to SBW, it was apparent that nkaα1 was the predominate isoform. Hence, it is not unexpected that the transient increase in mRNA expression of nkaα3 did not result in a significant increase in the total protein abundance of Nkaα.
Overall, these results suggest that the ammonia-induced increase in Nka activity in the gills of P. schlosseri could be attributed mainly to post-translational modification. This could be of physiological significance to P. schlosseri which is frequently found moving on land during low tide and hiding in a burrow during high tide. Amino acids serve as a major energy source for its locomotor activity on land (Ip et al., 2001b), and the catabolism of these amino acids would result in an increase in the production of ammonia which is actively excreted into fenestrae of the gills formed by intrafilamentous interlamellar fusions (Chew et al., 2007). On the other hand, P. schlosseri could be exposed to high concentrations of ammonia when it is inside the burrow, and needs to actively excrete the ammonia therein. As there are two tides daily in the tropics and each low/high tide lasts approximately 6 h, P. schlosseri must have the ability to adapt promptly to environmental changes. It would be inefficient if P. schlosseri was to modulate its branchial Nka activity predominantly through transcriptional and/or translational changes which are relatively slow processes as compared to resorting to post-translational modification.
Exposure to SW
It has been established that SW acclimation induces an increase in the Nka activity from the gills of many fish species (see Hwang and Lee, 2007 for a review). Indeed, 6-days SW acclimation led to a significant increase in the Vsat of branchial Na+/K+-ATPase and Na+/NH+4-ATPase in P. schlosseri. Since exposure of P. schlosseri to SW also resulted in a significant increase in the mRNA expression and protein abundance of nkcc1a/Nkcc1a in its gills (Chew and Ip, unpublished results), it is probable that NaCl extrusion through the gills of P. schlosseri exposed to SW is mediated by the basolateral cotransport of Na+, K+, and 2Cl− through Nkcc1a, coupled with the apical exit of Cl− through the apical cystic fibrosis transmembrane conductance regulator (Cftr) and the paracellular extrusion of Na+ (see Hwang and Lee, 2007; Evans, 2008 for reviews). Being a euryhaline brackish water fish, how P. schlosseri regulate the paracellular movement of Na+ instantaneously after abrupt transfer between SW and SBW is unclear at present. Although the mRNA expression of nkaα3 increased significantly in the gills of P. schlosseri exposed to SW for 6 days, the comprehensive Nkaα protein abundance remained unchanged. Therefore, similar to exposure to ammonia in SBW, the increase in Nka activity during SW exposure could be attributed mainly to post-translational modification.
In rainbow trout (O. mykiss), Atlantic salmon (S. salar), and Arctic char (Salvelinus alpinus), SW acclimation involves the differential regulation of nkaα1a and nkaα1b, which are freshwater- and seawater-isoforms, respectively (Richards et al., 2003; Bystriansky et al., 2006, 2007). Similarly, Ip et al. (2012a) obtained results which suggested nkaα1a and nkaα1b as the freshwater- and seawater-isoforms, respectively, in the gills of A. testudineus, which can progressively acclimate from fresh water to SW. In euryhaline freshwater fishes, changes in ionocyte types are critical to branchial osmoregulatory acclimation (Hiroi et al., 1999). For A. testudineus, the small Nkaα1a-positive ionocytes are replaced by the large Nkaα1b-positive ionocytes during a progressive acclimation from fresh water to SW, and the replacement process involves extensive apoptosis in the gills (Ching et al., 2013). By contrast, our results indicated the absence of distinct freshwater- and seawater-Nkaα isoforms in the gills of P. schlosseri. It is probable that the lack of delineation between freshwater- and seawater-Nkaα isoforms and demarcation between freshwater- and seawater-ionocytes are pre-requisites for euryhaline brackish water fishes like P. schlosseri to survive large and abrupt changes in salinity.
