- Plant and Soil Science Section, Department of Agriculture and Ecology, Faculty of Life Sciences, University of Copenhagen, Frederiksberg C, Copenhagen, Denmark
The occurrence of manganese (Mn) deficiency in cereal crops has increased in recent years. This coincides with increasing phosphorus (P) status of many soils due to application of high levels of animal manure and P-fertilizers. In order to test the hypothesis that elevated P my lead to Mn deficiency we have here conducted a series of hydroponics and soil experiments examining how the P supply affects the Mn nutrition of barley. Evidence for a direct negative interaction between P and Mn during root uptake was obtained by on-line inductively coupled plasma mass spectrometry (ICP-MS). Addition of a pulse of KH2PO4 rapidly and significantly reduced root Mn uptake, while a similar concentration of KCl had no effect. Addition of a P pulse to the same nutrient solution without plants did not affect the concentration of Mn, revealing that no precipitation of Mn–P species was occurring. Barley plants growing at a high P supply in hydroponics with continuous replenishment of Mn2+ had up to 50% lower Mn concentration in the youngest leaves than P limited plants. This P-induced depression of foliar Mn accelerated the development of Mn deficiency as evidenced by a marked change in the fluorescence induction kinetics of chlorophyll a. Also plants growing in soil exhibited lower leaf Mn concentrations in response to elevated P. In contrast, leaf concentrations of Fe, Cu, and N increased with the P supply, supporting that the negative effect of P on Mn acquisition was specific rather than due to a general dilution effect. It is concluded that elevated P supply directly interferes with Mn uptake in barley roots and that this negative interaction can induce Mn deficiency in the shoot. This finding has major implications in commercial plant production where many soils have high P levels.
Introduction
Phosphorus (P) and manganese (Mn) are essential plant nutrients. Phosphorus is a constituent of nucleic acids, phospholipids and ATP, and is also involved in the regulation of key metabolic pathways (Yang and Finnegan, 2010; Byrne et al., 2011; Yao et al., 2011). Manganese (Mn) primarily acts as an activator of many enzymes and may in many cases be substituted by other nutrients, especially magnesium. However, in at least three cases Mn is irreplaceable (Burnell, 1988; Hansch and Mendel, 2009). These include (i) oxalate oxidase, catalyzing the conversion of oxalate and oxygen to hydrogen peroxide and carbon dioxide (Requena and Bornemann, 1999); (ii) superoxide dismutase (MnSOD), converting the oxygen free radical O2− to H2O2 and O2 (Alscher et al., 2002; Poage et al., 2011); and (iii) the oxygen evolving complex (OEC) of photosystem II, placed in the thylakoid membranes of the chloroplasts and containing Mn bound in a 4-atom cluster (Ono et al., 1992).
Manganese deficiency is regularly observed in plants growing on soils with low Mn availability, but the frequency of the problem has increased during recent years (Hebbern et al., 2005). During the same period, soil-P levels have increased due to high application of animal manure and P-fertilizers (Kyllingsbæk and Hansen, 2007; Reijneveld et al., 2010; MacDonald et al., 2011). It can therefore be hypothesized that the increased frequency of Mn deficiency may be influenced by the increased soil-P levels. Both P and Mn ions are rather immobile in soils implying that factors such as root length and root architecture, together with rhizosphere processes, have a major impact on their plant availability (Gahoonia and Nielsen, 1997; Gherardi and Rengel, 2004; Raghothama and Karthikeyan, 2005). The presence of Mn substantially inhibited root hair elongation in Arabidopsis, whereas Mn-deficient seedlings displayed stimulated root hair development (Yang et al., 2008). Also P starvation increases the number of lateral roots and root hair abundance (Gahoonia et al., 1997; Zhu et al., 2010; Miura et al., 2011). Simultaneously, nitrate uptake is inhibited by P starvation leading to excess uptake of cations relative to anions and acidification of the rhizosphere (Hedley et al., 1982; Schjoerring, 1986; Vegh et al., 2009). The absence of these adaptive root responses in soils with high P status will reduce the ability of roots to acquire Mn.
Phosphorus interacts with other micronutrients than Mn, e.g., zinc (Zn), iron (Fe), and copper (Cu; Murphy et al., 1981; Broadley et al., 2010; Perez-Novo et al., 2011). Phosphate fertilization of plants with low Zn status may lead to visible Zn deficiency symptoms, a phenomenon referred to as P-induced Zn deficiency (Cakmak and Marschner, 1987). It is not clear whether decreased Zn uptake or dilution following increased biomass production are the primary reason for P-induced Zn deficiency (Singh et al., 1988; Gianquinto et al., 2000; Zhu et al., 2001; Li et al., 2003). Genes en-coding high-affinity orthophosphate transporters are up-regulated under Zn deficiency (Huang et al., 2000) resulting in higher P uptake, potentially accompanied by P toxicity which can be alleviated by addition of Zn (Marschner and Cakmak, 1986; Silber et al., 2002).
A few cases of negative influence of high soil-P availability on Mn uptake have been reported, tentatively assigned to the reduced formation of cluster roots, root-induced changes in the rhizosphere or negative interactions with P during translocation from roots to shoots (Neilsen et al., 1992; Zhu et al., 2002; Shane and Lambers, 2005). However, the exact mechanisms behind the Mn–P interaction are still unknown.
The objective of the present study was to examine interactions between P and Mn in barley plants. A series of experiments were conducted with plants exposed to different Mn and P treatments in soil or hydroponics. The results show a negative effect of high P levels on Mn accumulation and it is suggested that P interferes directly with Mn at the uptake and/or translocation level.
Materials and Methods
Plant Cultivation
Hydroponic experiments
Seeds of the Mn-efficient genotype Vanessa and the inefficient genotype Antonia were geminated at 21°C in vermiculite. After 4 days, uniform seedlings were selected and transferred to opaque 4 l cultivation units, each holding four plants. The units were filled with aerated nutrient solution having the following composition: 0.2 mM KH2PO4, 0.2 mM K2SO4, 0.3 mM MgSO4·7H2O, 0.1 mM NaCl, 0.3 mM Mg(NO3)2·6H2O, 0.9 mM Ca(NO3)2·4H2O, 0.6 mM KNO3, 0.8 μM Na2MoO4·2H2O, 0.7 μM ZnCl2, 0.8 μM CuSO4·5H2O, 2 μM H2BO3, 1 μM NiSO4·6H2O, 50 μM Fe(NO3)3·9H2O, and 10 mM EDTA. Before use, the macronutrient stock solutions were purified with chelex-100 resin (Sigma-Aldrich) in order to remove multivalent cations (Pedas et al., 2005). During the first week all plants were supplied with medium levels of P, Mn, and other nutrients. Thereafter P and Mn were applied on a daily basis in three contrasting levels (Table 1), while all other nutrients were applied once a week simultaneously with renewal of the nutrient solution and re-randomization of the cultivation units. Phosphorus was added in the form of KH2PO4 and extra K was supplied in the form of 0, 400, and 490 μM K2SO4 to the low, medium and high P treatment, respectively, in order to obtain similar K levels for all treatments. The pH was adjusted daily with 1 M HCl. The plants were placed in the greenhouse at 18/15°C day/night temperature and 16 h day/8 h night cycle. The photon flux density was 250–300 μmol m−2 s−1. Plants were harvested after 33 days of growth when they had attained growth stage 32 (Zadoks et al., 1974). The roots were washed in double deionised water for 5 min, followed by drying of shoots and roots.
