- 1College of Life Science, Northwest A & F University, Yangling, China
- 2Department of E-A Information Engineering, Liaoning Institute of Science and Technology, Benxi, China
- 3College of Forestry, Northwest A & F University, Yangling, China
- 4Southern Crop Protection and Food Research Centre, Agriculture and Agri-food Canada, London, ON, Canada
- 5Key Laboratory of South China Agricultural Plant Genetics and Breeding, South China Botanical Garden, Chinese Academy of Sciences, Guangzhou, China
The histone deacetylases play important roles in the regulation of gene expression and the subsequent control of a number of important biological processes, including those involved in the response to environmental stress. A specific group of histone deacetylase genes, HD2, is present in plants. In Arabidopsis, HD2s include HD2A, HD2B, HD2C, and HD2D. Previous research showed that HD2A, HD2B, and HD2C are more related in terms of expression and function, but not HD2D. In this report, we studied different aspects of AtHD2D in Arabidopsis with respect to plant response to drought and other abiotic stresses. Bioinformatics analysis indicates that HD2D is distantly related to other HD2 genes. Transient expression in Nicotiana benthamiana and stable expression in Arabidopsis of AtHD2D fused with gfp showed that AtHD2D was expressed in the nucleus. Overexpression of AtHD2D resulted in developmental changes including fewer main roots, more lateral roots, and a higher root:shoot ratio. Seed germination and plant flowering time were delayed in transgenic plants expressing AtHD2D, but these plants exhibited higher degrees of tolerance to abiotic stresses, including drought, salt, and cold stresses. Physiological studies indicated that the malondialdehyde (MDA) content was high in wild-type plants but in plants overexpressing HD2D the MDA level increased slowly in response to stress conditions of drought, cold, and salt stress. Furthermore, electrolyte leakage in leaf cells of wild type plants increased but remained stable in transgenic plants. Our results indicate that AtHD2D is unique among HD2 genes and it plays a role in plant growth and development regulation and these changes can modulate plant stress responses.
Introduction
In higher plants, transcriptional regulation of gene expression plays an important role in plant life. Post-translational modifications of histones, including acetylation, methylation, phosphorylation, ubiquitinylation, and adenosine diphosphateribosylation, result in modulating dynamic changes in chromatin configuration and gene activity (Berger, 2002; Reyes et al., 2002; Lee et al., 2005). Among these modifications, histone acetylation regulates a number of fundamental biological processes in plants. Histone acetylation levels are regulated by the action of histone acetyltransferases (HAT) that catalyze hyper acetylation and histone deacetylases (HDACs) that catalyze deacetylation by removing the acetyl group from the core histones. HDACs are an ancient group of enzymes that are widely spread in various organisms.
HDACs in plants can be divided into three families based on phylogenetic analysis. They are reduced potassium dependency 3(RPD3) or histone deacetylase 1 (HDA1), silent information regulator 2 (SIR2), and histone deacetylase 2 (HD2, also HD-tuins; Taunton et al., 1996; Wu et al., 2000; Tian and Chen, 2001; Pandey et al., 2002; Liu et al., 2014; Zhao et al., 2014). The HD2 family is specific to plants and is unrelated to the RPD3/HDA1 and SIR2 HDAC types (Sridha and Wu, 2006; Grandperret et al., 2014; Zhao et al., 2014). The first HD2 was isolated as an acidic nuclear phosphoprotein from maize embryos (Lusser et al., 1997). Expressed sequence tag (EST) homology searches identified four HD2 genes in Arabidopsis, namely, HD2A, HD2B, HD2C, and HD2D (also known as HDT1, HDT2, HDT3, and HDT4, respectively; Dangl et al., 2001; Pandey et al., 2002; Zhou et al., 2004). Bioinformatics analysis of the HDAC domains of these HD2 proteins revealed a series of highly conserved motifs. A phylogenetic analysis of the nucleotide sequences indicates that a single HD2 gene led to the development of all HD2 genes in both monocot and dicot plants (Pandey et al., 2002).
It was demonstrated that AtHD2A, AtHD2B, and AtHD2C were able to mediate transcriptional repression (Wu et al., 2000, 2003; Zhou et al., 2004). The findings that AtHD2A was required for deacetylation and subsequent methylation of H3 Lys9 supported the idea that HD2 proteins repressed gene expression by modifying histone (Lawrence et al., 2004). Research showed that HD2A, HD2B, and HD2C were all localized in the nucleolus (Lusser et al., 1997; Lawrence et al., 2004; Zhou et al., 2004) and were expressed globally in Arabidopsis (Lawrence et al., 2004; Zhou et al., 2004; Hollender and Liu, 2008). Gene expression mediated by HD2A, 2B, and 2C can regulate several aspects of plant development. It was shown that the repression of AtHD2A expression led to the development of a short silique and the abortion of seeds (Lagacé et al., 2003). Ectopic expression of AtHD2A created a mutant phenotype, characteristics of which included the narrowing and curling of leaves, abnormal flower development, and delayed blooms (Lagacé et al., 2003). AtHD2B expression was up-regulated by low temperature and after-ripening (dry storage of mature seed) treatments that are known to break seed dormancy, so suppression of AtHD2B expression was important in maintaining seed dormancy (Yano et al., 2013). In tobacco (Nicotiana tobacum), NtHD2a and NtHD2b were found to act as negative regulators of cell death induced by the elicitor cryptogein (Bourque et al., 2011). During longan (Dimocarpus longan) fruit senescence under different storage conditions, a histone deacetylase 2-like gene (DlHD2) may interact with an ethylene-responsive factor-like gene (DlERF1) to regulate expression of genes involved in fruit senescence (Kuang et al., 2012).
Several studies have shown that certain HD2s are involved in plant responses to abiotic stresses. Sridha and Wu (2006) showed that expression of AtHD2C was largely repressed by abscisic acid (ABA), a hormone that regulates plant response to abiotic stresses. Over-expression of AtHD2C in Arabidopsis resulted in plants that were insensitive to ABA. Further, transgenic AtHD2C plants exhibited reduced transpiration and increased tolerance to salt and drought stresses compared to wild-type plants. Luo et al. (2012a) showed that several hd2c mutants were more sensitive to ABA and NaCl during seed germination. The plants with HD2C insertion mutation displayed decreased tolerance to salt stress. Using both BiFC and co-immuno precipitation assays, the study found that HD2C physically interacted with HDA6, another type of HDAC, and the abiotic stress response was modulated through the HD2C/HDA6 complex. Further, research showed that histone H3 was a target of the HD2C/HDA6 complex. A more recent study showed that there existed at least two HD2 genes in rice, HDT701 and HDT702. Overexpression of HDT701 in rice resulted in an increased tolerance to salt and drought during seedling stage (Zhao et al., 2015). These studies suggest that plant responses to abiotic stresses are controlled by an antagonistic mechanism of histone acetylation and deacetylation on expression of target genes and that HD2s play unique roles in plant reaction to environmental stresses.
Previous research indicates that the expression profile of HD2D gene was largely different from other HD2 genes. The expression HD2A, HD2B, and HD2C was detected in ovules, embryos, shoot apical meristems, and primary leaves and the expression of these genes were strongly induced during somatic embryogenesis. On the other hand, HD2D transcript was only detected in the stems and flowers with young siliques (Zhou et al., 2004). The authors suggest that HD2D may have adopted different functions. At this time, little is known about the specific expression and function of the HD2D gene in plants. In this study, we provided evidence that Arabidopsis AtHD2D is localized in the nucleus and it is involved in regulating the growth of roots and flowers in Arabidopsis. When AtHD2D was overexpressed, Arabidopsis plants displayed more tolerance to drought, salt, and cold stresses compared to wild-type plants. The research indicates that HD2D is an important protein in plants for environmental stress response.
Materials and Methods
Gene and Protein Sequence Analyses
The AtHD2 gene sequence analysis was conducted using the ExPASy net (Bioinfor- matics Resource Portal (http://www.expasy.ch/tools/#proteome). DNAMAN (Version 5.5.2, Lynnon Biosoft) was used forsequence alignment analysis of AtHD2 coding-sequence (CDS). Considering HD2s belong to one family and share certain similarities, we used Maximum Parsimony method for phylogenetic tree construction. Bootstrap was used to test the developed trees. MEGA 6.0 was used in the analysis. The bioinformation of AtHD2D gene expression product was predicted by primary structure analysis. Two software programs, ProtParam, and PSORT II Prediction, were used to predict the subcellular localization of AtHD2 proteins. In addition, the tool for transmembrane regions and signal peptide sequence analysis, ProtComp 9.0 tool (http://linux1.softberry.com/berry.phtml?topic=protcomppl&group=programs&subgroup=proloc) fuzzy k-nearest neighbors (k-NN) algorithm (version 41.0), and TMHMM Server v. 2.0 were used for AtHD2 protein analysis.