Exposure to Ammonia in SW
Exposure of P. schlosseri to 50 mmol l−1 NH4Cl in SW resulted in increases in Nka activity, mRNA expression of nkaα1 and nkaα3 and the overall Nkaα protein abundance in its gills. Ammonia toxicity in fishes increases in SW because of multiple reasons. SW has a pH of 8.2, at which the ratio of NH3 to NH+4 would be greater than that at pH 7.0 in SBW (or fresh water), and NH3 is the predominant species that permeate biomembranes. More importantly, fishes dehydrate in SW and therefore need to imbibe SW and excrete excess salt through the gills. Ingestion of SW containing ammonia would lead to a large influx of ammonia through the digestive tract in addition to the branchial and cutaneous epithelial surfaces, and the formation of paracellular route for the extrusion of excess Na+ through the branchial epithelium may also result in increased influx of ammonia. Hence, the ability of P. schlosseri to survive in 50 mmol l−1 NH4Cl in SW at pH 8.0 is extraordinary. Indeed, exposure of P. schlosseri to ammonia in SW led to a further increase (~2-fold) in Vsat of branchial Na+/K+-ATPase and Na+/NH+4-ATPase as compared with exposure to SW only. Notably, the Vsat of 0.35 μmol Pi min−1 mg−1 protein exhibited by the gills of P. schlosseri exposed to ammonia in SW could be the highest activity recorded for fish gills. The increase in Vmax was accompanied with significant increases in mRNA expression of nkaα1 on day 2 and nkaα3 on day 6, which together contributed to a moderate increase in the overall protein abundance of Nkaα, indicating that, once again, post-translational modification could be an important modulating factor. At present, it is uncertain whether active salt excretion and active NH+4 excretion involved different types of ionocytes in the gills of P. schlosseri exposed to ammonia in SW.
Perspective
Ip et al. (2012a,b) reported that active excretion of NH+4 through the gills of A. testudineus in fresh water involved a type of Nka-immunopositive ionocytes with apical Cftr and basolateral Nkcc distinctly smaller than those Nka-immunopositive ionocytes involved in SW acclimation. They suggested that the active excretion of NH+4 through some unknown apical NH+4 channels could be driven by the transmembrane electrical potential generated by the efflux of Cl−/HCO−3 through the apical Cftr. However, it is highly probable that the mechanism of active ammonia excretion in P. schlosseri would be different from that in A. testudineus because P. schlosseri acidifies the branchial water and the external medium during active NH+4 excretion (Ip et al., 2004b), but A. testudineus alkalinizes the branchial water instead (Ip et al., 2012b). Since P. schlosseri can survive abrupt transfer between SBW and SW with or without high concentrations of ammonia, it is probable that its gills comprise multiple types of ionocytes for hyperionic regulation in SBW, hypoosmotic hypoionic regulation in SW, and active NH+4 excretion in high concentrations of environmental ammonia. Hence, it would be rewarding in the future to examine the types of ionocytes present in the gills of P. schlosseri, with emphases on their association with a specific Nkaα isoform and other relevant ion transporters (e.g., Nkcc1, Cftr, H+-ATPase, Na+/H+ exchanger and Rhesus glycoproteins), and to elucidate how they can be functionally switched on and off instantly during abrupt changes in salinity (e.g., between SBW and SW) and abrupt exposure to environmental ammonia.
Conflict of Interest Statement
The authors declare that the research was conducted in the absence of any commercial or financial relationships that could be construed as a potential conflict of interest.
Acknowledgments
This study was supported by the Singapore Ministry of Education through grants to Shit F. Chew (RI 9/08 CSF & RI 4/12 CSF) and to Yuen K. Ip (R154-000-470-112).
References
Aperia, A., Holtbäck, U., Syrén, M. L., Svensson, L. B., Fryckstedt, J., and Greengard, (1994). Activation/deactivation of renal Na+,K+-ATPase: a final common pathway for 766 regulation of natriuresis. FASEB J. 8, 436–439.
Beguin, P., Beggah, A. T., Chibalin, A. V., Burgener-Kairuz, P., Jaisser, F., Mathews, P. M., et al. (1994). Phosphorylation of the Na,K-ATPase alpha-subunit by protein kinase A and C in vitro and in intact cells. Identification of a novel motif for PKC-mediated phosphorylation. J. Biol. Chem. 269, 24437–24445.
Blanco, G., and Mercer, R. W. (1998). Isozymes of the Na-K-ATPase: heterogeneity in structure, diversity in function. Am. J. Physiol. 275, F633–F650.
