- 1Developmental Tumor Biology Laboratory, Institut de Recerca Pediàtrica – Hospital Sant Joan de Déu, Barcelona, Spain
- 2Department of Oncology, Institut de Recerca Pediàtrica – Hospital Sant Joan de Déu, Barcelona, Spain
During normal development of the nervous system (NS), neural progenitor cells (NPCs) produce specialized populations of neurons and glial cells upon cell fate restriction and terminal differentiation. These sequential processes require the dynamic regulation of thousands of genes. The calcium-sensing receptor (CaSR) is temporally and spatially regulated in both neurons and glial cells during development of the NS. In particular, CaSR expression and function have been shown to play a significant role during differentiation of NPCs toward the oligodendrocyte lineage and also in maturation of cerebellar granule cell precursors (GCPs). Moreover, CaSR regulates axonal and dendritic growth in both central and peripheral nervous systems (PNSs), a process necessary for proper construction of mature neuronal networks. On the other hand, several lines of evidence support a role for CaSR in promotion of cell differentiation and inhibition of proliferation in neuroblastoma, a tumor arising from precursor cells of developing PNS. Thus, among the variety of NS functions in which the CaSR participates, this mini-review focuses on its role in differentiation of normal and tumoral cells. Current knowledge of the mechanisms responsible for CaSR regulation and function in these contexts is also discussed, together with the therapeutic opportunities provided by CaSR allosteric modulators.
The calcium-sensing receptor (CaSR), a G protein-coupled receptor (GPCR) whose primary ligand is calcium, was initially identified in the parathyroid gland, where it regulates calcium homeostasis (Brown et al., 1993). Subsequent studies found that this GPCR is present in many other organs (Riccardi and Kemp, 2012) and in some cancers (Brennan et al., 2013), where it plays versatile roles. CaSR also participates in a wide range of cellular functions that are important for proper development of the nervous system (NS; Bandyopadhyay et al., 2010; Ruat and Traiffort, 2013), and any alterations in its expression and/or function may lead to disease, including tumors.
During development, cell fate restriction occurs in the NS where specialized neuronal and glial populations arise from neural progenitor cells (NPCs). This is achieved without changes of DNA sequence through the coordinated regulation of gene expression promoted by cell-intrinsic programs, such as epigenetic mechanisms and transcription factors, as well as extracellular cues. Increasing evidence indicates that proper CaSR expression and function is important during differentiation of specific neural precursor cells upon commitment toward neuronal and glial fates. CaSR also plays significant roles during differentiation of neuroblastoma, a developmental tumor of the peripheral nervous system (PNS). Therefore, among many other functions of the NS in which it participates (Bandyopadhyay et al., 2010; Ruat and Traiffort, 2013), this mini-review focuses on the role of CaSR in differentiation, a cellular process that is crucial for both normal development and tumor biology.
Role of CaSR in Differentiation of Normal Developing Nervous System
The rat Casr gene was cloned from a striatal cDNA library by homology screening (Ruat et al., 1995). Subsequent expression analyses identified its presence in almost all regions of the central nervous system (CNS) including hypothalamus, striatum, hippocampus, pituitary, cerebellum, brainstem, circumventricular organs, and spinal cord (Ruat et al., 1995; Chattopadhyay et al., 1997; Rogers et al., 1997).
The CNS is composed of neurons and three major populations of glial cells, astrocytes, oligodendrocytes, and microglia. Although, expression of CaSR has been reported in astrocytes (Chattopadhyay et al., 2000; Dal Pra et al., 2005) and microglia (Chattopadhyay et al., 1999a), the CaSR was first localized to nerve terminals of neurons and fiber tracts (Ruat et al., 1995). Nerve tracts consist of axons wrapped by myelin sheaths which are produced by oligodendrocytes in the CNS and by Schwann cells in the PNS. In order to be able to produce myelin, oligodendrocyes precursor cells (OPCs) progress through a series of differentiation steps, lose their capacity to proliferate and migrate, and finally generate mature oligodendrocytes (Barateiro and Fernandes, 2014).
Several lines of evidence support a role for CaSR in this differentiation process. First, a period of increased CaSR expression was identified during rat postnatal development in myelinated structures (Chattopadhyay et al., 1998; Ferry et al., 2000). By double in situ hybridization, Casr and myelin basic protein (Mbp) mRNAs were shown to co-localize in cerebellum, brainstem, corpus callosum, fimbria of the hippocampus, stria medullaris, and lateral olfactory tracts during myelogenesis. Second, Northern blot and reverse transcription polymerase chain reaction (RT-PCR) confirmed Casr mRNA expression in purified oligodendrocytes. Moreover, exposure to high and calcimimetic NPS R-568 resulted in phosphatidylinositol hydrolysis and intracellular Ca2+ () mobilization (Chattopadhyay et al., 1998; Ferry et al., 2000). Altogether, these data indicate that a functional CaSR is present in olygodendrocytes and temporally regulated during OPCs differentiation.
The role of CaSR in the transition of neural precursor cells toward the oligodendrocyte lineage has also been established (Chattopadhyay et al., 2008). To this end, neural stem cells were isolated from fetal rat brains and induced to commit to neuronal, oligodendrocyte, or astrocytic lineages. Casr expression increased in OPCs, remained high during the premyelinating stage and declined in mature oligodendrocytes. Furthermore, Mbp mRNA levels increased in OPCs exposed to high or spermidine for 1–3 days. This phenotype was blocked by overexpression of a naturally-occurring dominant-negative CaSR variant p.Arg185Gln (Bai et al., 1997). Furthermore, Mbp mRNA levels were significantly reduced in the cerebellum of 2-week old Casr-null (Casr−∕−) mice as compared to age-matched Casr+∕+ mice. Altogether, these results indicate that prolonged CaSR activation promotes maturation of OPCs. However, a brief exposure to high induced OPCs proliferation, suggesting that acute and long-term activation of CaSR differentially affects cell proliferation and differentiation (Chattopadhyay et al., 2008).