Plant Materials and Growth
Arabidopsis thaliana (ecotype, Columbia) was used in this research. Arabidopsis seeds were surface-sterilized in 70% ethanol for 1, 10 min in 10% v/v sodium hypochlorite, and rinsed 5 times with sterile distilled water. The seeds were sown on MS medium (Murashige and Skoog, 1962; Sigma-Aldrich, Lenexa, KS, USA) in Petri dishes. The plants were maintained in a tissue culture room with 16 h of light at 25°C/8 h of dark conditions at 22°C. Germinated seedlings were transferred into soil (PRO-MIX “BX” with Mycorise PRO, Primer Horticulture, Grower Services Canada). Plant seedlings were grown in a growth chamber under 16 h of light at 25°Cand 8 h of dark conditions at 22°C (unless for stress treatments which are described specifically). Each overexpression independent line of Arabidopsis was grown in one of conjoined pots (9 × 12 cm, height × diameter). Each pot was planted with 12 seedlings, all from the same clone. The overexpression seedlings of the same independent line were planted into pots randomly for different stress treatments. After plants were transferred into soil, each pot was covered with transparent film for 4 days to avoid rapid water loss.
DNA Constructs
The putative HD2D mRNA complete coding sequence (CDS) was obtained from the NCBI (National Center for Biotechnology Information; http://www.ncbi.nlm.nih.gov/nucleotide/145360405?report=genbanklog=nucltop) and TAIR (The Arabidopsis Inform- ation Resource (http://www.arabidopsis.org/servlets/TairObject?type=locus&name=AT2G27840) databases. According to the coding sequence, the 35S:AtHD2D vector for overexpression and 35S:AtHD2D-gfp vector for HD2D subcellular location were constructed. The AtHD2D was amplified using the primer pair of pr1 (5′-ACCAGATCTATGGAG TTTTGGGGTATCGAG-3′) and pr2 (5′- CCACTAGTCTACTTT TTGCAAGAGGGACCAC-3′) and fusion AtHD2D-gfp was amplified using the pr1 and pr3 (5′-CCACTAGTCTTTTTGC AAGAGGGACCAC-3′; Supplementary Table 1) and all with appropriate restriction sequences (BglII and SpeI). Phusion high-fidelity DNA polymerase (F-530S, 100U New England Biolabs, Inc. USA) was used in the PCR reaction to minimize undesired mutations in the sequences. The construct for subcellular location of AtHD2D was made by in-frame fusion of complete AtHD2D CDS with the gfp sequence in which the stop codon (TAG) of AtHD2D CDS was deleted. The resulting PCR products were cloned to pGEM®-T and pGEM®-T Easy Vector Systems (A1360 Promega Corporation) digested by BglII and SpeI and then transferred into the pCAMBIA1302 binary vector containing the hptII coding for hygromycin resistance which was used as the selectable marker for plant transformation selection. The constructs were introduced into Agrobacterium strain GV3101/pMP90 by electroporation using the GENE PULSER II system (Bio-Rad, Hercules, CA, USA).
Plant Transformation
The 35S:AtHD2D vector and 35S:AtHD2D-gfp vector were introduced into Arabidopsis separately for overexpression studies and for subcellular location studies, respectively. Arabidopsis transformation followed the floral dip method (Clough and Bent, 1998). T1 seeds were harvested, dried at 25°Cand germinated on MS medium containing 30 μg mL−1 hygromycin to select transgenic seedlings, and 50 μg mL−1 carbenicillin was added to prevent Agrobacterium contamination. Seeds obtained from the primary transgenic lines were germinated on antibiotic–containing medium, and PCR analyses were performed on resistant plants. Surviving and PCR analysis positive T1 plantlets were transferred to soil to harvest T2 seeds. T2 seeds were germinated as described before and T3 seeds were harvested. Plants developed from T3 seeds were used as the materials for all experiments.
To produce the Arabidopsis plants for confocal fluorescence observation, seeds were sown on MS medium in vertical plates and the plants were grown for 6 days. Root tips were harvested and used for observation. Nicotiana benthamiana were grown as described by Han et al. (2013), and plants were maintained in growth chambers for investigating the subcellular localization of HD2D-GFP in transient gene expression experiments. The chamber conditions were set at 14 h light and 10 h dark at 21°C. Young leaves were infiltrated with Agrobacterium GV3101/pMP90 containing the HD2D-gfp construct and observations were conducted 48–72 h after infiltration.
Molecular Analysis of Plants
Total genomic DNA was extracted from plant leaves using a CTAB method as described by Wang et al. (1993). Total RNA was isolated from leaves using TRIzol reagent (Invitrogen Life Technologies, Carlsbad, CA) following manufacturer's instructions. Primers specific to the hptII gene for a 563 bp PCR product (Supplementary Table 1) were used in PCR analysis of plants. The pr1 and pr2 primers specific for HD2D, and the pr1 and pr3 primers specific for HD2D-gfp were also used in PCR analysis. The primer for the Actin2 gene of Arabidopsis (Czechowski et al., 2004) was used as an internal control.
All PCR reactions were performed with 1 unit of Taq polymerase (GenScript, the Biology Co, USA), 0.2 mM dNTPs, and a pair of primers (0.1 μM each) in a final volume of 20 μl. PCR was conducted at 94°C for 2 min followed by 30 cycles of 94°C for 15 s, 55–57°C for 20 s, and 72°C for 45 s, with a final polymerization step at 72°C for 3 min.
For RT-PCR analysis, 2 μg of each total RNA sample was treated with the RNase-free DNase according to manufacturer's instructions (Promega). Treated RNA samples were desalted (to prevent carry-over of magnesium) before cDNA synthesis using Microcon-100 spin columns (Millipore). The first-strand cDNA was synthesized using random hexamers and SuperscriptII reverse transcriptase (Invitrogen), according to manufacturer's instructions, and subsequently diluted with nuclease-free water (Sigma) to 12.5 ng μl−1 cDNA. Reactions were run on a thermocycler (model Mastercycler gradient; Eppendorf Canada Ltd., Mississauga, Ontario) using the same conditions as described above.
Plant Growth Evaluation
Only seed batches that had been harvested and stored at the same time and under the same conditions were used. Seeds were sown on MS medium, vernalized at 4–8°C and then transferred to a tissue culture room under 16 h of light at 25°C/8 h of dark at 22°C. The germination rate (defined as the emergence of the hypocotyl axis through the seed coat) was determined at different days after seeds were placed for germination. Experiments were conducted in triplicate with 180 seeds for each experiment. For investigation of root growth, seeds were sterilized and grown on vertically oriented MS agar plates in a tissue culture room. Transgenic plants and wild-type plants were grown in adjacent parallel rows in the same Petri dishes (9 cm), 10 transgenic seedlings in one row and 10 wild-type seedlings in the other. After photos were taken, the number of lateral roots was counted under a dissecting microscope. The plants were placed on a flat glass and then main roots and lateral roots were straightened as much as possible using a forceps. A verniercalliper was used for measuring the length of main and lateral roots. For determination of root and shoot ratio, 10 day old plants were removed from plates, medium residues were cleaned from plants and plants were blotted dry using filter papers. Roots were cut off from stems (with leaves) and roots and shoots were dried in an oven. The dry matters were weighed and root:shoot ratio was calculated. For flowering assessment, 5 days after seed germination on MS medium, the seedlings were transferred into soil-containing pots. Twenty-six days after germination when plants started to show the rosette stage of leaf growth and plants showed sign of flowering, flowering was evaluated, which was based on plant bolting and the formation of flower buds. All tests were replicated three times and each replicate consisted of at least six independent lines.
Stress Treatment
Stress treatments were initiated after plants were grown for 10–13 days in soil under regular conditions. Each treatment included six independent lines with three replicates for overexpression and wild type. The results presented in figures were calculated based on the values of all six independent lines and three replicates. The drought treatment was conducted by withholding water after seedlings were transplanted into pots. For cold treatment, plants were moved to a growth chamber at 2°C, and leaf samples were collected every 2 days. For salt stress, water containing 300 mmol per L of NaCl was evenly spayed on the soil surface and leaf samples were collected every 3 days.
With the time extension under stresses, plant leaves gradually turned brown then dark brown. When 50% leaf area had turned brown, the leaf was counted as brown leaves. Leaves under salt stress gradually turned yellow and wilted. When 50% leaf area had turned yellow the leaf was recorded as withered leaves.