Bradford, M. M. (1976). A rapid and sensitive method for quantitation of microgram quantities of protein utilising the principle of protein-dye binding. Anal. Biochem. 72, 248–254. doi: 10.1016/0003-2697(76)90527-3
Bystriansky, J. S., Frick, N. T., Richards, J. G., Schulte, P. M., and Ballantyne, J. S. (2007). Wild Arctic Char (Salvelinus alpines) upregulate gill Na+/K+-ATPae during freshwater migration. Physiol. Biochem. Zool. 80, 270–282. doi: 10.1086/512982
Bystriansky, J. S., Richards, J. G., Schulte, P. M., and Ballantyne, J. S. (2006). Reciprocal expression of gill Na+/K+-ATPase α-subunit isoforms α1a and α1b during seawater acclimation of three salmonid fishes that vary in their salinity tolerance. J. Exp. Zool. 209, 1848–1858.
Chang, E. W. Y., Loong, A. M., Wong, W. P., Chew, S. F., Wilson, J. M., and Ip, Y. K. (2007). Changes in tissue free amino acid contents, branchial Na+/K+-ATPase activity and bimodal breathing pattern in the freshwater climbing perch, Anabas testudineus (Bloch), during seawater acclimation. J. Exp. Zool. 207A, 708–723. doi: 10.1002/jez.a.424
Chen, X. L., Wee, N. L. J. E., Hiong, K. C., Ong, J. L. Y., Chng, Y. R., Ching, B., et al. (2013). Properties and expression of Na+/K+-ATPase α-subunit isoforms in the brain of the swamp eel, Monopterus albus, which has unusually high brain ammonia tolerance. PLoS ONE 8:e84298. doi: 10.1371/journal.pone.0084298
Chew, S. F., Hong, L. N., Wilson, J. M., Randall, D. J., and Ip, Y. K. (2003). Alkaline environmental pH has no effect on the excretion of ammonia in the mudskipper Periophthalmodon schlosseri but inhibits ammonia excretion in the related species Boleophthalmus boddaerti. Physiol. Biochem. Zool. 76, 204–214. doi: 10.1086/374281
Chew, S. F., and Ip, Y. K. (2014). Excretory nitrogen metabolism and defense against ammonia toxicity in air-breathing fishes. J. Fish. Biol. 84, 603–638. doi: 10.111/jfb.12279
Chew, S. F., Sim, M. Y., Phua, Z. C., Wong, W. P., and Ip, Y. K. (2007). Active ammonia excretion in the giant mudskipper, Periophthalmodon schlosseri (Pallas), during emersion. J. Exp. Zool. 307A, 357–369. doi: 10.1002/jez.385
Chew, S. F., Wilson, J. M., Ip, Y. K., and Randall, D. J. (2006). “Nitrogen excretion and defence against ammonia toxicity,” in The Physiology of Tropical Fishes, Vol. 23, eds V. Almedia-Val, D. J. Randall, and A. Val (New York, NY: Academic Press), 307–395.
Ching, B., Chen, X. L., Yong, J. H. A., Wilson, J. M., Hiong, K. C., Sim, E. W. L., et al. (2013). Increases in apoptosis, caspase activity, and expression of p53 and bax, and the transition between two types of mitochondrion-rich cells, in the gills of the climbing perch, Anabas testudineus, during a progressive acclimation from freshwater to seawater. Front. Physiol. 4:135. doi: 10.3389/fphys.201300135
Crombie, H. J., Bell, M. V., and Tytler, P. (1996). Inhibition of sodium-plus-potassium-stimulated adenosine triphosphatase (Na+,K+-ATPase) by protein kinase C activators in the gills of Atlantic cod (Gadus morhua). Comp. Biochem. Physiol. B 113, 765–772. doi: 10.1016/0305-0491(95)02067-5
Evans, D. H. (2008). Teleost fish osmoregulation: what have we learned since August Krogh, Homer Smith, and Ancel Keys. Am. J. Physiol. Regul. Integr. Comp. Physiol. 295, R704–R713. doi: 10.1152/ajpregu.90337.2008
Feng, S. H., Leu, J. H., Yang, C. H., Fang, M. J., Huang, C. J., and Hwang, P. P. (2002). Gene expression of Na+–K+-ATPase α1 and α3 subunits in gills of the teleost Oreochromis mossambicus, adapted to different environmental salinities. Mar. Biotechnol. 4, 379–391. doi: 10.1007/s10126-002-0006-0
Feschenko, M. S., and Sweadner, K. J. (1994). Conformation-dependent phosphorylation of Na,K-ATPase by protein kinase A and protein kinase C. J. Biol. Chem. 269, 30436–30444.