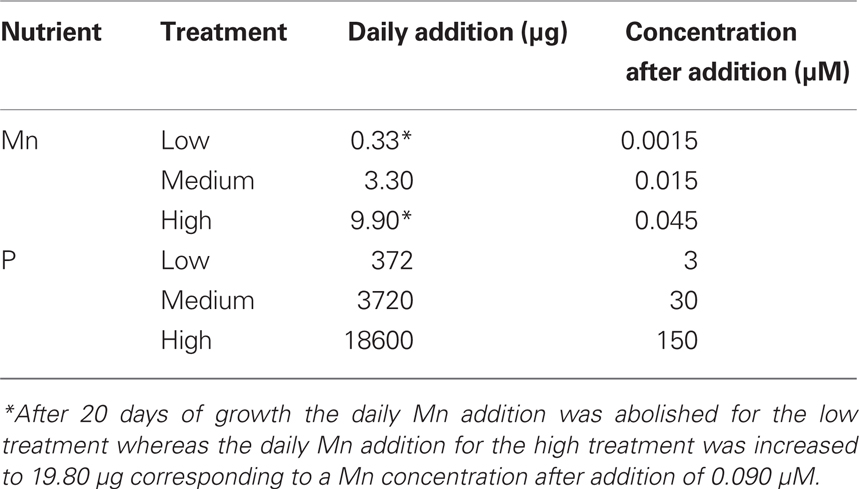
Table 1. Daily addition rates of phosphorus and manganese per cultivation unit with four plants in the hydroponic experiment.
Soil experiments
Soil was collected in a field cropped with cereals for more than 40 years without application of any organic or inorganic P or K fertilizers (Schjoerring and Nielsen, 1987). The NaHCO3-extractable P content of the soil was 10.8 mg P kg−1 dry soil and the pH 5.1 (0.01 M CaCl2). The soil was mixed with quartz sand and sphagnum (2:3:1 v/v) and 0.3 g N kg−1 mixed soil added in the form of NK-fertilizer with S, Mg, and B (Kemira 19-0-15; 50% nitrate-N, 50% ammonium-N). The pH (0.01 M CaCl2) of the mixed soil was adjusted to 6.0 by mixing the soil with 1.25 g CaCO3 kg−1 soil. Four P levels were produced by addition of 0, 13.5, 41.4, or 83.2 mg KH2PO4 kg−1 soil. In order to achieve similar K levels, K2SO4 was also added (587.3, 549.4, 470.9, or 353.2 mg K2SO4 kg−1 soil). All pots were watered with Milli-Q-water to field capacity and were left standing for 48 h before planting.
Seeds of barley (Hordeum vulgare L. cv. Antonia) were geminated at 21°C in vermiculite. After 4 days, uniform seedlings were selected and transferred to 2.5 l pots with soil. The pots each held four plants and were placed in trays allowing watering from below. The plants were grown in greenhouse with 25/18°C (16/8 h) day/night temperature regime and 250–300 μmol m−2 s−1 photon flux density. Plants were harvested after 50 days of growth (growth stage 37; Zadoks et al., 1974) and the dry weight of the different plant organs was determined.
Plant Analysis
Chlorophyll a fluorescence
The effect of Mn deficiency on PSII was recorded by measurement of the fluorescence induction kinetics of chlorophyll a using a Handy PEA (Plant Efficiency Analyzer, Hansatech Instruments, King’s Lynn, UK). A saturating light flash of 3000 μmol photons m−2 s−1 was applied for 2 s to leaves which had been dark adapted for 25 min. The fluorescence results were parameterized to Fv/Fm using the Handy PEA software (version 1.30).
On-line measurements of nutrient uptake by inductively coupled plasma mass spectrometry
Plants were pre-grown as described above for 25–53 days depending on the availability of the ICP-MS instrument. Starting at least 7 days before the experiments, the nutrient solution was exchanged daily. In addition, 24 h before the experiments, plants were pre-equilibrated to the uptake solution containing a reduced micronutrient supply composed of 0.01 μM Na2MoO4·2H2O, 0.01 μM ZnCl2, 0.01 μM CuSO4·5H2O, 0.01 μM H2BO3, 0.01 μM NiSO4·6H2O, 0.01 μM Fe(NO3)3·9H2O, and 100 nM MnCl2. This procedure was necessary in order to reduce the effects of ion-exchange processes between root and nutrient solution.
The experiments were conducted with plants growing in 300 ml vials (Bie & Berntsen, Denmark) and exposed to a 250 to 280-μmol m−2 s−1 photon flux density and 20°C (16/8 h) day/night temperature regime. Each series of measurements lasted up to 8 h and embraced one vial with one plant and one without plant (control). Each of the two vials was analyzed every 12 min during the experimental period. Pulses of 150 or 500 μM KH2PO4, or 200 μM KCl, were added at specified time points and the rate of P and Mn depletion from the nutrient solution were recorded by on-line ICP-MS (Agilent 7500c, Agilent Technologies, Manchester, UK). A multi-channel peristaltic pump continuously recirculated nutrient solution between an auto sampler vial (50 ml vial, Product no. 02-6100N, CM-LAB, Denmark) and the cultivation unit with plants. The tube inlet and outlet were vertically displaced in the auto sampler tube providing a steady-state volume of approx. 7.5 ml due to a flow rate difference between inlet and outlet. During sampling, a mixture of 1.75% HNO3, 0.2% HF, and 10 mg l−1 45Sc was continuously added to the nebulizer (split-ratio 1:20) in order to reduce memory effects and to correct for drift and argon plasma instability. In separate experiments, continuous analysis of control nutrient solution without plants documented that no significant interferences caused by, e.g., precipitation and adsorption of Mn to surfaces of the experimental system were occurring during the experiments (Pedas et al., 2005).
Nutrient composition of plant tissue
Following freeze drying (Christ Alpha 2–4; Martin Christ GmbH), milling, and digestion, leaf and root samples were analyzed by ICP-MS as previously described (Hansen et al., 2009). For every 40 samples, four blanks without plant material and four with certified reference material (apple leaf, standard reference material 1515; US Department of Commerce, National Institute of Standards and Technology, Gaithersburg, MD, USA) were included. For total-N, determination 4 mg of dried plant material was weighed into tin capsules and analyzed in a system consisting of an ANCA-SL Elemental Analyzer coupled to a 20–20 Tracermass Mass Spectrometer (Europa Scientific Ltd., Crewe, UK).
Root hair density
Representative root samples in terms of size, root geometry, and thickness were analyzed with respect to root hair density using a scanner (ScanJet IIcx) and DT-Scan Software (Delta-T Devices, Cambridge, England) as described by Gahoonia et al. (2000).
Results
Interactions between Mn and P during Uptake by Roots
The influence of P on Mn acquisition was assessed in a series of on-line uptake experiments using ICP-MS. The experiments were carried out with the Mn-efficient genotype Vanessa growing at Mn concentrations <70 nM, i.e., mimicking the low Mn concentration in the rhizosphere soil solution of well-aerated agricultural soils. All plants were at the time of the experiments in a healthy nutritional state with Fv/Fm values of approximately 0.8, close to the theoretical optimum of 0.83 (Kriedemann et al., 1985). Prior to the addition of Mn/P pulses, plant roots had been exposed to the uptake solution for 24 h which resulted in Mn and P concentrations below 10 nM and 1 μM, respectively.