Detection of GFP Signals by Confocal Laser Scanning Microscopy
Transient expression and stable expression of 35S:GFP and 35S:AtHD2D-GFP fusion protein in N. benthamiana leaves and Arabidopsis root tips were detected using laser fluorescence microscopy (Sparkes et al., 2006). Photographs were taken using a confocal florescent microscope (Leica Microsystems, Germany) under excitation at 450–490 nm, emission at 520 nm and dichroic mirror at 510 nm.
Malondialdehyde (MDA) and Electrolyte Leakage and Analysis
Malondialdehyde (MDA) content and electrolyte leakage were used to analyze stress damage to plants (Campos and Nunes, 2003). For MDA analysis, the cell injury level was expressed as the content of MDA according to Zhang et al. (2005). Fresh leaves from each treatment were homogenized in 5 mL of 10% trichloroacetic acid (TCA), and then centrifuged at 4000x g for 20 min. To each 2 mL aliquot of the supernatant, 2 mL of 0.6% thiobarbituric acid in 10% TCA was added. The mixtures were heated at 100°C for 15 min and then quickly cooled in an ice bath. After centrifugation at 10,000x g for 20 min, the absorbance of the supernatant was recorded at 532 and 450 nm. Lipid peroxidation was expressed as the MDA content in nmol per g FW. For electrolyte leakage analysis, freshly cut leaf discs of 0.5 cm2 each were rinsed 3 times (2–3 min) with demineralized water and subsequently floated on 20 mL of demineralized water. The electrolyte leakage in the solution was measured after 24 h room temperature using a conductivity meter (Smart Stability, Orion, Thermo Scientific U.S.A). Total conductivity was obtained after the flasks were maintained in an oven (90°C) for 2 h.
Statistical Analysis
Data analysis was performed using SPSS 16.0.2 computer statistical program (IBM SPSS, 2008). The data are presented as the mean ± standard deviation of the mean (SD) and were tested for significant difference using Student's t-test. p < 0.05 was selected as the point of minimal significant difference in all of the analyses.
Results
Bioinformatics Analyses of AtHD2 Genes and Proteins
DNA sequence analysis showed that the overall homology of AtHD2 genes is 35% (Figure 1A). Sequence homology of AtHD2A and AtHD2B was 59% which is the highest. AtHD2D is distantly related to the other AtHD2 genes, and this observation is supported by the sequence homology value of only 35%. Meanwhile the phylogenetic tree showed that the genetic distance (GD) between AtHD2A and AtHD2B was 0.042, next was AtHD2C, and the largest GD was AtHD2D with a value of 0.056 (Figure 1B). Protein sequence alignment showed that the overall similarity of the HD2 proteins was 51% (Figure 1C). The phylogenetic tree showed that AtHD2A and AtHD2B were the closest, the GD being the smallest. On the other hand, the AtHD2D protein was furthest among the HD2 proteins with a GD-value of 0.481 (Figure 1D).
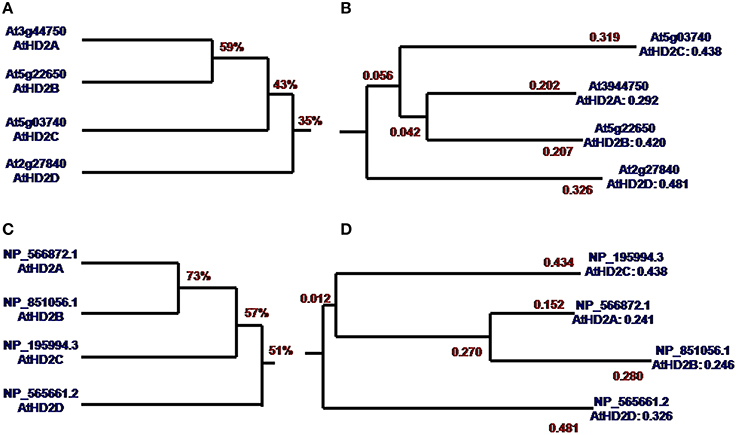
Figure 1. Homology tree and phylogenetic tree of AtHD2 genes and AtHD2 proteins. (A) CDS of AtHD2 gene homology tree. (B) CDS of AtHD2 gene phylogenetic tree. (C) AtHD2 protein homology tree. (D) AtHD2 protein phylogenetic tree.
The analysis of HD2 genes showed that HD2D is largely different from other HD2 genes. The molecular weight of HD2D is significantly lower compared to other HD2 genes (Supplementary Table 2). While HD2A, HD2C genes contain the zinc finger domain, the HD2D lacks this important region. The HD2D showed the lowest random coil value and the highest values of β-turn, pI and extended strand (Supplementary Table 2).
Plant Transformation and Transgene Gene Expression Analyses
Plants of 30 randomly selected independently lines developed on medium containing hygromycin were analyzed by PCR and RT- PCR using specific primers (Supplementary Table 1, Figure 2A). All the lines analyzed were PCR positive for HD2D using pr1 and pr2 primer pair as shown in a representative image Figure 2B. The RNA expression of transgene was analyzed for randomly selected independent lines using RT-PCR using transgene specific primers (Figures 2A,C, Supplementary Table 1) and the actin2 gene was used as the internal control. The RNA expression of AtHD2D was increased in 35S:AtHD2D transgenic lines comparing to the control (Figure 2C). Plants transformed with AtHD2D-gfp showed expression of the fused gene (Figure 2C) but no expression of AtHD2D-GFP was observed in the non-transformed wild type. Expression of actin2 as the internal control was consistent in both non-transformed wild types and transformed plants (Figure 2C). These results confirmed the expression of transgenes in plants developed following transformation.
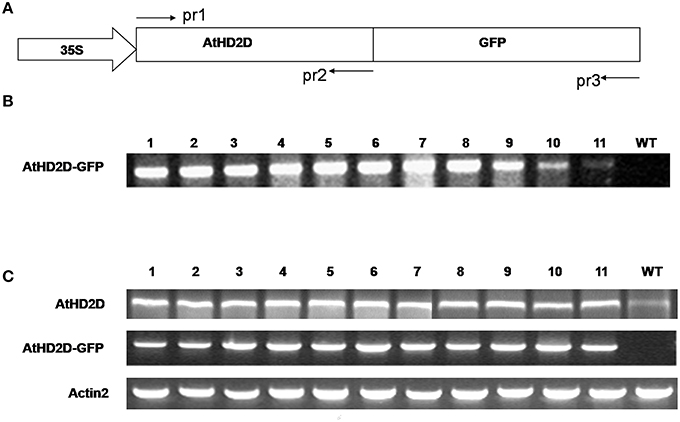
Figure 2. Expression analysis of AtHD2D and of AtHD2D-gfp in transgenic Arabidopsis thaliana plants. (A) Diagram of the 35S:AtHD2C-gfp construct. AtHD2D CDS was fused with the gfp-coding region driven by a 35S promoter. The primers, pr1, pr2, and pr3, used to analyze AtHD2D and AtHD2D-gfp in transgenic plants were shown. (B) PCR analysis of 35S:AtHD2D-gfp transgenic and wild-type (WT) plants using primer pairs of pr1 and pr3. No PCR product was found in WT. (C) RT-PCR analysis of AtHD2D and AtHD2D-gfp expression in transgenic and wild-type plants using the primer pairs of pr1 and pr2 or pr1 and pr3. No RT-PCR product of AtHD2D-gfp was found in WT.
Expression Localization of HD2D
Two software programs, ProtComp and PSORT II Prediction, were used to predict the subcellular location of AtHD2 protein. The HD2D protein was positioned in the cell nucleus but with the lowest value compared to other HD2 genes (Supplementary Table 3) and it does not have a membrane spanning domain nor a signal peptide. Meanwhile, HD2D showed a high possibility to localize in cytoplasm and the highest possibility to be distributed in cytoskeleton, mitochondria, and peroxisome. AtHD2D also had the lowest integral prediction value. The actual location of the AtHD2D protein was studied via gene expression experiments. N. benthamiana leaves were infiltrated with a construct containing AtHD2D fused with gfp and the expression was observed 48–72 h after the infiltration. The gfp gene alone driven by CaM35S promoter showed constitutive protein expression activity in plant cells (Figure 3A). The expression of AtHD2D-GFP was mainly localized in nucleus (Figures 3B,C). There were halos around the fusion protein, as opposed to clear boundariess which were very sharp and without a fringe or halo, as seen in other AtHD2 proteins.