Feschenko, M. S., and Sweadner, K. J. (1995). Structural basis for species-specific differences in the phosphorylation of Na,K-ATPase by protein kinase C. J. Biol. Chem. 270, 14072–71407. doi: 10.1074/jbc.270.23.14072
Furriel, R. P., Masui, D. C., McNamara, J. C., and Leone, F. A. (2004). Modulation of gill Na+, K+-ATPase activity by ammonium ions: putative coupling of nitrogen excretion and ion uptake in the freshwater shrimp Macrobrachium olfersii. J. Exp. Zool. 301, 63–74. doi: 10.1002/jez.a.20008
Gasteiger, E., Gattiker, A., Hoogland, C., Ivanyi, I., Appel, R. D., and Bairoch, A. (2003). ExPASy: the proteomics server for in-depth protein knowledge and analysis. Nucleic Acids Res. 31, 3784–3788. doi: 10.1093/nar/gkg563
Gerwick, L., Corley-Smith, G., and Bayne, C. J. (2007). Gene transcript changes in individual rainbow trout livers following an inflammatory stimulus. Fish Shellfish Immunol. 22, 157–171. doi: 10.1016/j.fsi.2006.04.003
Graham, J. B. (1997). Air-breathing Fishes: Evolution, Diversity and Adaptation. London: Academic Press.
Hall, T. A. (1999). BioEdit: a user-friendly biological sequence editor and analysis program for Windows 95/98/NT. Nucl. Acids Symp. Ser. 41, 95–98.
Hiong, K. C., Ip, Y. K., Wong, W. P., and Chew, S. F. (2014). Brain Na+/K+-ATPase α-subunit isoforms and aestivation in the African lungfish, Protopterus annectens. J. Comp. Physiol. B. doi: 10.1007/s00360-014-0809-0. [Epub ahead of print].
Hiroi, J., Kaneko, T., and Tanaka, M. (1999). In vivo sequential changes in chloride cell morphology in the yolk-sac membrane of mozambique tilapia (Oreochromis mossambicus) embryos and larvae during seawater adaptation. J. Exp. Biol. 202, 3485–3495.
Hwang, P. P., and Lee, T. H. (2007). New insights into fish ion regulation and mitochondrion-rich cells. Comp. Biochem. Physiol. A 148, 479–497. doi: 10.1016/j.cbpa.2007.06.416
Hwang, P. P., Lee, T. H., and Lin, L. Y. (2011). Ion regulation in fish gills: recent progress in the cellular and molecular mechanisms. Am. J. Physiol. Regul. Integr. Comp. Physiol. 301, R28–R47. doi: 10.1152/ajpregu.00047.2011
Ip, Y. K., and Chew, S. F. (2010). Ammonia production, excretion, toxicity, and defense in fish: a review. Front. Physiol. 1:134. doi: 10.3389/fphys.2010.00134
Ip, Y. K., Chew, S. F., and Randall, D. J. (2001a). “Ammonia toxicity, tolerance, and excretion,” in Fish Physiology, Vol. 20, eds P. A. Wright and P. M. Anderson (New York, NY: Academic Press), 109–148.
Ip, Y. K., Chew, S. F., Wilson, J. M., and Randall, D. J. (2004a). Defenses against ammonia toxicity in tropical air breathing fishes exposed to high concentration of environmental ammonia: a review. J. Comp. Physiol. B 174, 565–575. doi: 10.1007/s00360-004-0445-1
Ip, Y. K., Hiong, K. C., Wong, S. Z. H., Ching, B., Chen, X. L., Soh, M. M. L., et al. (2013). Branchial Na+:K+:2Cl− cotransporter 1 and Na+/K+-ATPase α-subunit in a brackish water-type ionocyte of the euryhaline freshwater white-rimmed stingray, Himantura signifer. Front. Physiol. 4:362. doi: 10.3389/fphys.2013.00362
Ip, Y. K., Lee, C. Y., Chew, S. F., Low, W. P., and Peng, K. W. (1993). Differences in the responses of two mudskippers to terrestrial exposure. Zool. Sci. 10, 511–519.