Plant roots receiving a Mn pulse in the absence of a simultaneous P pulse steadily depleted the nutrient solution for Mn (Figure 1). However, when the Mn pulse was accompanied by addition of 150 μM KH2PO4, the Mn uptake rate over the first h after the pulse decreased substantially from 7.8 to 1.7 nmol g−1 DW h−1 (Figure 1). Addition of a similar P pulse to the same nutrient solution without plants did not affect the concentration of Mn, revealing that no precipitation of Mn–P species was occurring (data not shown). Calculations using Geochem-EZ (version 1.0) showed that no uncharged Mn–P complexes, e.g., , were likely to form under the condition given and that >99.5% of the Mn was expected to be present as the free Mn2+ ion (data not shown).
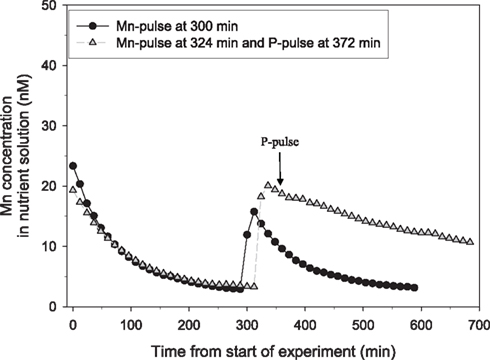
Figure 1. The direct effect of addition of a pulse of 150 μM P on the net uptake rate of Mn in 25-day-old plants of the Mn-efficient genotype Vanessa as measured by on-line ICP-MS. The experiment was repeated several times with similar results. The shoot and root weight was 0.48 and 0.45 g DW for the plant receiving only the Mn pulse (circles) and 0.48 and 0.53 g DW for the plant receiving an additional P pulse (triangles). The curves were normalized to equal root weight for both plants.
Tripling the P pulse to approximately 500 μM KH2PO4 completely inhibited the Mn uptake (Figure 2). Application of a similar P pulse, i.e., 500 μM KH2PO4, to only half of the roots in a split-root system instantaneously stopped the Mn uptake in roots exposed to extra P, while that in the other part of the root system was not affected (Figure 3). Approximately 2 h after addition of the P pulse, the Mn uptake resumed (Figure 3). When the KH2PO4 pulse was replaced with 200 μM KCl, no detectable decline in the Mn uptake rate occurred showing that the recorded effect was specific for P (Figure 4).
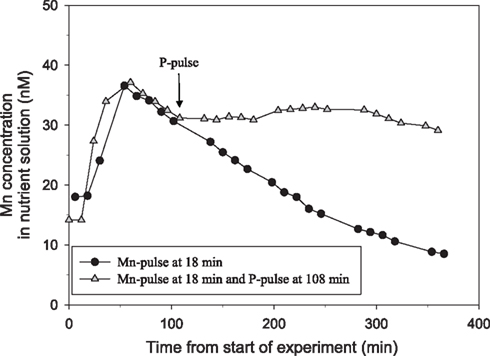
Figure 2. The direct effect of addition of a pulse of 500 μM P on Mn net uptake in 44-day-old plants of the Mn-efficient genotype Vanessa as measured by on-line ICP-MS. The experiment was repeated several times with similar results. The shoot and root weight was 4.78 and 1.7 g DW, for the plant receiving only the Mn pulse (circles) and 4.84 and 1.8 g DW for the plant receiving an additional P pulse (triangles). The curves were normalized to equal root weight for both plants.
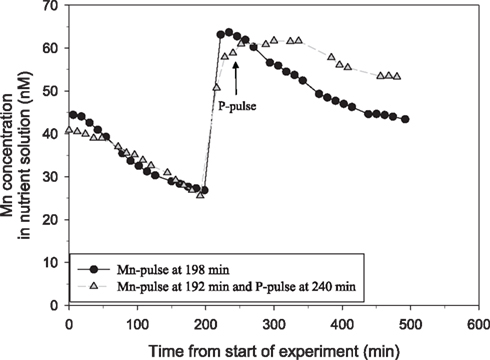
Figure 3. Split-root experiment analyzing the root specific effect of addition of a pulse of 500 μM P on Mn net uptake in a 53-day-old plant of the Mn-efficient genotype Vanessa as measured by on-line ICP-MS. Both root compartments were given a Mn pulse, followed by addition of a 500-μM P pulse to only one of the compartments. The experiment was repeated several times with similar results. The shoot weight was 6.93 g DW. The root weight was 1.36 g DW for the compartment pulsed with Mn only (circles) and 0.71 g DW of the other compartment receiving additional P. The two curves were normalized to equal root weight.
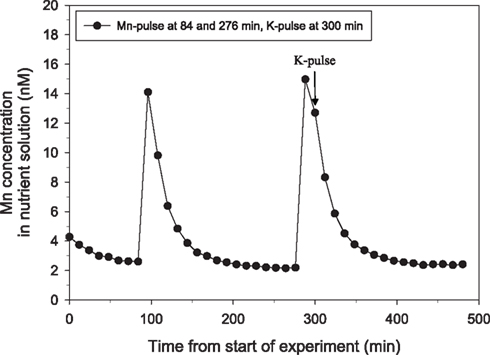
Figure 4. The direct effect of addition of a pulse of 200 μM K on Mn net uptake in 25-day-old plants of the Mn-efficient genotype Vanessa as measured by on-line ICP-MS. Two Mn pulses were given, the second followed by an additional pulse of 200 μM KCl (triangles). The experiment was repeated several times with similar results. The shoot and root weight were 4.51 and 2.90 g DW, respectively.
Plant Growth and Elemental Composition as Affected by Mn–P Interactions
No visual deficiency symptoms were observed in any of the treatments (data not shown). The Mn treatments did not result in any clear differences in biomass production in the hydroponic experiments (Table 2). This was the case even though plants growing at the low Mn level became clearly Mn deficient as evidenced by a decline in the Fv/Fm value to below 0.6 (Figure 5). Biomass production in the medium P treatment was significantly higher than that in the low P treatment and the root/shoot ratio decreased about 50% with increasing P supply showing preferential shoot growth in response to extra P (Table 2). In both the Mn-inefficient genotype Antonia and the efficient genotype Vanessa, Fv/Fm values for plants growing at low Mn supply started to decrease earlier and declined more pronouncedly at the medium and high P levels compared to the low P level thus showing a significant negative interaction between P and Mn (P < 0.05; Figures 5B,D). The Mn-efficient genotype Vanessa produced at all combinations of P and Mn more biomass (up to 50%) than the inefficient genotype Antonia (Table 2).