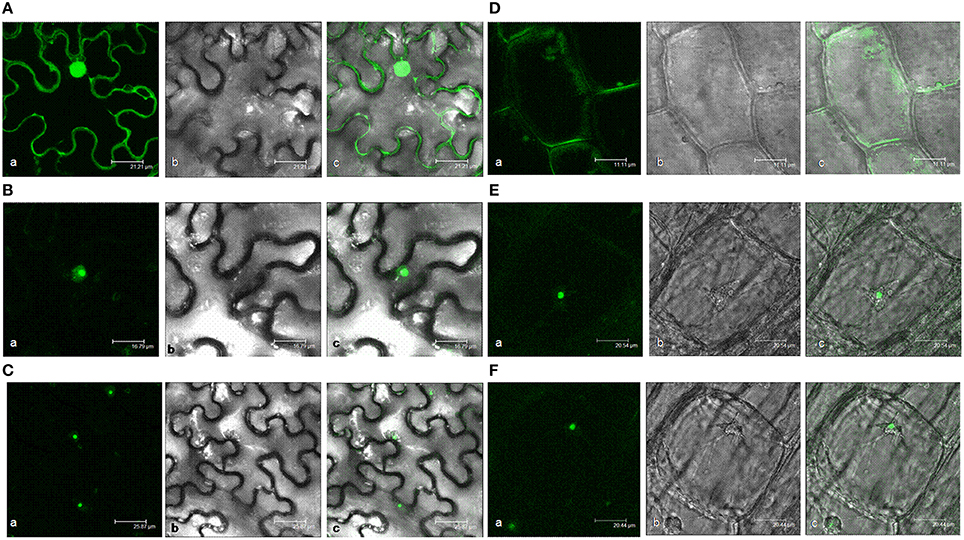
Figure 3. Subcellular localization analyses of 35S:AtHD2D and 35S:AtHD2D-GFP. (a) Fluorescence image, (b) With white light, (c) Merge of fluorescence and bright images. (A) Subcellular localization of 35S:GFP in N. bentamiana. (B) Subcellular localization of AtHD2D-GFP in N. bentamiana. (C) General view of subcellular localization of AtHD2D-GFP in N. bentamiana. (D) Subcellular localization of 35S:GFP in root tip of transgenic Arabidopsis. (E) Subcellular localization of 35S:AtHD2D-GFP in root tip of transgenic Arabidopsis. (F) General view of subcellular localization of 35S:AtHD2D-GFP in root tip of transgenic Arabidopsis.
AtHD2D subcellular localization was further studied via stable expression of the gene in Arabidopsis. Two transgenic lines with higher levels of AtHD2D-gfp expression were selected in the study. Stable expression of AtHD2D gene in transgenic Arabidopsis root cells was observed. Plants transformed with gfp driven by 35S promoter showed constitutive expression in root cells (Figure 3D). Plants transformed with a construct carrying the fusion AtHD2D-gfp showed that GFP was expressed in the nucleus (Figures 3E,F). Both transient and stable expression studies indicated that the location of AtHD2D is mainly in the cell nucleus.
Involvement of AtHD2D in Plant Growth
Seed germination was evaluated for plants overexpressing the AtHD2D. Germination of seeds from transgenic plants was slower compared to the wild-type (Figure 4A). While germination of the wild type seeds was 75.6% in 2 days and 98.2% by day 4, germination of transgenic seeds was delayed by 5–6 days.
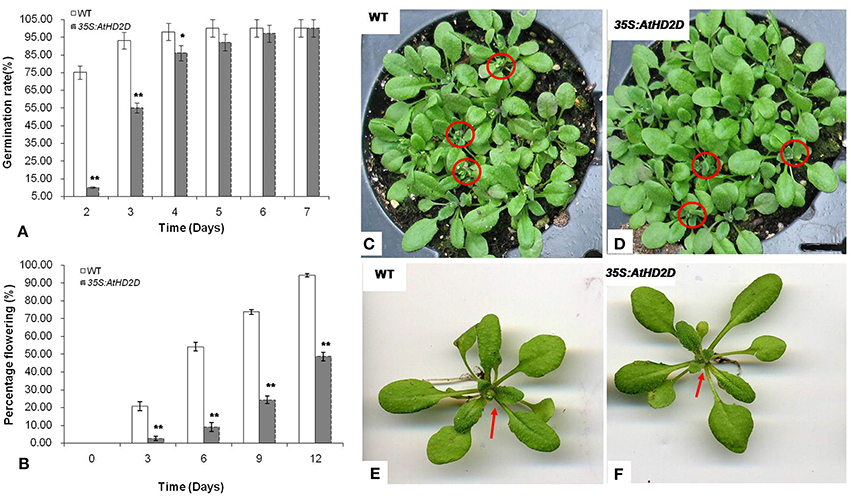
Figure 4. Seed germination and flowering of wild type (WT) and 35S: AtHD2D transgenic plants under regular conditions on MS medium. The data are presented as the mean ± standard deviation of the mean (SD) and were tested for significant difference using Student's t-test (*p < 0.05, **p < 0.01). (A) Seed germination rate of WT and 35S:AtHD2D transgenic plants. (B) Plant flowering rate of WT and 35S:AtHD2D transgenic seedlings. (C,E) WT seedlings. (D,F) 35S:AtHD2D transgenic seedlings.
Flowering time was significantly delayed in transgenic plants (Figure 4B). During the 12 day observation period, transgenic plants consistently showed lower flowering rates compared to wild type plants. By day 12, about 90% of WT plants showed bloom development, but transgenic plants were largely still in a vegetative growth stage (Figures 4C–F).
Ten days after germination on MS medium, the root development was evaluated. The plants overexpressing AtHD2D had shorter main roots and longer lateral roots (Figures 5A,C). In contrast, the wild type plants under the same growth conditions had obviously longer main roots but shorter lateral roots (Figures 5B–D). Transgenic plants also had more lateral roots compared to wild type plants (Figures 5A,C). The root:shoot ratio of transgenic plants was higher than that of wild type plants (Figure 5D).
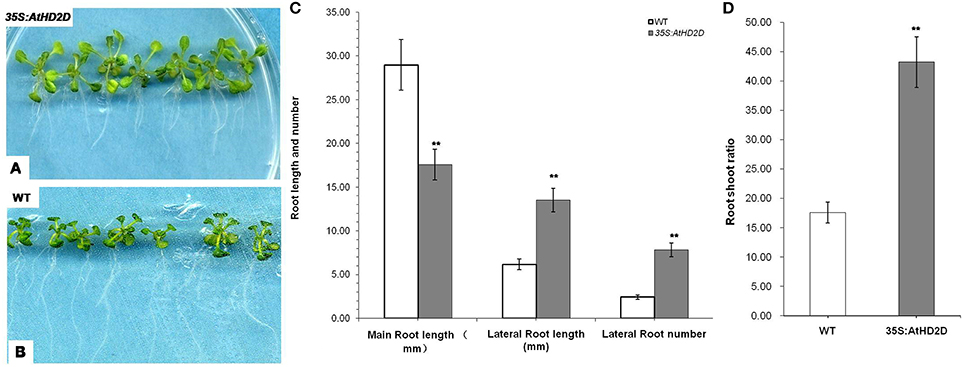
Figure 5. Root development of wild-type and 35S:AtHD2D transgenic plants. The error bars represent the SD. The data are presented as the mean ± standard deviation of the mean (SD) and were tested for significant difference using Student's t-test (**p < 0.01). (A,C) Roots of wild-type (WT) 10 days after germination. (B,D) Phenotype of roots and leaves of 35S:AtHD2D of transgenic seedlings 10 days after germination. (E) Root and shoot ratio of WT and 35S:AtHD2D transgenic plants 10 days after germination on MS medium. (F) Root length and root number of WT and 35S:AtHD2D transgenic plants.
Involvement of HD2D for Stress Tolerance
Plants overexpressing AtHD2D showed increased tolerance to cold, drought, and salt stresses (Figure 6). Under cold and drought conditions, the HD2D overexpressing plants grew normally and retained normal green color in leaves (Figures 6A,B,D,E). However, wild type plants grew slowly and the leaves wilted and turned brown and showed stressed phenotypes. Under salt stress, wild type plants became yellow in color and plants were obviously injured but plants overexpressing HD2D grew relatively normally and produced flowers (Figures 6C,F).