Ip, Y. K., Lim, C. B., Chew, S. F., Wilson, J. M., and Randall, D. J. (2001b). Partial amino acid catabolism leading to the formation of alanine in Periophthalmodon schlosseri (mudskipper): a strategy that facilitates the use of amino acids as an energy source during locomotory activity on land. J. Exp. Biol. 204, 1615–1624.
Ip, Y., K., Loong, A. M., Kuah, J. S., Sim, E. W. L., Chen, X. L., Wong, W. P., et al. (2012a). The roles of three branchial Na+/K+-ATPase α-subunit isoforms in freshwater adaptation, seawater acclimation and active ammonia excretion in Anabas testudineus. Am. J. Physiol. Regul. Integr. Comp. Physiol. 303, R112–R125. doi: 10.1152/ajpregu.00618.2011
Ip, Y. K., Randall, D. J., Kok, T. K. T., Barzaghi, C., Wright, P. A., Ballantyne, J. S., et al. (2004b). The giant mudskipper Periophthalmodon schlosseri facilitates active NH+4 excretion by increasing acid excretion and decreasing NH3 permeability in the skin. J. Exp. Biol. 207, 787–801. doi: 10.1242/jeb.00788
Ip, Y. K., Wilson, J. M., Loong, A. M., Chen, X. L., Wong, W. P., Inês Delgado, L. S., et al. (2012b). Cystic fibrosis transmembrane conductance regulator in the gills of the climbing perch, Anabas testudineus, is involved in both hypoosmotic regulation during seawater acclimation and active ammonia excretion during ammonia exposure. J. Comp. Physiol. B 182, 793–812. doi: 10.1007/s00360-012-0664-9
Ip, Y. K., Zubaidah, R. M., Liew, P. C., Loong, A. M., Hiong, K. C., Wong, W. P., et al. (2004c). African sharptooth catfish Clarias gariepinus does not detoxify ammonia to urea or amino acids but actively excretes ammonia during exposure to environmental ammonia. Physiol. Biochem. Zool. 77, 242–254. doi: 10.1086/383499
Kok, T. W. K., Lim, C. B., Lam, T. J., and Ip, Y. K. (1998). The mudskipper Periophthalmodon schlosseri respires more efficiently on land than in water and vice versa for Boleophthalmus boddaerti. J. Exp. Zool. 280, 86–90.
Laemmli, U. K. (1970). Cleavage of structural proteins during the assembly of the head bacteriophage T4. Nature 227, 680–685. doi: 10.1038/227680a0
Lee, T. H., Tsai, J. C., Fang, M. J., Yu, M. J., and Hwang, P. P. (1998). Isoform expression of Na+–K+-ATPase α-subunit in gills of the teleost Oreochromis mossambicus. Am. J. Physiol. 44, R926–R932.
Lin, Y. M., Chen, C. N., Yoshinaga, T., Tsai, S. C., Shen, I. D., and Lee, T. H. (2006). Short-term effects of hyposmotic shock on Na+/K+-ATPase expression in gills of the euryhaline milkfish, Chanos chanos. Comp. Biochem. Physiol. A 143, 406–415. doi: 10.1016/j.cbpa.2005.12.031
Loong, A. M., Chew, S. F., Wong, W. P., Lam, S. H., and Ip, Y. K. (2012). Both seawater acclimation and environmental ammonia exposure lead to increases in mRNA expression and protein abundance of Na+:K+:2Cl− cotransporter in the gills of the climbing perch, Anabas testudineus. J. Comp. Physiol. B 182, 491–506. doi: 10.1007/s00360-011-0634-7
Low, W. P., Lane, D. J. W., and Ip, Y. K. (1988). A comparative study of terrestrial adaptations of the gills in three mudskippers - Periophthalmus chrysospilos, Boleophthalmus boddaerti, and Periophthalmodon schlosseri. Biol. Bull. 175, 434–438. doi: 10.2307/1541736
Low, W. P., Lane, D. J. W., and Ip, Y. K. (1990). A comparative study of the gill morphometry in the mudskippers - Periophthalmus chrysospilos, Boleophthalmus boddaerti and Periophthalmodon schlosseri. Zool. Sci. 7, 29–38.