Studies conducted in Casr−∕− mice (Liu et al., 2013), a mouse model of human neonatal severe hyperparathyroidism (Ho et al., 1995), have provided direct in vivo evidence for CaSR roles during differentiation of CNS. In these mice, both brain weight and size were reported to be lower than that of wild-type littermates during the first 2 weeks of postnatal development. Small brain size was associated with a reduced number of cells and proliferation rates, but deletion of the parathyroid hormone (Pth) gene, which corrects hyperparathyroidism, hypercalcemia, and hypophosphatemia, normalized these alterations. Interestingly, decreased expression of neuronal (neuronal nuclear antigen, NeuN) and glial (glial fibrillary acidic protein and MBP) differentiation markers were detected in these brains, and levels of expression were not normalized upon deletion of the Pth gene, thus suggesting that CaSR is necessary for differentiation of neural progenitors toward neuronal and glial fates, but not for their proliferation.
More recently, the role of CaSR has also been evaluated in the developing cerebellum (Tharmalingam et al., 2016) during a period that includes initial proliferation of granule cell precursors (GCPs) in the external granule cell layer (EGL) followed by differentiation and cell cycle exit. At later stages, differentiated GCPs migrate within the EGL, a process called tangential migration, and then toward the internal granule cell layer (IGL) by radial migration. Immunoblots showed high rat Casr protein expression in the cerebellum from P7 to P18, a period during which maximal GCPs migration occurs. Moreover, CaSR allosteric activators NPS R-568 and R-467 increased GCPs migration in vitro, and these effects were blocked by calcilytic NPS 2143 (Bandyopadhyay et al., 2010). Also, calcimimetics promoted increased radial migration of GCPs from the EGL into the IGL. Specificity of this phenotype was corroborated by experiments conducted with NPS 2143. Interestingly, the number of cells positive for NeuN was higher in rats treated with CaSR allosteric activators and reduced in those receiving NPS 2143 when compared to controls. Moreover, rats exposed to the calcilytic also showed significantly increased numbers of Ki67-positive GCPs, a nuclear marker of cell proliferation. Together, these studies argue that CaSR expression and function are necessary for proper migration and differentiation of GCPs.
While several studies have analyzed the expression and function of CaSR in the CNS, much less attention has been devoted to the role of this receptor in the PNS. Several lines of evidence support that CaSR expression and function during normal formation of the PNS are critical for axonal and dendritic growth. Proper regulation of axon growth and branching are crucial for constructing functional, mature neuronal networks. These processes are regulated by extracellular cues, growth factors, and morphogens that signal through receptors, activate intracellular signaling cascades and regulate cytoskeletal dynamics (Kalil and Dent, 2014). Compelling data reported by Vizard et al. (2008) support that CaSR would be among receptors that integrate extracellular signals during axon growth and branching. These authors showed that a brief period of increased Casr mRNA expression occurs in mouse neurons of the superior cervical ganglion (SCG) from embryonic day 16 (E16) until E18, a time when murine sympathetic axon are branching at their targets. They functionally showed that neurons at this peak of Casr expression display enhanced axonal growth when exposed to high and calcimimetic NPS R-467, whereas this output is blunted by blocking CaSR function by either calcilytic NPS 89636, Casr deletion, or overexpression of a dominant-negative CaSR (Bai et al., 1997). Also, a significant reduction in the iris sympathetic innervation density was shown in Casr−∕− mice. Furthermore, a similar role was demonstrated in hippocampal pyramidal neurons, thus providing evidence for a CaSR role in neuronal growth and branching in both PNS and CNS.
Role of CaSR in Differentiation of Tumoral Developing Nervous System
Childhood solid tumors have been recognized as a group of cancers significantly different from adult neoplasias (Scotting et al., 2005; Marshall et al., 2014). They arise from precursor cells during organogenesis and retain many of the morphological and biological features of their undifferentiated, highly proliferative, and sometimes migratory normal cells of origin.
Neuroblastomas originate from PNS precursor cells (Cheung and Dyer, 2013) which in turn derive from trunk neural crest cells. This is a transient population of embryonic cells (LeDouarin, 1982) that generate several derivatives including neurons and glia of the sympathetic NS (Bronner and LeDouarin, 2012). This process involves a period of cellular proliferation, followed by delamination, migration, specification, and terminal differentiation. To produce glial cells and neurons, a portion of trunk neural crest cells migrate along a ventral pathway (Henion and Weston, 1997). Several environmental cues contribute to their fate restriction and, upon terminal differentiation, they give rise to the sympathetic ganglia and medullary region of the adrenal gland (Anderson and Axel, 1986; Anderson et al., 1991).
The potential origin of neuroblastomas in neural crest precursor cells “blocked” at different stages of this process, as well as a combination of various molecular and genetic events, is thought to underlie the heterogeneity of this group of tumors that include both benign and malignant cases (Brodeur, 2003; Maris, 2010; van Noesel, 2012). The most relevant factors associated with these different clinical behaviors of neuroblastomas are age at diagnosis, clinical stage, MYCN amplification, alterations of ploidy, numerical and structural chromosomal abnormalities, and histological degree of differentiation (Ambros et al., 1996; Bown et al., 1999; Janoueix-Lerosey et al., 2008; Molenaar et al., 2012; Cheung and Dyer, 2013; Pugh et al., 2013).