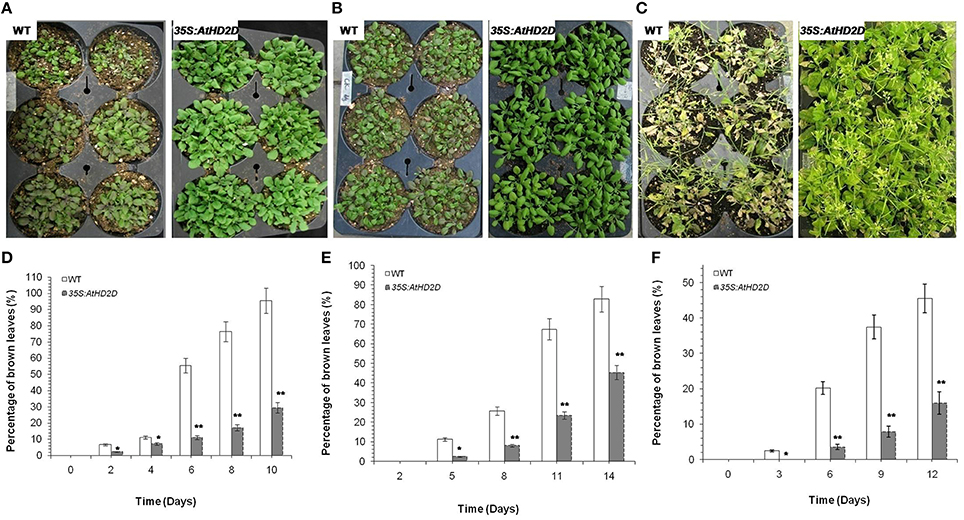
Figure 6. Full view of wild type (WT) and 35S:AtHD2D transgenic plants under abiotic stresses. The error bars represent the SD. The data are presented as the mean ± standard deviation of the mean (SD) and were tested for significant difference using Student's t-test (*p < 0.05, **p < 0.01). (A) WT and 35S:AtHD2D transgenic plants under cold stress for 8 days. (B) WT and 35S:AtHD2D transgenic plants under drought stress conditions for 9 days. (C) WT and 35S:AtHD2D transgenic plants under salt stress conditions for 18 days. (D) Browning levels in leaves of WT and 35S:AtHD2D transgenic plants under cold stress. (E) Browning levels in leaves of WT and 35S:AtHD2D transgenic seedlings under drought stress. (F) Browning levels in leaves ofWT and 35S:AtHD2D transgenic seedlings under salt stress.
Based on Campos and Nunes (2003), MDA and electrolyte leakage were conducted. MDA levels in transgenic plants overexpressing AtHD2D increased slowly under cold, drought and salt conditions, while MDA levels in wild type leaves increased rapidly over the time (Figures 7A–C). Analysis of electrolyte leakage from leaves (an indicator of cell membrane damage) during the course of abiotic stresses showed that electrolyte leakage of wild type plants increased steadily, while electrolyte leakage in transgenic plants overexpressing AtHD2D increased slowly and remained relatively stable (Figures 7D–F).
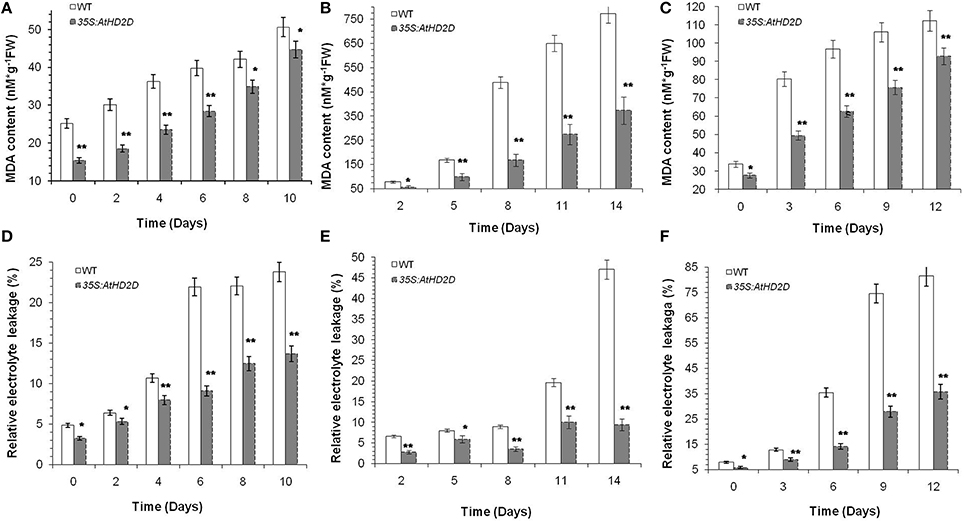
Figure 7. Malonaldehyde (MDA) and electrolyte leakage levels in WT and 35S:AtHD2D transgenic plants under abiotic stresses. The error bars represent the SD. The data are presented as the mean ± standard deviation of the mean (SD) and were tested for significant difference using Student's t-test (*p < 0.05, **p < 0.01). (A) MDA level under cold stress condition. (B) MDA level under drought stress condition. (C) MDA level under salt stress condition. (D) Electrolyte leakage level under cold stress. (E) Electrolyte leakage level under drought stress. (F) Electrolyte leakage level under salt stress.
Discussion
HDACs function by removing the acetyl groups from lysine residues, thus allowing the nucleosomes to pack more tightly which consequently represses transcription (Song et al., 2005; Liu et al., 2014). Silencing gene expression by HDACs is selective and altered gene expression controls certain biological processes, especially plant development and response to environmental stresses (Kim et al., 2010; Yano et al., 2013; Kim et al., 2015; Pi et al., 2015; Yang et al., 2015; Zhao et al., 2015). Even in the earliest phases of plant development, such as embryogenesis, the histone code is necessary to establish the correct developmental pathways (Tai et al., 2005; Köhler and Makarevich, 2006; Long et al., 2006; Kim et al., 2010; Wickramasuriya and Dunwell, 2015). During later stages of the plant life cycle, histone modifications influence growth phase change (Vlachonasios et al., 2003; Chua et al., 2005; Rossi et al., 2007; Yano et al., 2013), flowering timing (He and Amasino, 2005; Deng et al., 2007; Luo et al., 2015), and maternal imprinting and fertilization (Guitton and Berger, 2005; Köhler and Makarevich, 2006).
The first HD2 was discovered in maize (Lusser et al., 1997). A comparative analysis of the HD2 type of HDAC proteins in higher plants revealed three HD2 genes in maize and four genes in Arabidopsis (Dangl et al., 2001). The uniqueness and specificity of HD2s to plants suggests this type of HDACs possess specific features and functions (Grandperret et al., 2014). Previous studies have focused on HD2A, HD2B, and HD2C and less research has been done for HD2D. Limited research indicates, to certain extent, that HD2D is different from other HD2 genes in expression and potential functions (Zhou et al., 2004). Our research studied AtHD2D, especially with respect to plant development and plant response to environmental stresses.
Bioinformatics analysis and prediction are based on the information accumulated in molecular biology databases. We did a series of bioinformatics analysis on AtHD2D. The DNA sequence analysis showed that AtHD2D tends to be distantly related to other AtHD2 genes. The phylogenetic tree also shows the genetic distance of AtHD2D is the furthest. Protein sequence alignment also showed that AtHD2D protein is less related to other HD2 proteins. The analysis of AtHD2 gene composition shows that AtHD2D appears to be different from other AtHD2 genes in several categories. All these suggest that AtHD2D is relatively unique among AtHD2 genes. Although it retains certain characteristics typical of AtHD2 genes, AtHD2D probably branched off from AtHD2s a long time ago during evolution.
AtHD2s have a conserved N-terminal EFWG (well-conserved motif) amino acid region which is required for repression of gene expression, followed by a central acidic region rich in glutamic and/or aspartic acids. In AtHD2A, this acidic region is essential for catalytic activity, since the deletion of this region resulted in a compromised HDAC activity (Wu et al., 2000). HD2A and HD2C contain a single C2H2 type zinc finger domain in the carboxyl terminus, which may enable high affinity DNA-binding or mediate protein-protein interactions (Aravind and Koonin, 1998; Wu et al., 2000; Dangl et al., 2001; Zhou et al., 2004). Previous research showed that while other AtHD2A, AtHD2B, and AtHD2C exhibited an effective repression of gene expression, AtHD2D was much weaker to repress reporter gene expression (Zhou et al., 2004). As AtHD2B also lacks the zinc finger domain, the zinc finger may not be essential for repression of gene expression.