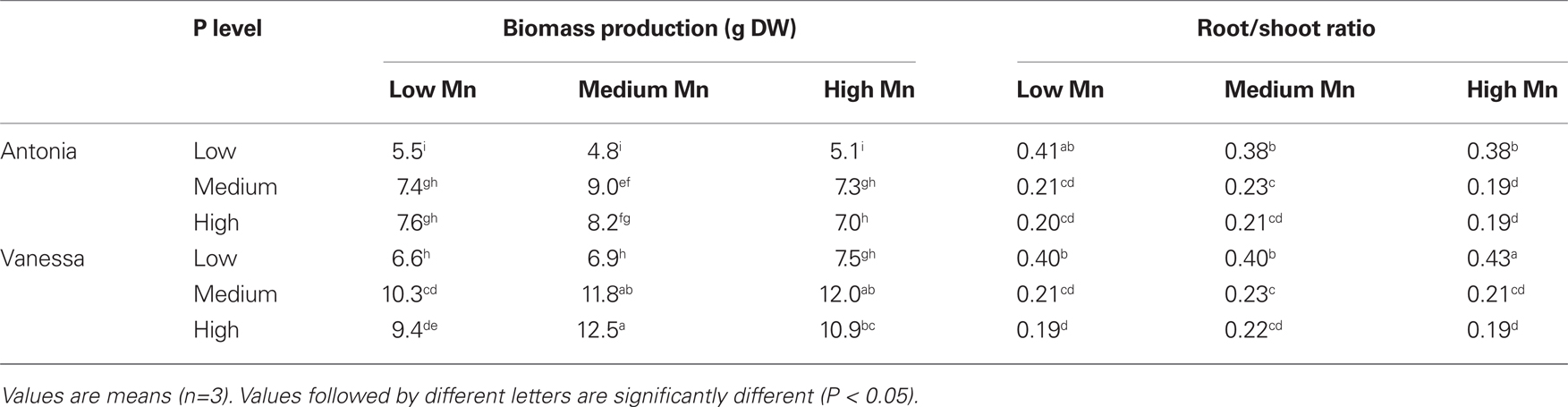
Table 2. Total biomass (shoot + root) and root/shoot ratios of barley genotypes Antonia and Vanessa grown in a hydroponic system at different Mn and P levels.
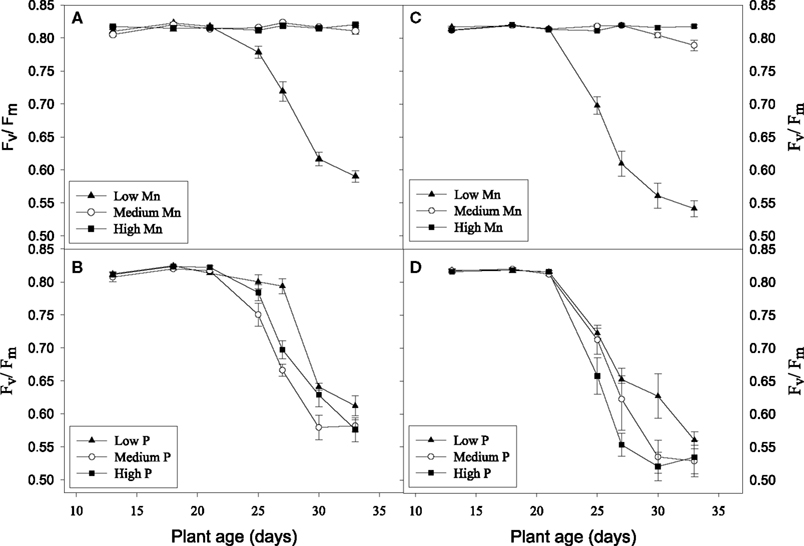
Figure 5. Chlorophyll a fluorescence measurements of hydroponically grown plants receiving different Mn and P levels. Fv/Fm values are shown for the Mn-inefficient genotype Antonia (A,B) and the efficient genotype Vanessa (C,D). Inserts (A) and (C) show Fv/Fm values depending on the Mn level summarized across the P levels. Inserts (B) and (D) show Fv/Fm values for plants grown at low Mn supply and increasing P additions. Each data point represents the mean ± SE (n = 6–18).
The elemental composition of the fully expanded youngest leaf was analyzed in order to reveal the influence of the interactions between P and Mn on the nutrient supply to growing tissues. Plants growing in hydroponics with frequent replenishment of Mn were used to avoid indirect dilution effects associated with formation of depletion zones in the rhizosphere soil when biomass production increases in response to elevated P. The P concentration in the youngest leaf increased significantly (P < 0.05) with the P level in the root medium (Figures 6C,F). In the low Mn treatment, the Mn concentration in the youngest leaf decreased below 15 μg g−1 at low P supply and further down below 10 μg g−1 at medium and high P supply (Figures 6A,D). Overall, the Mn concentration in the youngest leaf was in both genotypes negatively correlated with the P supply (P < 0.05). Conversely, the Fe and Cu concentrations in the youngest leaf were only marginally affected by increasing P supply (Figure A1 in Appendix), while the corresponding N concentration increased (Figure A2 in Appendix), supporting that the negative interaction between Mn and P concentrations was specific rather than due to a general dilution effect. The Zn concentration in the youngest leaf decreased with increasing P supply as was the case for Mn (Figures 6B,E). At low P supply, the Zn concentration in the youngest leaf was up to 70% higher than at medium and high P supply. The negative effect of P supply on the Zn concentration was most pronounced at low Mn supply and almost disappeared at ample Mn supply (Figures 6B,E).
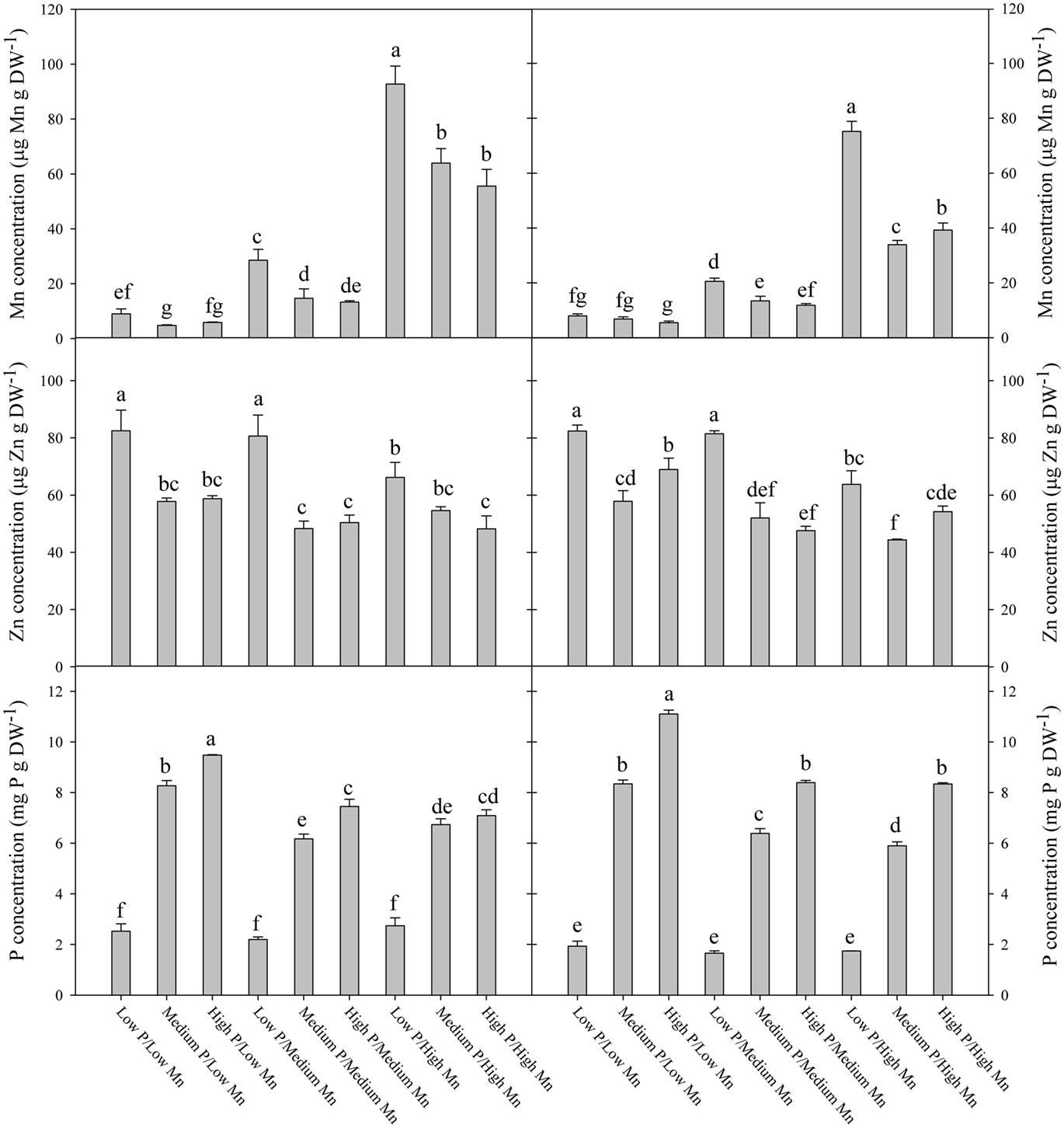
Figure 6. The concentration of Mn (A,D), Zn (B,E), and P (C,F) in the youngest fully emerged leaf of the Mn-inefficient genotype Antonia (A–C) and Mn-efficient genotype Vanessa (D–F) growing in hydroponics at different Mn and P levels. Values are means ± SE (n = 3); same letter above columns denote no significant differences between treatments (p > 0.05).