The first genetic alteration to be described in neuroblastoma was the amplification of the oncogene MYCN (Schwab et al., 1983). It is present in only 22% of these tumors but it is the most significant genetic predictor of poor outcome (Brodeur et al., 1984). MYCN is part of the basic helix-loop-helix family of transcription factors that also includes MYC (c-MYC) and MYCL (Zimmerman et al., 1986; Gustafson and Weiss, 2010). MYCN is a critical promoter of cell proliferation while inhibiting differentiation and apoptosis in early post-migratory neural crest cells and also during CNS neurogenesis (Knoepfler et al., 2002). This is achieved by a complex network of interactions with other transcription factors and epigenetic mechanisms that cooperate to regulate a wide array of genes (Huang and Weiss, 2013). Intriguingly, MYC, which exhibits some structural and functional similarities with MYCN, is an important transcriptional regulator in the transition from proliferating to differentiating OPCs (Magri et al., 2014).
Neuroblastic tumors are composed of two main cellular components: neuroblasts, of neuronal origin, and glial, Schwannian-like cells. Classifications based on the degree of neuroblasts maturation and the extent of the glial component showed that differentiated tumors were associated with good clinical outcome (Hughes et al., 1974; Shimada et al., 1999, 2001). A variety of proteins participate in the differentiation processes of neuroblastomas (reviewed in Mohlin et al., 2011). Among them, the CaSR was found to be highly expressed in differentiated neuroblastic tumors and up-regulated upon differentiation induction (de Torres et al., 2009). CaSR was previously identified in adult CNS tumors (Chattopadhyay et al., 1999b, 2000), but it had not been reported in any developmental malignancy. In neuroblastoma, CaSR mRNA expression significantly correlated with several factors associated with good outcome such as age at diagnosis <1 year, low clinical stage and differentiated histology. Immunohistochemistry showed that undifferentiated neuroblasts were mostly CaSR-negative while even the earliest stages of neuroblast differentiation displayed CaSR immunostaining. When present, glial cells were also strongly positive for CaSR. Moreover, upon neuroblastoma differentiation induction, increased CaSR expression was seen both in clinical specimens obtained after treatment, and in vitro, at early phases of neuronal differentiation induced by retinoic acid.
In accordance with these data, CASR gene silencing by epigenetic mechanisms was found in undifferentiated, MYCN-amplified, aggressive neuroblastomas (Casalà et al., 2013). These mechanisms included promoter 2 hypermethylation and histone modifications. CpG islands are clusters of GC dinucleotides located in promoter regions, and also in intragenic regions, that are usually unmethylated (Deaton and Bird, 2011). Methylation of the fifth position of cytosines at CpG islands around promoter regions is a mechanism of gene silencing that inactivates tumor-suppressor genes (Herman et al., 1996; Timp and Feinberg, 2013) and also contributes to gene modulation during cell type specification and lineage commitment (Hirabayashi and Gotoh, 2010; Hu et al., 2014). A particular region of the CpG island encompassing CASR gene promoter 2 was found to be hypermethylated in 25% primary neuroblastomas, in association with reduced CaSR mRNA expression, MYCN amplification, undifferentiated histopathology and other factors of poor outcome. In neuroblastoma cell lines, treatment with demethylating agent 5′aza-2-deoxycitidine and/or histone deacetylase inhibitor trichostatin A decreased the percentage of methylated cytosines in this specific region of CASR gene promoter and concomitantly restored CaSR expression in MYCN-amplified cell lines. Association of MYCN amplification and epigenetic silencing of several genes in neuroblastoma had been previously reported (Alaminos et al., 2004), although the underlying mechanisms are still under investigation (Perini et al., 2005; Hervouet et al., 2009; Murphy et al., 2009, 2011; He et al., 2013). In addition, monosomy of chromosome 3, where the human CASR gene resides, was observed in >90% of primary neuroblastic tumors of all subgroups by interphase fluorescence in situ hybridization. Interestingly, other genes that exert tumor-suppressor functions in neuroblastoma, like RASSF1A, are also located on chromosome 3 and hypermethylated in neuroblastic tumors and phaeochromocytomas, which are also PNS tumors (Astuti et al., 2001). Furthermore, ectopic overexpression of full-length CaSR in two MYCN-amplified cell lines in which this gene was previously shown to be silenced by promoter hypermethylation significantly decreased their proliferative and tumorigenic capacities. Moreover, acute exposure to high concentrations prompted their apoptosis. In all, these data provided functional evidence of the biological relevance of CaSR epigenetic silencing in neuroblastoma biology.
In addition, when non-synonimous genetic variants located at the intracellular tail encoded by exon 7 of the CASR gene were analyzed in a cohort of neuroblastoma patients, a haplotype including a polymorphism considered to mildly reduce CaSR activity (Heath et al., 1996; Cole et al., 1999; Scillitani et al., 2004, 2007; Hu and Spiegel, 2007; Vezzoli et al., 2007; Yun et al., 2007) was associated with poor outcome (Masvidal et al., 2013).
Finally, a recent study has shown that cinacalcet, an allosteric activator of the CaSR approved for clinical use (Nemeth et al., 1998), inhibits neuroblastoma tumor growth in vitro and in vivo (Rodríguez-Hernández et al., 2016). Mechanisms involved include ER stress coupled to apoptosis dependent on phospholipase C activation in MYCN-amplified neuroblastoma cells and, irrespective of MYCN status, differentiation of surviving cells. Induction of differentiation was also observed upon prolonged exposure to cinacalcet in vivo. Genome-wide gene expression analysis by microarrays of xenografts showed up-regulation of numerous genes involved in neuroblastoma differentiation, like NTRK3 (Nakagawara et al., 1993) and GABRA3 (Roberts et al., 2004). Gene Ontology categories also unveiled up-regulation of genes involved in axon growth like doublecortin and ephrins (Kalil and Dent, 2014). Concomitantly, genes that critically support neuroblastoma proliferation were down-regulated, such as MYCN, inhibitor of differentiation 2 (ID2) and MYB (Figure 1). ID2 is a helix-loop-helix transcription factor controlled by MYC proteins that blocks differentiation and promotes cell proliferation (Lasorella et al., 2000), and MYB is a transcription factor that cooperates with MYCN in cell cycle regulation of MYCN-amplified neuroblastomas (Gualdrini et al., 2010). Quite unexpectedly, cinacalcet also promoted up-regulation of cancer-testis antigens, a family of proteins that are almost exclusively expressed in tumor cells and are thus considered ideal targets for immunotherapy (Fratta et al., 2011). Although mechanisms involved are not yet understood, histone acetyltransferases such as p300 and CREB binding protein might be recruited, taking into account that these genes are mainly regulated by epigenetic mechanisms (Rao et al., 2011).