The analysis revealed that there is no signal peptide in the full coding sequence of AtHD2D, therefore, HD2D is not a secretory or mosaic protein in membranes. Bioinformatics analysis indicated that the products of AtHD2D tend to be in cytoplasm. However, transient expression analysis in N. benthamiana and stable expression in Arabidopsis suggested that AtHD2D-GFP was mainly localized in the nucleus of cells in both plant species. A study by Luo et al. (2012b) showed HD2D interacted with RPD3 family proteins of HDA6 and HDA19 and the signals were observed in cell nucleus, suggesting nucleus localization of HD2D. AtHD2D was not diffusely distributed in the nucleoplasm but was localized in approximately half the nucleus. The results also indicate that sequence analysis predictions do not reflect the real expression and localization of AtHD2D in cells and these outcomes are different from other AtHD2 proteins. A recent study by Zhao et al. (2015) showed that different members of tomato SiHDACs had different subcellular localizations and they proposed that different SiHDAC genes have distinct functions. Bioinformatics analysis and gene expression localization study results suggest that AtHD2D is unique among AtHD2s with respect to gene and protein composition as well as the localization of gene expression (Lawrence et al., 2004; Zhou et al., 2004; Sridha and Wu, 2006). The differences in these categories suggest the HD2D may have some different functions among HD2s.
Plant phenotypical characteristics of root development showed that 35S-AtHD2D transgenic plants had more lateral roots compared to wild-type. Root:shoot ratio was also higher in transgenic plants. Nguyen et al. (2013) showed that histone deacetylase inhibitors impeded root development including primary root elongation as well as lateral root emergence. The research further showed that the lateral root growth was significantly reduced in hda19 seedlings in which the HDA19 was mutated. More lateral roots and higher root:shoot ratios as shown in this study suggest that such structures can help plants to obtain more nutrients and water, which enable plants to cope with unfavorable conditions, especially environmental stresses. The results indicated that transgenic plants expressing higher levels of HD2D may adopt more plasticity in response to abiotic stresses, particularly drought stress.
Histone modification appears to act by integrating signals from different environment stimuli in order to regulate plant growth and development (Stockinger et al., 2001; Lee et al., 2005). Published data also show that each HDAC may have special functions during plant development or upon exposure to certain environmental stimulations, and moreover, one HDAC enzyme can also affect several processes in plant growth (Pi et al., 2015, Kim et al., 2015). The switch from vegetative growth to flowering is a major developmental transition that is initiated by environmental signals, such as the cold weather (vernalization) and changes in day length. Several studies have directly or indirectly indicated involvement of HDACs in plant flowering (Sheldon et al., 1999, 2000; Bastow et al., 2004; Sung and Amasino, 2004; He and Amasino, 2005; Deal et al., 2007; Yu et al., 2011; Krogan et al., 2012; Luo et al., 2015). However, not all HDACs are involved in plant development and flowering, and further, the mechanisms by which a HDAC is involved in plant growth and development may be different for different HDAC genes (Nguyen et al., 2013; Van Zanten et al., 2014). Our study showed that AtHD2D overexpressing plants exhibited delayed seed germination. The flowering time was also delayed in plants overexpressing AtHD2D. A recent study by Farhi et al. (unpublished results) showed that a significantly higher number of rosette leaves was observed in AtHD2D-overexpressing Arabidopsis plants when these plants exhibited delayed flowering (Farhi and Tian, unpublished results). This indicates the late flowering is due to longer vegetative plant growth. Our study demonstrates that AtHD2D directly influenced plant development. A longer period of vegetative growth or delayed onset of flowering can help plants to absorb more nutrients for later reproductive growth to cope with environment stresses. Further study is needed to reveal the mechanisms by which HD2D is involved in plant growth and development.
We conducted further research to test AtHD2D responses to abiotic stresses. Transgenic 35S:AtHD2D plants were grown under drought, cold, or salt stress conditions. AtHD2D transgenic seedlings displayed a decreased sensitivity to drought, cold, and salt stresses. Further, changes of MDA and electrolyte leakage were analyzed in 35S:AtHD2D transgenic plants and control plants. Higher content of MDA inhibits cell metabolism and can lead to cell death (Campos and Nunes, 2003). When wild type seedlings were exposed to stresses, MDA content increased quickly. On the other hand, MDA in the 35S:AtHD2D transgenic seedlings increased slowly and the values remained lower throughout stress treatments. Electrolyte leakage in leaves under stress indicates membrane damage and subsequent cell damage (Rolny et al., 2011), with electrolyte leakage increasing as damage increases. Analysis showed that electrolyte leakage of 35S:AtHD2D transgenic seedlings were not largely affected under different stresses while the electrolyte leakage in controls increased along with the stress treatments. These results indicate that AtHD2D is positively involved in plant response to different environmental stresses.
A recent study showed that Athd2c T-DNA insertion mutants (hd2c-1 and hd2c-3) were more sensitive to ABA and salt during seed germination (Luo et al., 2012a). Sridha and Wu (2006) showed that while Arabidopsis overexpressing AtHD2C exhibited an increased tolerance to drought and salt stresses, the expression of endogenous AtHD2C was decreased in response to ABA treatment. It would be interesting to investigate if involvement of AtHD2D in stress tolerance is regulated by ABA or by other signals. At this time, the number of studies on the relationship of HD2D as well as other HD2s and ABA are very limited. Along with more research in this area, the relationships of HD2D/HD2s with ABA will be elucidated. Although several HDACs have been shown to involve in plant response to stresses, research also indicates individual HDACs play different roles in plant stress reaction and certain HDACs can interact each other as well as interact with other proteins to exert the full function in stress response pathways in plants (Luo et al., 2012a; Perrella et al., 2013). A study by Luo et al. (2012b) using bimolecular fluorescence complementation (BiFC) assay indicated that HD2D interacted with RPD3 family members HDA6 and HDA19. A recent study by Farhi and Tian showed that HD2D interacted with HD2A and HD2C via BiFC assay (Farhi and Tian, unpublished results). These studies suggest that there might exist a protein complex that modulates gene expression for plant development and plant response to stresses. Indeed, plant growth, especially plant growth and development under environmental stress situations should be a complicated process and could need the function of multiple and related proteins, such as HDACs/HD2s. At this time, the studies only demonstrated there were physical interactions among HDAC proteins, research has not shown the function of the protein interactions yet. Further study should aim to elucidate the relationship of HDACs and HD2s in plant development and in plant response to abiotic stresses.
Genome sequencing of many plant species has provided a fundamental base to study the mechanisms underlining plant life at molecular level. Nevertheless, it has become clear that the DNA sequence itself may not be the determining factor in genetic control of plant life. Epigenetic factors are important in regulating plant phenomena. HD2s are plant specific epigenetic factors that are heavily involved in a number of plant processes. In our study with HD2D gene sequence and protein composition analyses showed that HD2D is less related to the other HD2 genes. HD2D is involved in the control of plant development including seed germination, root development and flowering. Our research further showed that AtHD2D is also involved in plant responses to environmental stresses, including drought, cold, and salt stimuli. Recent studies have showed several HDAC and HD2 proteins interacted each other in BiFC assay (Luo et al., 2012a,b), indicating certain HADC/HD2s may coordinate to function in response to stress. Further research, especially on the aspect of protein interaction may elucidate the biological mechanisms of HD2D in plants, and that knowledge will be used to manipulate gene expression to increase plant resistance to environmental stresses.
Author Contributions
ZH Conceived and designed the experiments then performed the experiments and analyzed the data, finally wrote the paper. HY Designed the experiments then performed the experiments; analyzed the data, finally wrote the paper. ZZ Conceived and designed the experiments and wrote the paper. DH Conceived and designed the experiments and wrote and revised the paper. XL Performed the experiments and analyzed the data; JD Conceived and designed the experiments. LT Conceived and designed the experiments and wrote and revised the paper.
Funding
This work was partially supported by China Scholarship Council, National Natural Science Foundation of China (31400233), Program of Basal Scientific Research and Innovation Science, and Northwest A&F University of China (2014YB035)
Conflict of Interest Statement
The authors declare that the research was conducted in the absence of any commercial or financial relationships that could be construed as a potential conflict of interest.
The reviewer (AM) and Handling Editor declared their shared affiliation, and the Handling Editor states that the process met the standards of a fair and objective review.
Acknowledgments
We would like to thank K. Wu and L. An for research discussion, D. Yang, S. Sawhney, and S. Sibbald for technical help. We thank three reviewers for their critical reading on the manuscript and for constructive comments for manuscript improvement.