Increasing P supply to soil-grown plants of the genotype Antonia resulted in more than two-fold increase in shoot weights (Figure 7A) and three-fold increase in foliar P concentrations (Figure 8B). Conversely, root hair density decreased 35% (Figure 7B). Leaf Zn and Mn concentrations were negatively affected by increasing P supply, decreasing 37 and 29%, respectively, while the Fe and Cu concentrations increased 42 and 71%, respectively (Figure 8A).
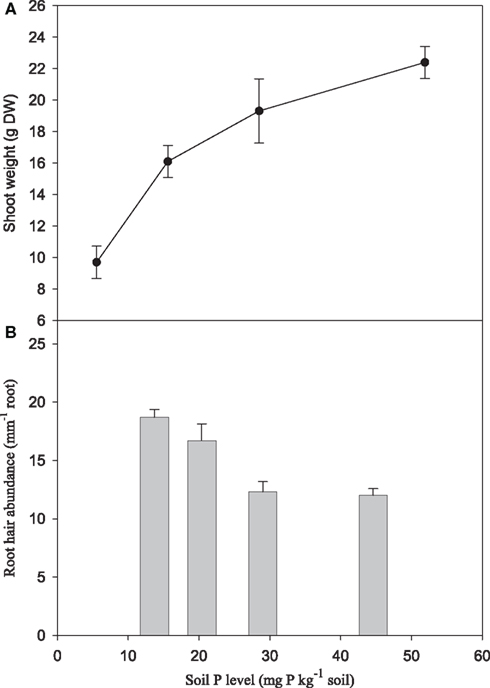
Figure 7. Shoot dry matter yield (A) and root hair abundance (B) for the Mn-inefficient genotype Antonia in response to increasing soil-P levels. Values are means ± SE (n = 3).
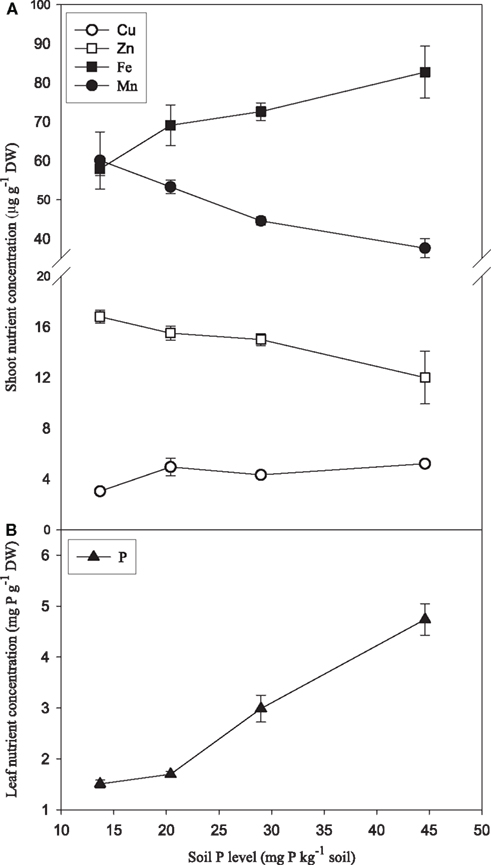
Figure 8. Leaf concentrations of Mn, Zn, Fe, Cu (A), and P (B) in plants of the Mn-inefficient genotype Antonia growing in soil with increasing P levels. Values are means ± SE (n = 3).
Discussion
Previous studies of interactions between Mn and P have been carried out with plants growing in soil (Neilsen et al., 1992; Shane and Lambers, 2005). In the present study we primarily used a hydroponic growth system to examine how interactions between Mn and P affect growth, Mn uptake, and nutrient concentrations in root and leaf tissues. One of the advantages associated with nutrient solutions instead of soil is that effects of P addition on Mn uptake by the roots can be measured dynamically. Using a very sensitive on-line ICP-MS system, enabling analysis in the nM range, we have here demonstrated that Mn uptake respond instantaneously and specifically to changes in the external P concentration (Figures 1–4). The decline in Mn uptake appeared to increase with the P concentration, ranging from 75% inhibition at 150 μM P (Figure 1) to complete abolishment following addition of 500 μM P (Figure 2). Addition of a similar P pulse to the same nutrient solution without plants did not affect the concentration of Mn, revealing that precipitation of Mn–P species were not occurring (data not shown). It has been hypothesized that Mn2+ may form an ion-par with HPO42− resulting in the uncharged soluble complex (Lindsay, 1979). Using Geochem-EZ (version 1.0) we therefore examined to which extent inorganic soluble Mn–P complexes were likely to form after addition of P. According to the Geochem calculations, no uncharged Mn–P complexes were likely to form and >99.5% of the Mn was present as the free Mn2+ ion (data not shown). The observed decline in Mn uptake following application of a P pulse is therefore unlikely to reflect changes in Mn speciation but rather occurred due to a direct interaction between Mn2+ and H2PO4− during Mn uptake.
Plants acquire Pi by active uptake into the epidermal and cortical cells of the root via proton–Pi symporters of the PHT1 family (Raghothama, 2000). The high-affinity barley Pi transporter HvPHT1;1 is highly expressed in root segments with dense root hairs, is localized to the plasma membrane and shows preferential selectivity for Pi relative to SO42− and NO3− (Preuss et al., 2011). Uptake of Pi via this transporter leads to a depolarization of the membrane potential (Preuss et al., 2011) which hypothetically may affect Mn2+ uptake. In addition, the accompanying proton uptake may lead to a transient alkalinisation of the apoplastic solution around the root cells which potentially can promote Mn2+ adsorption to negative charges in the cell walls and limit the concentration of Mn2+ at the uptake sites.