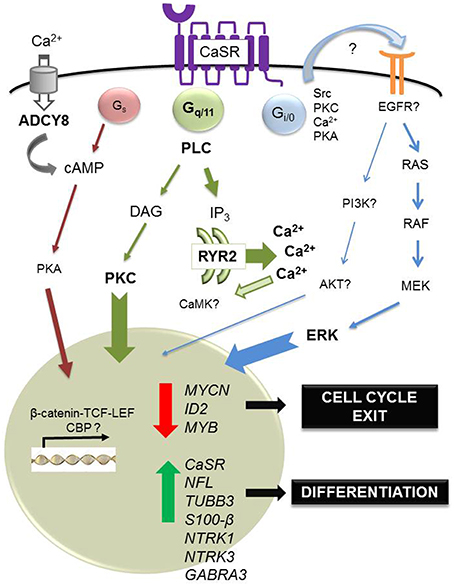
Figure 1. Regulation of differentiation by calcium-sensing receptor in neuroblastoma. In neuroblastoma, the CaSR is expressed in benign, differentiated tumors. In this tumoral context, the main physiological ligand remains unknown. However, acute exposure to high extracellular concentrations of Ca2+ induces apoptosis of CaSR-positive, MYCN-amplified cells, dependent on sustained activation of ERK. Also, short in vitro exposure to cinacalcet, an allosteric activator of the CaSR approved for clinical use, induces endoplasmic reticulum (ER) stress coupled to apoptosis in CaSR-positive, MYCN-amplified neuroblastoma cells. This output is dependent on activation of phospholipase C (PLC). Massive Ca2+ exit from the ER up-regulates ryanodine receptor 2 (RYR2) and activation of adenylyl cyclase type 8 (ADCY8) via capacitative calcium entry, a mechanism triggered by depletion of intracellular Ca2+ stores. Furthermore, prolonged treatment with cinacalcet promotes up-regulation of genes associated with neuroblastoma differentiation (NFL, TUBB3, S100-β, NTRK1, NTRK3, GABRA3) and down-regulation of genes that are critical for proliferation of these tumors (MYCN, ID2, MYB). Concomitantly, sustained exposure to cinacalcet also induces up-regulation of CaSR and increased expression of cancer-testis antigens.
In summary, studies conducted in neuroblastoma indicate that CaSR promotes differentiation and inhibits proliferation in this malignancy. Also, they are in accordance with data obtained in developing NS supporting that CaSR plays a significant role in differentiation processes of specific NPCs upon commitment toward neuronal and glial lineages. More importantly, neuroblastoma models show that pharmacological modulation of CaSR activity can provide novel therapeutic opportunities.
Conclusions and Future Directions
Over the past decades, the patterns of expression of CaSR in the NS have been described. However, our understanding of CaSR regulation and functions during normal and pathological development of CNS and PNS is incomplete. In the coming years, epigenetic mechanisms responsible for CaSR regulation during formation of NS will be elucidated. They will probably involve cytosines methylation and demethylation by DNA methyltransferases and 10–11 translocation enzymes (Hahn et al., 2013), histones modifications and non-coding RNAs. Also, the complex interplay of these mechanisms with transcription factors such as the MYC family will be characterized. This knowledge, together with a precise picture of signaling pathways downstream of CaSR during differentiation processes, will help to evaluate whether pharmacological modulation of this GPCR might be beneficial in the treatment of NS developmental diseases.
Author Contributions
CdT is the Principal Investigator of the project. SM-L, CR-H, and MG are postdoctoral researchers working in the project, notably contributing to the development of both execution and conception of experiments.
Conflict of Interest Statement
CdT holds a patent, WO 2013144397 (A1), and a patent submission (PCT/ES2015/070561) on cinacalcet.
The other authors declare that the research was conducted in the absence of any commercial or financial relationships that could be construed as a potential conflict of interest.
Acknowledgments
We apologize that, due to space constraints, we could not cite many relevant studies. We wish to thank Gerda E. Breitwieser for English editing and critical reading of the manuscript. Also, insight from the anonymous reviewers is acknowledged. This work is supported by Spanish Ministry of Health (FIS PI14/00040) to CdT.
References
Alaminos, M., Davalos, V., Cheung, N. K., Gerald, W. L., and Esteller, M. (2004). Clustering of gene hypermethylation associated with clinical risk groups in neuroblastoma. J. Natl. Cancer Inst. 96, 1208–1219. doi: 10.1093/jnci/djh224
Ambros, I. M., Zellner, A., Roald, B., Amann, G., Ladenstein, R., Printz, D., et al. (1996). Role of ploidy, chromosome 1p, and Schwann cells in the maturation of neuroblastoma. N. Engl. J. Med. 334, 1505–1511. doi: 10.1056/NEJM199606063342304
Anderson, D. J., and Axel, R. (1986). A bipotential neuroendocrine precursor whose choice of cell fate is determined by NGF and glucocorticoids. Cell 47, 1079–1090. doi: 10.1016/0092-8674(86)90823-8
Anderson, D. J., Carnahan, J. F., Michelsohn, A., and Patterson, P. H. (1991). Antibody markers identify a common progenitor to sympathetic neurons and chromaffin cells in vivo and reveal the timing of commitment to neuronal differentiation in the sympathoadrenal lineage. J. Neurosci. 11, 3507–3519.