Supplementary Material
The Supplementary Material for this article can be found online at: http://journal.frontiersin.org/article/10.3389/fpls.2016.00310
References
Aravind, L., and Koonin, E. V. (1998). Second family of histone deacetylases. Science 280, 1167–1167. doi: 10.1126/science.280.5367.1167a
Bastow, R., Mylne, J. S., Lister, C., Lippman, Z., Martienssen, R. A., and Dean, C. (2004). Vernalization requires epigenetic silencing of FLC by histone methylation. Nature 427, 164–167. doi: 10.1038/nature02269
Berger, S. L. (2002). Histone modifications in transcriptional regulation. Curr. Opin. Genet. Dev. 12, 142–148. doi: 10.1016/S0959-437X(02)00279-4
Bourque, S., Dutartre, A., Hammoudi, V., Blanc, S., Dahan, J., Jeandroz, S., et al. (2011) Type-2 histone deacetylases as new regulators of elicitor-induced cell death in plants. New Phytol. 192, 127–139. doi: 10.1111/j.1469-8137.2011.03788.x
Campos, P. S., and Nunes, M. A. (2003). Electrolyte leakage and lipid degradation account for cold sensitivity in leaves of Coffea sp. plants. J. Plant Physiol. 160, 283–292. doi: 10.1078/0176-1617-00833
Chua, Y. L., Channelière, S., Mott, E., and Gray, J. C. (2005). The bromo domain protein GTE6 controls leaf development in Arabidopsis by histone acetylation at ASYMMETRIC LEAVES1. Genes Dev. 19, 2245–2254. doi: 10.1101/gad.352005
Clough, S. J., and Bent, A. F. (1998). Floral dip: a simplified method for Agrobacterium-mediated transformation of Arabidopsis thaliana. Plant J. 16, 735–743. doi: 10.1046/j.1365-313x.1998.00343.x
Czechowski, T., Bari, R. P., Stitt, M., Scheible, W. R., and Udvardi, M. K. (2004). Realtime RT-PCR profiling of over 1400 Arabidopsis transcription factors: unprecedented sensitivity reveals novel root and shoot specific genes. Plant J. 38, 366–379. doi: 10.1111/j.1365-313X.2004.02051.x
Dangl, M., Brosch, G., Haas, H., Loidl, P., and Lusser, A. (2001). Comparative analysis of HD2 type histone deacetylases in higher plants. Planta 213, 280–285. doi: 10.1007/s004250000506
Deal, R. B., Topp, C. N., McKinney, E. C., and Meagher, R. B. (2007). Repression of flowering in Arabidopsisrequires activation of FLOWERING LOCUS C expression by the histone variant H2A. Z. Plant Cell 19, 74–83. doi: 10.1105/tpc.106.048447
Deng, W., Liu, C., Pei, Y., Deng, X., Niu, L., and Cao, X. (2007). Involvement of the histone acetyltransferase AtHAC1 in the regulation of flowering time via repression of FLOWERING LOCUS C in Arabidopsis. Plant Physiol. 143, 1660–1668. doi: 10.1104/pp.107.095521
Grandperret, V., Nicolas-Francès, V., Wendehenne, D., and Bourque, S. (2014). Type-II histone deacetylases: elusive plant nuclear signal transducers. Plant Cell Environ. 37, 1259–1269. doi: 10.1111/pce.12236
Guitton, A. E., and Berger, F. (2005). Loss of function of MULTICOPY SUPPRESSOR OF IRA1 produces nonviable parthenogenetic embryos in Arabidopsis. Curr. Biol. 15, 750–754. doi: 10.1016/j.cub.2005.02.066
Han, Z., Hunter, D. M., Sibbald, S., Zhang, J., and Tian, L. (2013). Biological activity of the tzs gene of nopaline Agrobacterium tumefaciens GV3101 in plant regeneration and genetic transformation. Mol. Plant Microbe Interact. 26, 1359–1365. doi: 10.1094/MPMI-04-13-0106-R
He, Y., and Amasino, R. M. (2005). Role of chromatin modification in flowering-time control. Trends Plant Sci. 10, 30–35. doi: 10.1016/j.tplants.2004.11.003
Hollender, C., and Liu, Z. (2008). Histone deacetylase genes in Arabidopsis development. J. Integr. Plant Biol. 50, 875–885. doi: 10.1111/j.1744-7909.2008.00704.x
Kim, J. M., Sasaki, T., Ueda, M., Sako, K., and Seki, M. (2015). Chromatin changes in response to drought, salinity, heat, and cold stresses in plants. Front. Plant Sci. 6:114. doi: 10.3389/fpls.2015.00114
Kim, J. M., To, T. K., Nishioka, T., and Seki, M. (2010). Chromatin regulation functions in plant abiotic stress responses. Plant Cell Environ. 33, 604–611. doi: 10.1111/j.1365-3040.2009.02076.x
Köhler, C., and Makarevich, G. (2006). Epigenetic mechanisms governing seed development in plants. EMBO Rep. 7, 1223–1227. doi: 10.1038/sj.embor.7400854
Krogan, N. T., Hogan, K., and Long, J. A. (2012). APETALA2 negatively regulates multiple floral organ identity genes in Arabidopsis by recruiting the co-repressor TOPLESS and the histone deacetylase HDA19. Development 139, 4180–4190. doi: 10.1242/dev.085407
Kuang, J. F., Chen, J. Y., Luo, M., Wu, K. Q., Sun, W., Jiang, Y. M., et al. (2012). Histone deacetylase HD2 interacts with ERF1 and is involved in longan fruit senescence. J. Exp. Bot. 63, 441–454. doi: 10.1093/jxb/err290
Lagacé, M., Chantha, S. C., Major, G., and Matton, D. P. (2003). Fertilization induces strong accumulation of a histone deacetylase (HD2) and of other chromatin-remodeling proteins in restricted areas of the ovules. Plant Mol. Biol. 53, 759–769. doi: 10.1023/B:PLAN.0000023665.36676.89
Lawrence, R. J., Earley, K., Pontes, O., Silva, M., Chen, Z. J., Neves, N., et al. (2004). A concerted DNA methylation/histone methylation switch regulates rRNA gene dosage control and nucleolar dominance. Mol. Cell 13, 599–609. doi: 10.1016/S1097-2765(04)00064-4
Lee, B. H., Henderson, D. A., and Zhu, J. K. (2005). The Arabidopsis cold-responsive transcriptome and its regulation by ICE1. Plant Cell 17, 3155–3175. doi: 10.1105/tpc.105.035568
Liu, X., Yang, S., Zhao, M., Luo, M., Yu, C. W., Chen, C. Y., et al. (2014). Transcriptional repression by histone deacetylases in plants. Mol. Plant 7, 764–772. doi: 10.1093/mp/ssu033
Long, J. A., Ohno, C., Smith, Z. R., and Meyerowitz, E. M. (2006). TOPLESS regulates apical embryonic fate in Arabidopsis. Science 312, 1520–1523. doi: 10.1126/science.1123841
Luo, M., Tai, R., Yu, C. W., Yang, S., Chen, C. Y., Lin, W. D., et al. (2015). Regulation of flowering time by the histone deacetylase HDA5 in Arabidopsis. Plant J. 82, 925–936. doi: 10.1111/tpj.12868
Luo, M., Wang, Y., Liu, X., Yang, S., and Wu, K. (2012b). HD2 proteins interact with RPD3-type histone deacetylases. Plant Signal Behav. 7, 608–610 doi: 10.4161/psb.20044
Luo, M., Wang, Y. Y., Liu, X., Yang, S., Lu, Q., Cui, Y., et al. (2012a). HD2C interacts with HDA6 and is involved in ABA and salt stress response in Arabidopsis. J. Exp. Bot. 63, 3297–3306. doi: 10.1093/jxb/ers059
Lusser, A., Brosch, G., Loidl, A., Haas, H., and Loidl, P. (1997). Identification of maize histone deacetylase HD2 as an acidic nucleolar phosphoprotein. Science 277, 88–91. doi: 10.1126/science.277.5322.88
Murashige, T., and Skoog, F. (1962). A revised medium for rapid growth and bio assays with tobacco tissue cultures. Physiol. Plant. 15, 473–497. doi: 10.1111/j.1399-3054.1962.tb08052.x
Nguyen, H. N., Kim, J. H., Jeong, C. Y., Hong, S. W., and Lee, H. (2013). Inhibition of histone deacetylation alters Arabidopsis root growth in response to auxin via PIN1 degradation. Plant Cell Rep. 32, 1625–1636. doi: 10.1007/s00299-013-1474-6
Pandey, R., Müller, A., Napoli, C. A., Selinger, D. A., Pikaard, C. S., Richards, E. J., et al. (2002). Analysis of histone acetyltransferase and histone deacetylase families of Arabidopsis thaliana suggests functional diversification of chromatin modification among multicellular eukaryotes. Nucleic Acids Res. 30, 5036–5055. doi: 10.1093/nar/gkf660