Masui, D. C., Furriel, R. P., McNamara, J. C., Mantelatto, F. L., and Leone, F. A. (2002). Modulation by ammonium ions of gill microsomal (Na+,K+)-ATPase in the swimming crab Callinectes danae: a possible mechanism for regulation of ammonia excretion. Comp. Biochem. Physiol. C 132, 471–482. doi: 10.1016/S1532-0456(02)00110-2
Masui, D. C., Mantelatto, F. L., McNamara, J. C., Furriel, R. P., and Leone, F. A. (2009). Na+,K+- ATPase activity in gill microsomes from the blue crab, Callinectes danae, acclimated to low salinity: novel perspectives on ammonia excretion. Comp. Biochem. Physiol. A 153, 141–148. doi: 10.1016/j.cbpa.2009.01.020
McGuffin, L. J., Bryson, K., and Jones, D. T. (2000). The PSIPRED protein structure prediction server. Bioinformatics 16, 404–405. doi: 10.1093/bioinformatics/16.4.404
Morth, J. P., Pederson, B. P., Toustrup-Jensen, M. S., Sørensen, T. L., Petersen, J., Andersen, J. P., et al. (2007). Crystal structure of the sodium-potassium pump. Nature 450, 1043–1049. doi: 10.1038/nature06419
Ogawa, H., and Toyoshima, C. (2002). Homology modeling of the cation binding sites of Na+,K+-ATPase. Proc. Natl. Acad. Sci. U.S.A. 99, 15977–15982. doi: 10.1073/pnas.202622299
Page, R. D. M. (1996). TREEVIEW: an application to display phylogenetic trees on personal computers. Comput. Appl. Biosci. 12, 357–358.
Pedersen, P. A., Nielsen, J. M., Rasmussen, J. H., and Jørgensen, P. L. (1998). Contribution of Tl+, K+, and Na+ binding of Asn776, Ser775, Thr774, Thr772, and Tyr771 in cytoplasmic part of fifth transmembrane segment in α-subunit of renal Na,K-ATPase. Biochemistry 37, 17818–17827. doi: 10.1021/bi981898w
Peng, K. W., Chew, S. F., Lim, C. B., Kuah, S. S. L., Kok, T. W. K., and Ip, Y. K. (1998). The mudskippers Periophthalmodon schlosseri and Boleophthalmus boddaerti can tolerate environmental NH3 concentration of 446 and 36 μM, respectively. Fish Physiol. Biochem. 19, 59–69. doi: 10.1023/A:1007745003948
Randall, D. J., Wilson, J. M., Peng, K. W., Kok, T. W. K., Kuah, S. S. L., Chew, S. F., et al. (1999). The mudskipper, Periophthalmodon schlosseri, actively transports NH+4 against a concentration gradient. Am. J. Physiol. Regul. Integr. Comp. Physiol. 277, R1562–R1567.
Richards, J. G., Semple, J. W., Bystriansky, J. S., and Schulte, P. M. (2003). Na+/K+-ATPase α-isoform switching in gills of rainbow trout (Oncorhynchus mykiss) during salinity transfer. J. Exp. Biol. 206, 4475–4486. doi: 10.1242/jeb.00701
Scott, G. R., Richards, J. G., Forbush, B., Isenring, P., and Schulte, P. M. (2004). Changes in gene expression in gills of the euryhaline killifish Fundulus heteroclitus after abrupt salinity transfer. Am. J. Physiol. Cell Physiol. 287, C300–C309. doi: 10.1152/ajpcell.00054.2004
Singer, T. D., Clements, K. M., Semple, J. W., Schulte, P. M., Bystriansky, J. S., Finstad, B., et al. (2002). Seawater tolerance and gene expression in two strains of Atlantic salmon smolts. Can. J. Fish Aquat. Sci. 59, 125–135. doi: 10.1139/f01-205
Tay, Y. L., Loong, A. M., Hiong, K. C., Lee, S. J., Tng, Y. Y. M., Wee, N. L. J., et al. (2006). Active ammonia transport and excretory nitrogen metabolism in the climbing perch, Anabas testudineus, during 4 days of emersion or 10 minutes of forced exercise on land. J. Exp. Biol. 209, 4475–4489. doi: 10.1242/jeb.02557
Tipsmark, C. K., and Madsen, S. S. (2001). Rapid modulation of Na+/K+-ATPase activity in osmoregulatory tissues of a salmonid fish. J. Exp. Biol. 204, 701–709.