Astuti, D., Agathanggelou, A., Honorio, S., Dallol, A., Martinsson, T., Kogner, P., et al. (2001). RASSF1A promoter region CpG island hypermethylation in phaeochromocytomas and neuroblastoma tumours. Oncogene 20, 7573–7577. doi: 10.1038/sj.onc.1204968
Bai, M., Pearce, S. H., Kifor, O., Trivedi, S., Stauffer, U. G., Thakker, R. V., et al. (1997). In vivo and in vitro characterization of neonatal hyperparathyroidism resulting from a de novo, heterozygous mutation in the Ca2+-sensing receptor gene: normal maternal calcium homeostasis as a cause of secondary hyperparathyroidism in familial benign hypocalciuric hypercalcemia. J. Clin. Invest. 99, 88–96. doi: 10.1172/JCI119137
Bandyopadhyay, S., Tfelt-Hansen, J., and Chattopadhyay, N. (2010). Diverse roles of extracellular calcium-sensing receptor in the central nervous system. J. Neurosci. Res. 88, 2073–2082. doi: 10.1002/jnr.22391
Barateiro, A., and Fernandes, A. (2014). Temporal oligodendrocyte lineage progression: in vitro models of proliferation, differentiation and myelination. Biochim. Biophys. Acta 1843, 1917–1929. doi: 10.1016/j.bbamcr.2014.04.018
Bown, N., Cotterill, S., Lastowska, M., O'Neill, S., Pearson, A. D., Plantaz, D., et al. (1999). Gain of chromosome arm 17q and adverse outcome in patients with neuroblastoma. N. Engl. J. Med. 340, 1954–1961. doi: 10.1056/NEJM199906243402504
Brennan, S. C., Thiem, U., Roth, S., Aggarwal, A., Fetahu, ISh, Tennakoon, S., et al. (2013). Calcium sensing receptor signalling in physiology and cancer. Biochim. Biophys. Acta 1833, 1732–1744. doi: 10.1016/j.bbamcr.2012.12.011
Brodeur, G. M. (2003). Neuroblastoma: biological insights into a clinical enigma. Nat. Rev. Cancer 3, 203–216. doi: 10.1038/nrc1014
Brodeur, G. M., Seeger, R. C., Schwab, M., Varmus, H. E., and Bishop, J. M. (1984). Amplification of N-myc in untreated human neuroblastomas correlates with advanced disease stage. Science 224, 1121–1124. doi: 10.1126/science.6719137
Bronner, M. E., and LeDouarin, N. M. (2012). Development and evolution of the neural crest: an overview. Dev. Biol. 366, 2–9. doi: 10.1016/j.ydbio.2011.12.042
Brown, E. M., Gamba, G., Riccardi, D., Lombardi, M., Butters, R., Kifor, O., et al. (1993). Cloning and characterization of an extracellular Ca(2+)-sensing receptor from bovine parathyroid. Nature 366, 575–580. doi: 10.1038/366575a0
Casalà, C., Gil-Guiñón, E., Ordóñez, J. L., Miguel-Queralt, S., Rodríguez, E., Galván, P., et al. (2013). The calcium-sensing receptor is silenced by genetic and epigenetic mechanisms in unfavorable neuroblastomas and its reactivation induces ERK1/2-dependent apoptosis. Carcinogenesis 34, 268–276. doi: 10.1093/carcin/bgs338
Chattopadhyay, N., Espinosa-Jeffrey, A., Tfelt-Hansen, J., Yano, S., Bandyopadhyay, S., Brown, E. M., et al. (2008). Calcium receptor expression and function in oligodendrocyte commitment and lineage progression: potential impact on reduced myelin basic protein in CaR-null mice. J. Neurosci. Res. 86, 215921–215967. doi: 10.1002/jnr.21662
Chattopadhyay, N., Evliyaoglu, C., Heese, O., Carroll, R., Sanders, J., Black, P., et al. (2000). Regulation of secretion of PTHrP by Ca(2+)-sensing receptor in human astrocytes, astrocytomas, and meningiomas. Am. J. Physiol. Cell. Physiol. 279, C691–C699. Available online at: http://ajpcell.physiology.org/content/279/3/C691.long
Chattopadhyay, N., Legradi, G., Bai, M., Kifor, O., Ye, C., Vassilev, P. M., et al. (1997). Calcium-sensing receptor in the rat hippocampus: a developmental study. Brain Res. Dev. Brain Res. 100, 13–21. doi: 10.1016/S0165-3806(97)00009-6
Chattopadhyay, N., Ye, C. P., Yamaguchi, T., Kerner, R., Vassilev, P. M., and Brown, E. M. (1999a). Extracellular calcium-sensing receptor induces cellular proliferation and activation of a nonselective cation channel in U373 human astrocytoma cells. Brain Res. 851, 116–124. doi: 10.1016/S0006-8993(99)02132-0
Chattopadhyay, N., Ye, C. P., Yamaguchi, T., Kifor, O., Vassilev, P. M., Nishimura, R., et al. (1998). Extracellular calcium-sensing receptor in rat oligodendrocytes: expression and potential role in regulation of cellular proliferation and an outward K+ channel. Glia 24, 449–458.