The external P concentration may affect different ion transporters via phosphorylation/dephosphorylation reactions (Bayle et al., 2011). In yeast, protein phosphatase 2B has been shown to be essential for prevention of Mn entry into the cells (Farcasanu et al., 1995). Transporter gene families that have been implicated in Mn uptake by plant roots include natural resistance-associated macrophage protein (Nramp) transporters and zinc-regulated transporter/iron-regulated transporter (ZRT/IRT1)-related protein (ZIP) transporters (Pittman, 2005; Pedas et al., 2008; Cailliatte et al., 2010). None of these are presently known to interact with P. However, an alternative pathway for Mn uptake was identified in yeast where the high-affinity H+-Pi co-transporter Pho84 was shown also to mediate low-affinity Mn transport (Jensen et al., 2003). Barley and Arabidopsis have various P transporters with a significant similarity to Pho84 (Leggewie et al., 1997). The Arabidopsis Pho1 transporter shares extensive amino acid homology with the yeast Pho84 transporter and is involved in xylem loading and long-distance transfer of Pi from the root to the shoot (Hamburger et al., 2002). Overexpression of PHO1 in Arabidopsis leaves has recently been shown to lead to a dramatic efflux of Pi out of cells and into the xylem vessel, revealing a crucial role for PHO1 in Pi efflux (Stefanovic et al., 2011). A potential link between phosphate efflux and Mn acquisition remains to be investigated.
A split-root experiment showed that the initial rapid decline in Mn uptake in response to P addition only occurred in roots exposed to the P pulse, while the other root part continued to absorb Mn with the same rate as before (Figure 3). Thus, systemic feed-back signals in the root system, or between roots and shoots, were not responsible for the Mn–P interaction. However, after approximately 2 h, the Mn uptake started to recover again in the roots exposed to the P pulse (Figure 3). This gradual acclimation may involve long-distance signaling affecting P acquisition and translocation between roots and shoots (Schachtman and Shin, 2007; Liu et al., 2009). MicroRNAs play an important role in the regulation of stress responses in plants and some miRNAs respond both to changes in P as well as Mn availability (Valdés-López et al., 2010).
Physiological Effects of Mn–P Interactions
Measurements of fluorescence induction kinetics of chlorophyll a demonstrated that the low Mn treatment induced a decline in Fv/Fm values (Figure 5), showing that the plants were suffering from Mn deficiency although no visual symptoms were present (Hebbern et al., 2009; Husted et al., 2009). The decline in Fv/Fm values for plants growing at low Mn levels was dependent on the supply of P, occurring faster for plants growing at medium or high P levels compared to low P (Figures 5B,D). Ample P supply thus reduced Mn acquisition, translocation and/or the amount of Mn functionally available for photosynthesis. The ability to perform state transitions and the amount of the photosynthetic key protein PsbA (D1 protein) have previously been shown to be influenced by the plant Mn status (Husted et al., 2009). The Mn concentration in the youngest leaf of both genotypes growing in the low Mn treatment was approximately 12 μg Mn g DW−1 (Figures 6A,D) which is below the critical level for Mn (Reuter et al., 1997). The elemental analysis of the plant tissue thus confirmed the Chl a fluorescence measurements and both approaches documented the risk of inducing Mn deficiency in the youngest leaf by increasing P levels.
The Mn concentration in plants results from the integration of two dynamic processes, dry matter accumulation and Mn absorption. If dry matter accumulation increases at a faster rate than Mn absorption it will result in a decrease in the Mn concentration. This inverse relationship between growth and mineral concentration is termed the dilution effect (Jarrell and Beverly, 1981). The Mn concentration in the youngest leaf was clearly negatively affected by increasing P supplies (Figures 6A,D). However, for the following reasons we consider it unlikely that this decrease was due to a general dilution effect: Firstly, the plants were grown in hydroponics with continuous replenishment of Mn, ensuring that the availability of Mn to the roots was the same throughout the experiment. This is in contrast to soil, where increased biomass production in response to P application during early growth stages may enhance Mn depletion in the rhizosphere and reduce the amount of Mn available for uptake and translocation to the youngest leaf, thereby leading to dilution of its Mn concentration. Secondly, the fact that the weight of the youngest leaf was not affected by the P treatments (data not shown) supports that the decline in Mn concentration reflected a negative effect of P on the actual Mn import rather than increased growth. Thirdly, the Fe and Cu concentrations did not decrease, but were only marginally affected by increasing P supply (Figure A1 in Appendix), as was also the case for N (Figure A2 in Appendix), thus contradicting a general dilution effect. Also barley plants growing in soil showed decreased Mn and Zn concentrations in the leaves in response to P supply, while Cu and Fe increased (Figure 8). Reduced foliar Mn concentrations under high soil-P availability are in accordance with previous experiments (Neilsen et al., 1992; Shane and Lambers, 2005) who similarly attributed this to physiological interactions. In a sand-culture experiment with eight barley cultivars, Zhu et al. (2002) showed that increased P availability reduced Zn shoot concentration in all cultivars, but only Mn concentrations in some of the cultivars. In the present work, there were no clear differences between the Mn-inefficient genotype Antonia and the Mn-efficient genotype Vanessa in their response to elevated P, neither in terms of fluorescence induction kinetics of chlorophyll a (Figure 5) nor in the Mn concentration in the youngest fully emerged leaf (Figure 6). This was the case although Vanessa at all combinations of P and Mn produced up to 50% more biomass than Antonia (Table 2).
Conclusion
We have here shown that elevated P supply directly interferes with Mn uptake in barley roots and that this negative interaction can induce Mn deficiency in the shoot. The practical implication of this is that increased soil-P levels may be expected to lead to increased prevalence of Mn deficiency in barley crops.
Conflict of Interest Statement
The authors declare that the research was conducted in the absence of any commercial or financial relationships that could be construed as a potential conflict of interest.
Acknowledgments
We thank Karl Martin Schelde for assistance with plant cultivation and harvest. Funding from The Danish Strategic Research Council (grant 10-093498 NUTRIEFFICIENT), the Hedvig and Johannes Sørensen Foundation, the Hede Nielsen Family Foundation, the Ellen, Christian and Anders Petersen Foundation, the Petersen Fruervadgård Family Foundation, and The Ministry of Science, Technology, and Innovation (grant no 274-06-0325) are gratefully acknowledged.
References
Alscher, R. G., Erturk, N., and Heath, L. S. (2002). Role of superoxide dismutases (SODs) in controlling oxidative stress in plants. J. Exp. Bot. 53, 1331–1341.
Bayle, V., Arrighi, J. F., Creff, A., Nespoulous, C., Vialaret, J., Rossignol, M., Gonzalez, E., Paz-Ares, J., and Nussaume, L. (2011). Arabidopsis thaliana high-affinity phosphate transporters exhibit multiple levels of posttranslational regulation. Plant Cell 23, 1523–1535.