Tipsmark, C. K., Madsen, S. S., Seidelin, M., Christensen, A. S., Cutler, C. P., and Cramb, G. (2002). Dynamics of Na+, K+, 2Cl−cotransporter and Na+,K+-ATPase expression in the branchial epithelium of brown trout (Salmo trutta) and Atlantic salmon (Salmo salar). J. Exp. Zool. 293, 106–118. doi: 10.1002/jez.10118
Towle, D. W., and Holleland, T. (1987). Ammonium ion substitutes for K+ in ATP-dependent Na+ transport by basolateral membrane-vesicles. Am. J. Physiol. Regul. Integr. Comp. Physiol. 252, R479–R489.
Towle, D. W., Paulsen, R. S., Weihrauch, D., Kordylewski, M., Salvador, C., Lignot, J. H., et al. (2001). Na++K+-ATPase in the gills of the blue crab, Callinectes sapidus: cDNA sequencing and salinity-related expression of α-subunit mRNA and protein. J. Exp. Biol. 204, 4005–4012.
Weihrauch, D., Wilkie, M. P., and Walsh, P. J. (2009). Ammonia and urea transporters in gills of fish and aquatic crustaceans. J. Exp. Biol. 212, 1716–1730. doi: 10.1242/jeb.024851
Wilson, J. M., Kok, T. W. K., Randall, D. J., Vogl, A. W., and Ip, Y. K. (1999). Fine structure of the gill epithelium of the terrestrial mudskipper, Periophthalmodon schlosseri. Cell Tissue Res. 298, 345–356. doi: 10.1007/s004419900101
Wilson, J. M., Leitão, A., Goncalves, A. F., Ferreira, C., Reis-Santos, P., Fonseca, A.-V., et al. (2007). Modulation of branchial ion transport protein expression by salinity in glass eels (Anguilla anguilla L.). Mar. Biol. 151, 1633–1645. doi: 10.1007/s00227-006-0579-7
Wilson, J. M., Randall, D. J., Donowitz, M., Vogl, A. W., and Ip, Y. K. (2000). Immunolocalization of ion-transport proteins to branchial epithelium mitochondria-rich cells in the mudskipper (Periophthalmodon schlosseri). J. Exp. Biol. 203, 2297–2310.
Wong, M. L., and Medrano, J. F. (2005). Real-time PCR for mRNA quantitation. BioTechniques 39, 75–85. doi: 10.2144/05391RV01
Wright, S. H. (1991). “The interface of animal and aqueous environment: strategies and constraints on the maintenance of solute balance,” in Biochemistry and Molecular Biology of Fishes 1, Phylogenetic and Biochemical Perspectives, eds P. W. Hochachka and T. P. Mommsen (Amsterdam: Elsevier), 165–180.
Keywords: air-breathing fish, ammonia toxicity, gills, ionocytes, nitrogen metabolism, osmoregulation
Citation: Chew SF, Hiong KC, Lam SP, Ong SW, Wee WL, Wong WP and Ip YK (2014) Functional roles of Na+/K+-ATPase in active ammonia excretion and seawater acclimation in the giant mudskipper, Periophthalmodon schlosseri. Front. Physiol. 5:158. doi: 10.3389/fphys.2014.00158
Received: 19 February 2014; Accepted: 03 April 2014;
Published online: 23 April 2014.
Edited by:
Shigehisa Hirose, Tokyo Institute of Technology, JapanReviewed by:
Christian K. Tipsmark, University of Arkansas, USAC. Michele Nawata, University of Arizona, USA
Copyright © 2014 Chew, Hiong, Lam, Ong, Wee, Wong and Ip. This is an open-access article distributed under the terms of the Creative Commons Attribution License (CC BY). The use, distribution or reproduction in other forums is permitted, provided the original author(s) or licensor are credited and that the original publication in this journal is cited, in accordance with accepted academic practice. No use, distribution or reproduction is permitted which does not comply with these terms.
*Correspondence: Shit F. Chew, Natural Sciences and Science Education, National Institute of Education, Nanyang Technological University, 1 Nanyang Walk, Singapore 637616, Singapore e-mail: sfun.chew@nie.edu.sg