Chattopadhyay, N., Ye, C., Yamaguchi, T., Nakai, M., Kifor, O., Vassilev, P. M., et al. (1999b). The extracellular calcium-sensing receptor is expressed in rat microglia and modulates an outward K+ channel. J. Neurochem. 72, 1915–1922. doi: 10.1046/j.1471-4159.1999.0721915.x
Cheung, N. K., and Dyer, M. A. (2013). Neuroblastoma: developmental biology, cancer genomics and immunotherapy. Nat. Rev. Cancer 13, 397–411. doi: 10.1038/nrc3526
Cole, D. E., Peltekova, V. D., Rubin, L. A., Hawker, G. A., Vieth, R., Liew, C. C., et al. (1999). A986S polymorphism of the calcium-sensing receptor and circulating calcium concentrations. Lancet 353, 112–115. doi: 10.1016/S0140-6736(98)06434-4
Dal Pra, I., Chiarini, A., Nemeth, E. F., Armato, U., and Whitfield, J. F. (2005). Roles of Ca2+ and the Ca2+-sensing receptor (CASR) in the expression of inducible NOS (nitric oxide synthase)-2 and its BH4 (tetrahydrobiopterin)-dependent activation in cytokine-stimulated adult human astrocytes. J. Cell. Biochem. 96, 428–438. doi: 10.1002/jcb.20511
Deaton, A. M., and Bird, A. (2011). CpG islands and the regulation of transcription. Genes Dev. 25, 1010–1022. doi: 10.1101/gad.2037511
de Torres, C., Beleta, H., Díaz, R., Toran, N., Rodríguez, E., Lavarino, C., et al. (2009). The calcium-sensing receptor and parathyroid hormone-related protein are expressed in differentiated, favorable neuroblastic tumors. Cancer 115, 2792–2803. doi: 10.1002/cncr.24304
Ferry, S., Traiffort, E., Stinnakre, J., and Ruat, M. (2000). Developmental and adult expression of rat calcium-sensing receptor transcripts in neurons and oligodendrocytes. Eur. J. Neurosci. 12, 872–884. doi: 10.1046/j.1460-9568.2000.00980.x
Fratta, E., Coral, S., Covre, A., Parisi, G., Colizzi, F., Danielli, R., et al. (2011). The biology of cancer testis antigens: putative function, regulation and therapeutic potential. Mol. Oncol. 5, 164–182. doi: 10.1016/j.molonc.2011.02.001
Gualdrini, F., Corvetta, D., Cantilena, S., Chayka, O., Tanno, B., Raschellà, G., et al. (2010). Addiction of MYCN amplified tumours to B-MYB underscores a reciprocal regulatory loop. Oncotarget 1, 278–288. doi: 10.18632/oncotarget.138
Gustafson, W. C., and Weiss, W. A. (2010). Myc proteins as therapeutic targets. Oncogene 29, 1249–1259. doi: 10.1038/onc.2009.512
Hahn, M. A., Qiu, R., Wu, X., Li, A. X., Zhang, H., Wang, J., et al. (2013). Dynamics of 5-hydroxymethylcytosine and chromatin marks in mammalian neurogenesis. Cell Rep. 3, 291–300. doi: 10.1016/j.celrep.2013.01.011
He, S., Liu, Z., Oh, D. Y., and Thiele, C. J. (2013). MYCN and the epigenome. Front. Oncol. 25, 3:1. doi: 10.3389/fonc.2013.00001
Heath, H. III, Odelberg, S., Jackson, C. E., Teh, B. T., Hayward, N., Larsson, C., et al. (1996). Clustered inactivating mutations and benign polymorphisms of the calcium receptor gene in familial benign hypocalciuric hypercalcemia suggest receptor functional domains. J. Clin. Endocrinol. Metab. 81, 1312–1317. doi: 10.1210/jcem.81.4.8636323
Henion, P. D., and Weston, J. A. (1997). Timing and pattern of cell fate restrictions in the neural crest lineage. Development 124, 4351–4359.
Herman, J. G., Jen, J., Merlo, A., and Baylin, S. B. (1996). Hypermethylation-associated inactivation indicates a tumor suppressor role for p15INK4B. Cancer Res. 56:722–727.
Hervouet, E., Vallette, F. M., and Cartron, P. F. (2009). Dnmt3/transcription factor interactions as crucial players in targeted DNA methylation. Epigenetics 4, 487–499. doi: 10.4161/epi.4.7.9883
Hirabayashi, Y., and Gotoh, Y. (2010). Epigenetic control of neural precursor cell fate during development. Nat. Rev. Neurosci. 11, 377–388. doi: 10.1038/nrn2810
Ho, C., Conner, D. A., Pollak, M. R., Ladd, D. J., Kifor, O., Warren, H. B., et al. (1995). A mouse model of human familial hypocalciuric hypercalcemia and neonatal severe hyperparathyroidism. Nat. Genet. 11, 389–394. doi: 10.1038/ng1295-389
Hu, J., and Spiegel, A. M. (2007). Structure and function of the human calcium-sensing receptor: insights from natural and engineered mutations and allosteric modulators. J. Cell. Mol. Med. 11, 908–922. doi: 10.1111/j.1582-4934.2007.00096.x
Hu, N., Strobl-Mazzulla, P. H., and Bronner, M. E. (2014). Epigenetic regulation in neural crest development. Dev. Biol. 396, 159–168. doi: 10.1016/j.ydbio.2014.09.034
Huang, M., and Weiss, W. A. (2013). Neuroblastoma and MYCN. Cold Spring Harb. Perspect. Med. 3:a014415. doi: 10.1101/cshperspect.a014415
Hughes, M., Marsden, H. B., and Palmer, M. K. (1974). Histologic patterns of neuroblastoma related to prognosis and clinical staging. Cancer 34, 1706–1711.