Perrella, G., Lopez-Vernaza, M. A., Carr, C., Sani, E., Gosselé, V., Verduyn, C., et al. (2013). Histone deacetylase complex1 expression level titrates plant growth and abscisic acid sensitivity in Arabidopsis. Plant Cell 25, 3491–3505. doi: 10.1105/tpc.113.114835
Pi, L., Aichinger, E., Graaff, E., Llavata-Peris, C. I., Weijers, D., Hennig, L., et al. (2015). Organizer-derived WOX5 signal maintains root columellastem cells through chromatin-mediated repression of CDF4 expression. Dev. Cell. 33, 576–588. doi: 10.1016/j.devcel.2015.04.024
Reyes, A., Mezzina, M., and Gadaleta, G. (2002). Human mitochondrial transcription factor A (mtTFA): gene structure and characterization of related pseudogenes. Gene 291, 223–232. doi: 10.1016/S0378-1119(02)00600-5
Rolny, N., Costa, L., Carrión, C., and Guiamet, J. J. (2011). Is the electrolyte leakage assay an unequivocal test of membrane deterioration during leaf senescence? Plant Physiol. Biochem. 49, 1220–1227. doi: 10.1016/j.plaphy.2011.06.010
Rossi, V., Locatelli, S., Varotto, S., Donn, G., Pirona, R., Henderson, D. A., et al. (2007). Maize histone deacetylase hda101 is involved in plant development, gene transcription, and sequence-specific modulation of histone modification of genes and repeats. Plant Cell 19, 1145–1162. doi: 10.1105/tpc.106.042549
Sheldon, C. C., Burn, J. E., Perez, P. P., Metzger, J., Edwards, J. A., Peacock, W. J., et al. (1999). The FLF MADS box gene: a repressor of flowering in Arabidopsis regulated by vernalization and methylation. Plant Cell 11, 445–458. doi: 10.1105/tpc.11.3.445
Sheldon, C. C., Rouse, D. T., Finnegan, E. J., Peacock, W. J., and Dennis, E. S. (2000). The molecular basis of vernalization: the central role of FLOWERING LOCUS C (FLC). Proc. Natl. Acad. Sci. U.S.A. 97, 3753–3758. doi: 10.1073/pnas.97.7.3753
Song, C. P., Agarwal, M., Ohta, M., Guo, Y., Halfter, U., Wang, P., et al. (2005). Role of an Arabidopsis AP2/EREBP-type transcriptional repressor in abscisic acid and drought stress responses. Plant Cell 17, 2384–2396. doi: 10.1105/tpc.105.033043
Sparkes, I. A., Runions, J., Kearns, A., and Hawes, C. (2006). Rapid, transient expression of fluorescent fusion proteins in tobacco plants and generation of stably transformed plants. Nat. Protoc. 1, 2019–2025. doi: 10.1038/nprot.2006.286
Sridha, S., and Wu, K. (2006). Identification of AtHD2C as a novel regulator of abscisic acid responses in Arabidopsis. Plant J. 46, 124–133. doi: 10.1111/j.1365-313X.2006.02678.x
Stockinger, E. J., Mao, Y., Regier, M. K., Triezenberg, S. J., and Thomashow, M. F. (2001). Transcriptional adaptor and histone acetyltransferase proteins in Arabidopsis and their interactions with CBF1, a transcriptional activator involved in cold-regulated gene expression. Nucleic Acids Res. 29, 1524–1533. doi: 10.1093/nar/29.7.1524
Sung, S., and Amasino, R. M. (2004). Vernalization in Arabidopsisthaliana is mediated by the PHD finger protein VIN3. Nature 427, 159–164. doi: 10.1038/nature02195
Tai, H. H., Tai, G. C., and Beardmore, T. (2005). Dynamic histone acetylation of late embryonic genes during seed germination. Plant Mol. Biol. 59, 909–925. doi: 10.1007/s11103-005-2081-x
Taunton, J., Hassig, C. A., and Schreiber, S. L. (1996). A mammalian histone deacetylase related to the yeast transcriptional regulator Rpd3p. Science 272, 408–411. doi: 10.1126/science.272.5260.408
Tian, L., and Chen, Z. J. (2001). Blocking histone deacetylation in Arabidopsis induces pleiotropic effects on plant gene regulation and development. Proc. Natl. Acad. Sci. U.S.A. 98, 200–205. doi: 10.1073/pnas.98.1.200
Van Zanten, M., Zöll, C., Wang, Z., Philipp, C., Carles, A., Li, Y., et al. (2014). HISTONE DEACETYLASE 9 represses seedling traits in Arabidopsis thaliana dry seeds. Plant J. 80, 475–488. doi: 10.1111/tpj.12646
Vlachonasios, K. E., Thomashow, M. F., and Triezenberg, S. J. (2003). Disruption mutations of ADA2b and GCN5 transcriptional adaptor genes dramatically affect Arabidopsis growth, development, and gene expression. Plant Cell 15, 626–638. doi: 10.1105/tpc.007922
Wang, M., Qi, M., and Cutler, A. J. (1993). A simple method of preparing plant samples for PCR. Nucleic Acids Res. 21, 4153–4154. doi: 10.1093/nar/21.17.4153
Wickramasuriya, A. M., and Dunwell, J. M. (2015). Global scale transcriptome analysis of Arabidopsis embryogenesis in vitro. BMC Genomics 16:301. doi: 10.1186/s12864-015-1504-6
Wu, K., Tian, L., Malik, K., Brown, D., and Miki, B. (2000). Functional analysis of HD2 histone deacetylase homologues in Arabidopsis thaliana. Plant J. 22, 19–27. doi: 10.1046/j.1365-313x.2000.00711.x
Wu, K., Tian, L., Zhou, C., Brown, D., and Miki, B. (2003). Repression of gene expression by Arabidopsis HD2 histone deacetylases. Plant J. 34, 241–247. doi: 10.1046/j.1365-313X.2003.01714.x
Yang, P., Zhang, F., Luo, X., Zhou, Y., and Xie, J. (2015). Histone deacetylation modification participates in the repression of peanut (Arachis hypogaea L.) seed storage protein gene Ara h 2.02 during germination. Plant Biol. 17, 522–527. doi: 10.1111/plb.12268
Yano, R., Takebayashi, Y., Nambara, E., Kamiya, Y., and Seo, M. (2013). Combining association mapping and transcriptomics identify HD2B histone deacetylase as a genetic factor associated with seed dormancy in Arabidopsis thaliana. Plant J. 74, 815–828. doi: 10.1111/tpj.12167
Yu, C. W., Liu, X., Luo, M., Chen, C., Lin, X., Tian, G., et al. (2011). HISTONE DEACETYLASE6 interacts with FLOWERING LOCUS D and regulates flowering in Arabidopsis. Plant Physiol. 156, 173–184. doi: 10.1104/pp.111.174417
Zhang, H., Jiang, Y., He, Z., and Ma, M. (2005). Cadmium accumulation and oxidative burst in garlic (Alliumsativum). J. Plant Physiol. 162, 977–984. doi: 10.1016/j.jplph.2004.10.001
Zhao, J., Zhang, J., Zhang, W., Wu, K., Zheng, F., Tian, L., et al. (2015). Expression and function analysis of plant specific histone deacetylase HDT701 in rice. Front. Plant Sci. 5, 764. doi: 10.3389/fpls.2014.00764
Zhao, L., Lu, J., Zhang, J., Wu, P. Y., Yang, S., and Wu, K. (2014). Identification and characterization of histone deacetylases in tomato (Solanum lycopersicum). Front. Plant Sci. 5:760. doi: 10.3389/fpls.2014.00760
Keywords: AtHD2D, expression and function, protein sub-cellular localization, plant development, stress tolerance
Citation: Han Z, Yu H, Zhao Z, Hunter D, Luo X, Duan J and Tian L (2016) AtHD2D Gene Plays a Role in Plant Growth, Development, and Response to Abiotic Stresses in Arabidopsis thaliana. Front. Plant Sci. 7:310. doi: 10.3389/fpls.2016.00310
Received: 20 September 2015; Accepted: 29 February 2016;
Published: 31 March 2016.
Edited by:
Ariel Orellana, Universidad Andrés Bello, ChileReviewed by:
Woei-Jiun Guo, National Cheng Kung University, TaiwanAdrian A. Moreno, Universidad Andrés Bello, Chile
Jesus Vicente-Carbajosa, Polytechnic University of Madrid, Spain
Copyright © 2016 Han, Yu, Zhao, Hunter, Luo, Duan and Tian. This is an open-access article distributed under the terms of the Creative Commons Attribution License (CC BY). The use, distribution or reproduction in other forums is permitted, provided the original author(s) or licensor are credited and that the original publication in this journal is cited, in accordance with accepted academic practice. No use, distribution or reproduction is permitted which does not comply with these terms.
*Correspondence: Zhong Zhao, zhaozh@nwsuaf.edu.cn;
Lining Tian, lining.tian@agr.gc.ca
†These authors have contributed equally to this work.