Broadley, M. R., Lochlainn, S. O., Hammond, J. P., Bowen, H. C., Cakmak, I., Eker, S., Erdem, H., King, G. J., and White, P. J. (2010). Shoot zinc (Zn) concentration varies widely within Brassica oleracea L. and is affected by soil Zn and phosphorus (P) levels. J. Hort. Sci. Biotechnol. 85, 375–380.
Burnell, J. N. (1988). “The biochemistry of manganese in plants,” in Manganese in Soils and Plants, eds R. D. Graham, R. J. Hannam, and N. C. Uren (Dordrecht: Kluwer Academic Publishers), 125–137.
Byrne, S. L., Foito, A., Hedley, P. E., Morris, J. A., Stewart, D., and Barth, S. (2011). Early response mechanisms of perennial ryegrass (Lolium perenne) to phosphorus deficiency. Ann. Bot. 107, 243–254.
Cailliatte, R., Schikora, A., Briat, J. F., Mari, S., and Curie, C. (2010). High-affinity manganese uptake by the metal transporter NRAMP1 is essential for Arabidopsis growth in low manganese conditions. Plant Cell 22, 904–917.
Cakmak, I., and Marschner, H. (1987). Mechanism of phosphorus-induced zinc deficiency in cotton. III Changes in physiological availability of zinc in plants. Physiol. Plant 70, 13–20.
Farcasanu, I. C., Hirata, D., Tsuchiya, E., Nishiyama, F., and Miyakawa, T. (1995). Protein phosphatase 2B of Saccharomyces cerevisiae is required for tolerance to manganese, in blocking the entry of ions into the cells. Eur. J. Biochem. 232, 712–717.
Gahoonia, T. S., Asmar, F., Giese, H., Gissel-Nielsen, G., and Nielsen, N. E. (2000). Root-released organic acids and phosphorus uptake of two barley cultivars in laboratory and field experiments. Eur. J. Agron. 12, 281–289.
Gahoonia, T. S., Care, D., and Nielsen, N. E. (1997). Root hairs and phosphorus acquisition of wheat and barley cultivars. Plant Soil 191, 181–188.
Gahoonia, T. S., and Nielsen, N. E. (1997). Variation in root hairs of barley cultivars doubled soil phosphorus uptake. Euphytica 98, 177–182.
Gherardi, M. J., and Rengel, Z. (2004). The effect of manganese supply on exudation of carboxylates by roots of lucerne (Medicago sativa). Plant Soil 260, 271–282.
Gianquinto, G., Abu-Rayyan, A., Tola, L. D., Piccotino, D., and Pezzarossa, B. (2000). Interaction effects of phosphorus and zinc on photosynthesis, growth and yield of dwarf bean grown in two environments. Plant Soil 220, 219–228.
Hamburger, D., Rezzonico, E., Petetot, J. M. C., Somerville, C., and Poirier, Y. (2002). Identification and characterization of the Arabidopsis PHO1 gene involved in phosphate loading to the xylem. Plant Cell 14, 889–902.
Hansch, R., and Mendel, R. R. (2009). Physiological functions of mineral micronutrients (Cu, Zn, Mn, Fe, Ni, Mo, B, Cl). Curr. Opin. Plant Biol. 12, 259–266.
Hansen, T. H., Laursen, K. H., Persson, D. P., Pedas, P., Husted, S., and Schjoerring, J. K. (2009). Micro-scaled high-throughput digestion of plant tissue samples for multi-elemental analysis. Plant Methods 5, 12.
Hebbern, C. A., Laursen, K. H., Ladegaard, A. H., Schmidt, S. B., Pedas, P., Bruhn, D., Schjoerring, J. K., Wulfsohn, D., and Husted, S. (2009). Latent manganese deficiency increases transpiration in barley (Hordeum vulgare). Physiol. Plant 135, 307–316.
Hebbern, C. A., Pedas, P., Schjoerring, J. K., Knudsen, L., and Husted, S. (2005). Genotypic differences in manganese efficiency: a field trial with winter barley (Hordeum vulgare L.). Plant Soil 272, 233–244.
Hedley, M. J., Nye, P. H., and White, R. E. (1982). Plant-induced changes in the rhizosphere of rape seedlings. 2. Origin of the pH change. New Phytol. 91, 31–44.
Huang, C. Y., Barker, S. J., Langridge, P., Smith, F. W., and Graham, R. D. (2000). Zinc deficiency up-regulates expression of high-affinity phosphate transporter genes in both phosphate-sufficient and -deficient barley roots. Plant Physiol. 124, 415–422.
Husted, S., Laursen, K. H., Hebbern, C. A., Scmidt, S. B., Pedas, P., Haldrup, A., and Jensen, P. E. (2009). Manganese deficiency leads to genotype-specific changes in fluorescence induction kinetics and state transitions. Plant Physiol. 150, 825–833.
Jarrell, W. M., and Beverly, R. B. (1981). The dilution effect in plant nutrition studies. Adv. Agron. 34, 197–224.
Jensen, L. T., Ajua-Alemanji, M., and Culotta, V. C. (2003). The Saccharomyces cerevisiae high affinity phosphate transporter encoded by PHO84 also functions in manganese homeostasis. J. Biol. Chem. 278, 42036–42040.
Kriedemann, P. E., Graham, R. D., and Wiskich, J. T. (1985). Photosynthetic dysfunction and in vivo changes in chlorophyll a fluorescence from manganese-deficient wheat leaves. Aust. J. Agric. Res. 36, 157–169.
Kyllingsbaek, A., and Hansen, J. F. (2007). Development in nutrient balances in Danish agriculture 1980-2004. Nutr. Cycl. Agroecosys. 79, 267–280.
Leggewie, G., Willmitzer, L., and Riesmeier, J. W. (1997). Two cDNAs from potato are able to complement a phosphate uptake-deficient yeast mutant: identification of phosphate transporters from higher plants. Plant Cell 9, 381–392.
Li, H.-Y., Zhu, Y.-G., Smith, S. E., and Smith, F. A. (2003). Phosphorus-zinc interactions in two barley cultivars differing in phosphorus and zinc efficiencies. J. Plant Nutr. 26, 1085–1099.
Liu, T.-Y., Chang, C.-Y., and Chiou, T.-J. (2009). The long-distance signaling of mineral macronutrients. Curr. Opin. Plant Biol. 12, 312–319.
MacDonald, G. K., Bennett, E. M., Potter, P. A., and Ramankutty, N. (2011). Agronomic phosphorus imbalances across the world’s croplands. Proc. Natl. Acad. Sci. U.S.A. 108, 3086–3091.
Marschner, H., and Cakmak, I. (1986). Mechanism of phosphorus-induced zinc deficiency in cotton. II Evidence for impaired shoot control of phosphorus uptake and translocation under zinc deficiency. Physiol. Plant 68, 491–496.
Miura, K., Lee, J., Gong, Q. Q., Ma, S. S., Jin, J. B., Yoo, C. Y., Miura, T., Sato, A., Bohnert, H. J., and Hasegawa, P. M. (2011). SIZ1 regulation of phosphate starvation-induced root architecture remodeling involves the control of auxin accumulation. Plant Physiol. 155, 1000–1012.
Murphy, L. S., Ellis, R., and Adriano, D. C. (1981). Phosphorus-micronutrient interaction effects on crop production. J. Plant Nutr. 3, 593–613.