Janoueix-Lerosey, I., Lequin, D., Brugières, L., Ribeiro, A., de Pontual, L., Combaret, V., et al. (2008). Somatic and germline activating mutations of the ALK kinase receptor in neuroblastoma. Nature 455, 967–970. doi: 10.1038/nature07398
Kalil, K., and Dent, E. W. (2014). Branch management: mechanisms of axon branching in the developing vertebrate CNS. Nat. Rev. Neurosci. 15, 7–18. doi: 10.1038/nrn3650
Knoepfler, P. S., Cheng, P. F., and Eisenman, R. N. (2002). N-myc is essential during neurogenesis for the rapid expansion of progenitor cell populations and the inhibition of neuronal differentiation. Genes Dev. 16, 2699–2712. doi: 10.1101/gad.1021202
Lasorella, A., Noseda, M., Beyna, M., Yokota, Y., and Iavarone, A. (2000). Id2 is a retinoblastoma protein target and mediates signalling by Myc oncoproteins. Nature 407, 592–598. doi: 10.1038/35036504
Liu, X. L., Lu, Y. S., Gao, J. Y., Marshall, C., Xiao, M., Miao, D. S., et al. (2013). Calcium sensing receptor absence delays postnatal brain development via direct and indirect mechanisms. Mol. Neurobiol. 48, 590–600. doi: 10.1007/s12035-013-8448-0
Magri, L., Gacias, M., Wu, M., Swiss, V. A., Janssen, W. G., and Casaccia, P. (2014). c-Myc-dependent transcriptional regulation of cell cycle and nucleosomal histones during oligodendrocyte differentiation. Neuroscience 276, 72–86. doi: 10.1016/j.neuroscience.2014.01.051
Maris, J. M. (2010). Recent advances in neuroblastoma. N. Engl. J. Med. 362, 2202–2211. doi: 10.1056/NEJMra0804577
Marshall, G. M., Carter, D. R., Cheung, B. B., Liu, T., Mateos, M. K., Meyerowitz, J. G., et al. (2014). The prenatal origins of cancer. Nat. Rev. Cancer 14, 277–289. doi: 10.1038/nrc3679
Masvidal, L., Iniesta, R., Casalà, C., Galván, P., Rodríguez, E., Lavarino, C., et al. (2013). Polymorphisms in the calcium-sensing receptor gene are associated with clinical outcome of neuroblastoma. PLoS ONE 8:e59762. doi: 10.1371/journal.pone.0059762
Mohlin, S. A., Wigerup, C., and Påhlman, S. (2011). Neuroblastoma aggressiveness in relation to sympathetic neuronal differentiation stage. Semin. Cancer Biol. 21, 276–282. doi: 10.1016/j.semcancer.2011.09.002
Molenaar, J. J., Koster, J., Zwijnenburg, D. A., van Sluis, P., Valentijn, L. J., van der Ploeg, I., et al. (2012). Sequencing of neuroblastoma identifies chromothripsis and defects in neuritogenesis genes. Nature 483, 589–593. doi: 10.1038/nature10910
Murphy, D. M., Buckley, P. G., Bryan, K., Das, S., Alcock, L., Foley, N. H., et al. (2009). Global MYCN transcription factor binding analysis in neuroblastoma reveals association with distinct E-box motifs and regions of DNA hypermethylation. PLoS ONE 4:e8154. doi: 10.1371/journal.pone.0008154
Murphy, D. M., Buckley, P. G., Das, S., Watters, K. M., Bryan, K., and Stallings, R. L. (2011). Co-localization of the oncogenic transcription factor MYCN and the DNA methyl binding protein MeCP2 at genomic sites in neuroblastoma. PLoS ONE 6:e21436. doi: 10.1371/journal.pone.0021436
Nakagawara, A., Arima-Nakagawara, M., Scavarda, N. J., Azar, C. G., Cantor, A. B., and Brodeur, G. M. (1993). Association between high levels of expression of the TRK gene and favorable outcome in human neuroblastoma. N. Engl. J. Med. 328, 847–854. doi: 10.1056/NEJM199303253281205
Nemeth, E. F., Steffey, M. E., Hammerland, L. G., Hung, B. C., Van Wagenen, B. C., DelMar, G., et al. (1998). Calcimimetics with potent and selective activity on the parathyroid calcium receptor. Proc. Natl. Acad. Sci. U.S.A. 95, 4040–4045. doi: 10.1073/pnas.95.7.4040
Perini, G., Diolaiti, D., Porro, A., and Della Valle, G. (2005). In vivo transcriptional regulation of N-Myc target genes is controlled by E-box methylation. Proc. Natl. Acad. Sci. U.S.A. 102, 12117–121122. doi: 10.1073/pnas.0409097102
Pugh, T. J., Morozova, O., Attiyeh, E. F., Asgharzadeh, S., Wei, J. S., Auclair, D., et al. (2013). The genetic landscape of high-risk neuroblastoma. Nat. Genet. 45, 279–284. doi: 10.1038/ng.2529
Rao, M., Chinnasamy, N., Hong, J. A., Zhang, Y., Zhang, M., Xi, S., et al. (2011). Inhibition of histone lysine methylation enhances cancer-testis antigen expression in lung cancer cells: implications for adoptive immunotherapy of cancer. Cancer Res. 71, 4192–4204. doi: 10.1158/0008-5472.CAN-10-2442
Riccardi, D., and Kemp, P. J. (2012). The calcium-sensing receptor beyond extracellular calcium homeostasis: conception, development, adult physiology, and disease. Annu. Rev. Physiol. 74, 271–297. doi: 10.1146/annurev-physiol-020911-153318
Roberts, S. S., Mori, M., Pattee, P., Lapidus, J., Mathews, R., O'Malley, J. P., et al. (2004). GABAergic system gene expression predicts clinical outcome in patients with neuroblastoma. J. Clin. Oncol. 22, 4127–4134. doi: 10.1200/JCO.2004.02.032
Rodríguez-Hernández, C. J., Mateo-Lozano, S., García, M., Casalà, C., Briansó, F., Castrejón, N., et al. (2016). Cinacalcet inhibits neuroblastoma tumor growth and upregulates cancer-testis antigens. Oncotarget. doi: 10.18632/oncotarget.7448. [Epub ahead of print].