Neilsen, D., Neilsen, G. H., Sinclair, A. H., and Linehan, D. J. (1992). Soil phosphorus status, pH and the manganese nutrition of wheat. Plant Soil 145, 45–50.
Ono, T., Noguchi, T., Inoue, Y., Kusunoki, M., Matsushita, T., and Oyanagi, H. (1992). X-ray-detection of the period-4 cycling of the manganese cluster in photosynthetic water oxidizing enzyme. Science 258, 1335–1337.
Pedas, P., Hebbern, C. A., Schjoerring, J. K., Holm, P. E., and Husted, S. (2005). Differential capacity for high-affinity manganese uptake contributes to differences between barley genotypes in tolerance to low manganese availability. Plant Physiol. 139, 1411–1420.
Pedas, P., Ytting, C. K., Fuglsang, A. T., Jahn, T. P., Schjoerring, J. K., and Husted, S. (2008). Manganese efficiency in barley: identification and characterization of the metal ion transporter HvIRT1. Plant Physiol. 148, 455–466.
Perez-Novo, C., Bermudez-Couso, A., Lopez-Periago, E., Fernandez-Calvino, D., and Arias-Estevez, M. (2011). Zinc adsorption in acid soils: influence of phosphate. Geoderma 162, 358–364.
Pittman, J. K. (2005). Managing the manganese: molecular mechanisms of manganese transport and homeostasis. New Phytol. 167, 733–742.
Poage, M., Le Martret, B., Jansen, M. A. K., Nugent, G. D., and Dix, P. J. (2011). Modification of reactive oxygen species scavenging capacity of chloroplasts through plastid transformation. Plant Mol. Biol. 76, 371–384.
Preuss, C. P., Huang, C. Y., and Tyerman, S. D. (2011). Proton-coupled high-affinity phosphate transport revealed from heterologous characterization in Xenopus of barley-root plasma membrane transporter, HvPHT1;1. Plant Cell Environ. 34, 681–689.
Reijneveld, J. A., Ehlert, P. A. I., Termorshuizen, A. J., and Oenema, O. (2010). Changes in the soil phosphorus status of agricultural land in the Netherlands during the 20th century. Soil Use Manag. 26, 399–411.
Requena, L., and Bornemann, S. (1999). Barley (Hordeum vulgare) oxalate oxidase is a manganese-containing enzyme. Biochem. J. 343, 185–190.
Reuter, D. J., Edwards, D. G., and Wilhelm, N. S. (1997). “Temperate and tropical crops,” in Plant Analysis: An Interpretation Manual, 2nd Edn, eds D. J. Reuter and J. B. Robinson (Victoria: CSIRO Publishing), 81–279.
Schachtman, D. P., and Shin, R. (2007). Nutrient sensing and signaling: NPKS. Annu. Rev. Plant Biol. 58, 47–69.
Schjoerring, J. K. (1986). Nitrate and ammonium absorption by plants growing at a sufficient or insufficient level of phosphorus in nutrient solutions. Plant Soil 91, 313–318.
Schjoerring, J. K., and Nielsen, N. E. (1987). Root lenght and phosphorus uptake by 4 barley cultivars grown under moderate deficiency of phosphorus in field experiments. J. Plant Nutr. 10, 1289–1295.
Shane, M. W., and Lambers, H. (2005). Manganese accumulation in leaves of Hakea prostrata (Proteaceae) and the significance of cluster roots for micronutrient uptake as dependent on phosphorus supply. Physiol. Plant 124, 441–450.
Silber, A., Ben-Jaacov, J., Ackerman, A., Bar-Tal, A., Levkovitch, I., Matsevitz-Yosef, T., Swartzberg, D., Riov, J., and Granot, D. (2002). Interrelationship between phosphorus toxicity and sugar metabolism in Verticordia plumosa L. Plant Soil 245, 249–260.
Singh, J. P., Karamanos, R. E., and Stewart, J. W. B. (1988). The mechanism of phosphorus-induced zinc deficiency in bean (Phaseolus vulgaris L.). Can. J. Soil Sci. 68, 345–358.
Stefanovic, A., Arpat, A. B., Bligny, R., Gout, E., Vidoudez, C., Bensimon, M., and Poirier, Y. (2011). Over-expression of PHO1 in Arabidopsis leaves reveals its role in mediating phosphate efflux. Plant J. 66, 689–699.
Valdés-López, O., Yang, S. S., Aparicio-Fabre, R., Graham, P. H., Reyes, J. L., Vance, C. P., and Hernández, G. (2010). MicroRNA expression profile in common bean (Phaseolus vulgaris) under nutrient deficiency stresses and manganese toxicity. New Phytol. 187, 805–818.
Vegh, K. R., Osztoics, E., Csatho, P., Takacs, T., Lukacs, A., and Csillag, J. (2009). Barley root growth and phosphorus bioavailability in soil treated with phosphate rocks. Commun. Soil Sci. Plant 40, 844–853.
Yang, T. J. W., Perry, P. J., Cianai, S., Pandian, S., and Schmidt, W. (2008). Manganese deficiency alters the patterning and development of root hairs in Arabidopsis. J. Exp. Bot. 59, 3453–3464.
Yang, X. J., and Finnegan, P. M. (2010). Regulation of phosphate starvation responses in higher plants. Ann. Bot. 105, 513–526.
Yao, Y. A., Sun, H. Y., Xu, F. S., Zhang, X. J., and Liu, S. Y. (2011). Comparative proteome analysis of metabolic changes by low phosphorus stress in two Brassica napus genotypes. Planta 233, 523–537.
Zadoks, J. C., Chang, T. T., and Konzak, C. F. (1974). A decimal code for the growth stages of cereals. Weed Res. 14, 415–421.
Zhu, J. M., Zhang, C. C., and Lynch, J. P. (2010). The utility of phenotypic plasticity of root hair length for phosphorus acquisition. Funct. Biol. 37, 313–322.
Zhu, Y.-G., Smith, F. A., and Smith, S. E. (2002). Phosphorus efficiencies and their effects on Zn, Cu, and Mn nutrition of different barley (Hordeum vulgare) cultivars grown in sand culture. Aust. J. Agric. Res. 53, 211–216.
Keywords: manganese deficiency, phosphorus, barley, chlorophyll fluorescence, ICP-MS, zinc
Citation: Pedas P, Husted S, Skytte K and Schjoerring JK (2011) Elevated phosphorus impedes manganese acquisition by barley plants. Front. Plant Sci. 2:37. doi: 10.3389/fpls.2011.00037
Received: 30 June 2011;
Paper pending published: 15 July 2011;
Accepted: 19 July 2011;
Published online: 05 August 2011.
Edited by:
Karl H. Muehling, University of Kiel, GermanyReviewed by:
Heiner Goldbach, University of Bonn, GermanyVictoria Fernandez, Technical University of Madrid, Spain
Copyright: © 2011 Pedas, Husted, Skytte and Schjoerring. This is an open-access article subject to a non-exclusive license between the authors and Frontiers Media SA, which permits use, distribution and reproduction in other forums, provided the original authors and source are credited and other Frontiers conditions are complied with.
*Correspondence: Jan Kofod Schjoerring, Plant and Soil Science Section, Department of Agriculture and Ecology, University of Copenhagen, Thorvaldsensvej 40, DK-1871 Frederiksberg C, Copenhagen, Denmark. e-mail: jks@life.ku.dk