Rogers, K. V., Dunn, C. K., Hebert, S. C., and Brown, E. M. (1997). Localization of calcium receptor mRNA in the adult rat central nervous system by in situ hybridization. Brain Res. 744, 47–56. doi: 10.1016/S0006-8993(96)01070-0
Ruat, M., Molliver, M. E., Snowman, A. M., and Snyder, S. H. (1995). Calcium sensing receptor: molecular cloning in rat and localization to nerve terminals. Proc. Natl. Acad. Sci. U.S.A. 92, 3161–3165. doi: 10.1073/pnas.92.8.3161
Ruat, M., and Traiffort, E. (2013). Roles of the calcium sensing receptor in the central nervous system. Best. Pract. Res. Clin. Endocrinol. Metab. 27, 429–442. doi: 10.1016/j.beem.2013.03.001
Schwab, M., Alitalo, K., Klempnauer, K. H., Varmus, H. E., Bishop, J. M., Gilbert, F., et al. (1983). Amplified DNA with limited homology to myc cellular oncogene is shared by human neuroblastoma cell lines and a neuroblastoma tumour. Nature 305, 245–248. doi: 10.1038/305245a0
Scillitani, A., Guarnieri, V., Battista, C., De Geronimo, S., Muscarella, L. A., Chiodini, I., et al. (2007). Primary hyperparathyroidism and the presence of kidney stones are associated with different haplotypes of the calcium-sensing receptor. J. Clin. Endocrinol. Metab. 92, 277–283. doi: 10.1210/jc.2006-0857
Scillitani, A., Guarnieri, V., De Geronimo, S., Muscarella, L. A., Battista, C., D'Agruma, L., et al. (2004). Blood ionized calcium is associated with clustered polymorphisms in the carboxyl-terminal tail of the calcium-sensing receptor. J. Clin. Endocrinol. Metab. 89, 5634–5638. doi: 10.1210/jc.2004-0129
Scotting, P. J., Walker, D. A., and Perilongo, G. (2005). Childhood solid tumours: a developmental disorder. Nat. Rev. Cancer 5, 481–488. doi: 10.1038/nrc1633
Shimada, H., Ambros, I. M., Dehner, L. P., Hata, J., Joshi, V. V., Roald, B., et al. (1999). The International Neuroblastoma Pathology Classification (the Shimada system). Cancer 86, 364–372.
Shimada, H., Umehara, S., Monobe, Y., Hachitanda, Y., Nakagawa, A., Goto, S., et al. (2001). International neuroblastoma pathology classification for prognostic evaluation of patients with peripheral neuroblastic tumors: a report from the Children's Cancer Group. Cancer 92, 2451–2461. doi: 10.1002/1097-0142(20011101)92:9<2451::AID-CNCR1595>3.0.CO;2-S
Tharmalingam, S., Wu, C., and Hampson, D. R. (2016). The calcium-sensing receptor and integrins modulate cerebellar granule cell precursor differentiation and migration. Dev. Neurobiol. 76, 375–389. doi: 10.1002/dneu.22321
Timp, W., and Feinberg, A. P. (2013). Cancer as a dysregulated epigenome allowing cellular growth advantage at the expense of the host. Nat. Rev. Genet. 13, 497–510. doi: 10.1038/nrc3486
van Noesel, M. M. (2012). Neuroblastoma stage 4S: a multifocal stem-cell disease of the developing neural crest. Lancet Oncol. 13, 229–230. doi: 10.1016/S1470-2045(12)70012-8
Vezzoli, G., Terranegra, A., Arcidiacono, T., Biasion, R., Coviello, D., Syren, M. L., et al. (2007). R990G polymorphism of calcium-sensing receptor does produce a gain-of-function and predispose to primary hypercalciuria. Kidney Int. 71, 1155–1162. doi: 10.1038/sj.ki.5002156
Vizard, T. N., O'Keeffe, G. W., Gutierrez, H., Kos, C. H., Riccardi, D., and Davies, A. M. (2008). Regulation of axonal and dendritic growth by the extracellular calcium-sensing receptor. Nat. Neurosci. 11, 285–291. doi: 10.1038/nn2044
Yun, F. H., Wong, B. Y., Chase, M., Shuen, A. Y., Canaff, L., Thongthai, K., et al. (2007). Genetic variation at the calcium-sensing receptor (CASR) locus: implications for clinical molecular diagnostics. Clin. Biochem. 40, 551–551. doi: 10.1016/j.clinbiochem.2006.12.011
Keywords: development, nervous system, neuroblastoma, calcium-sensing receptor, differentiation, differentiation and proliferation
Citation: Mateo-Lozano S, García M, Rodríguez-Hernández CJ and de Torres C (2016) Regulation of Differentiation by Calcium-Sensing Receptor in Normal and Tumoral Developing Nervous System. Front. Physiol. 7:169. doi: 10.3389/fphys.2016.00169
Received: 25 January 2016; Accepted: 25 April 2016;
Published: 10 May 2016.
Edited by:
Enikö Kallay, Medical University of Vienna, AustriaCopyright © 2016 Mateo-Lozano, García, Rodríguez-Hernández and de Torres. This is an open-access article distributed under the terms of the Creative Commons Attribution License (CC BY). The use, distribution or reproduction in other forums is permitted, provided the original author(s) or licensor are credited and that the original publication in this journal is cited, in accordance with accepted academic practice. No use, distribution or reproduction is permitted which does not comply with these terms.
*Correspondence: Carmen de Torres, cdetorres@hsjdbcn.org