- 1Plant Molecular Biology Laboratory, Department of Biology, Faculty of Sciences, University of Chile, Santiago, Chile
- 2Centre for Research in Agricultural Genomics, Consejo Superior de Investigaciones Científicas–Institut de Recerca i Tecnologia Agroalimentàries–Universitat Autònoma de Barcelona–Universitat de Barcelon, Barcelona, Spain
Carotenoids and chlorophylls are photosynthetic pigments synthesized in plastids from metabolic precursors provided by the methylerythritol 4-phosphate (MEP) pathway. The first two steps in the MEP pathway are catalyzed by the deoxyxylulose 5-phosphate synthase (DXS) and reductoisomerase (DXR) enzymes. While DXS has been recently shown to be the main flux-controlling step of the MEP pathway, both DXS and DXR enzymes have been proven to be able to promote an increase in MEP-derived products when overproduced in diverse plant systems. Carrot (Daucus carota) produces photosynthetic pigments (carotenoids and chlorophylls) in leaves and in light-exposed roots, whereas only carotenoids (mainly α- and β-carotene) accumulate in the storage root in darkness. To evaluate whether DXS and DXR activities influence the production of carotenoids and chlorophylls in carrot leaves and roots, the corresponding Arabidopsis thaliana genes were constitutively expressed in transgenic carrot plants. Our results suggest that DXS is limiting for the production of both carotenoids and chlorophylls in roots and leaves, whereas the regulatory role of DXR appeared to be minor. Interestingly, increased levels of DXS (but not of DXR) resulted in higher transcript abundance of endogenous carrot genes encoding phytoene synthase, the main rate-determining enzyme of the carotenoid pathway. These results support a central role for DXS on modulating the production of MEP-derived precursors to synthesize carotenoids and chlorophylls in carrot, confirming the pivotal relevance of this enzyme to engineer healthier, carotenoid-enriched products.
Introduction
Many isoprenoids are present in plants and some of them act as primary metabolites with roles in respiration, photosynthesis, and regulation of growth and development. In plastids, the common precursors of all isoprenoid products, the 5-carbon units isopentenyl diphosphate (IPP) and dimethylallyl diphosphate (DMAPP), are produced by the methylerythritol 4-phosphate (MEP) pathway. MEP-derived precursors are used for the synthesis of isoprenoids such as volatiles (monoterpenes, diterpenes, isoprene), hormones (gibberellins, cytokinins, abscisic acid, strigolactones), and photosynthesis-related compounds (carotenoids, chlorophylls, tocopherols, and prenylquinones). Carotenoids are also responsible for the yellow, orange, and red color of non-photosynthetic organs like flowers and fruits, participating in the attraction of pollinators and seed dispersing agents (Grotewold, 2006).
The first step in the MEP pathway, which is catalyzed by the deoxyxylulose 5-phosphate (DXP) synthase (DXS) enzyme, is the formation of DXP from pyruvate and glyceraldehyde 3-phosphate. In the second reaction of MEP pathway the DXP reductoisomerase (DXR) enzyme synthetizes MEP by an intramolecular rearrangement and reduction of DXP. Then, five more enzymes convert MEP (the first intermediate that is specific of this pathway) into IPP and DMAPP (Figure 1) (Rodriguez-Concepcion and Boronat, 2002). Different studies have shown that DXS has a major role in the control of the MEP pathway flux (Rodriguez-Concepcion and Boronat, 2015). A recent work actually showed that DXS displays the highest flux control coefficient of the pathway, i.e., it is the main rate-determining enzyme (Wright et al., 2014). In agreement, overexpression of DXS-encoding genes in different plants typically results in increased levels of plastidial isoprenoids such as carotenoids and chlorophylls (Estevez et al., 2001; Enfissi et al., 2005; Carretero-Paulet et al., 2006; Morris et al., 2006; Munoz-Bertomeu et al., 2006; Zhang et al., 2009; Henriquez et al., 2016). Overexpression of DXR-encoding genes also led to increased levels of MEP-derived isoprenoids in many cases (Mahmoud and Croteau, 2001; Carretero-Paulet et al., 2006; Hasunuma et al., 2008; Yang et al., 2012; Chang et al., 2014; Zhang et al., 2015) but had no effect in others (Mendoza-Poudereux et al., 2014).
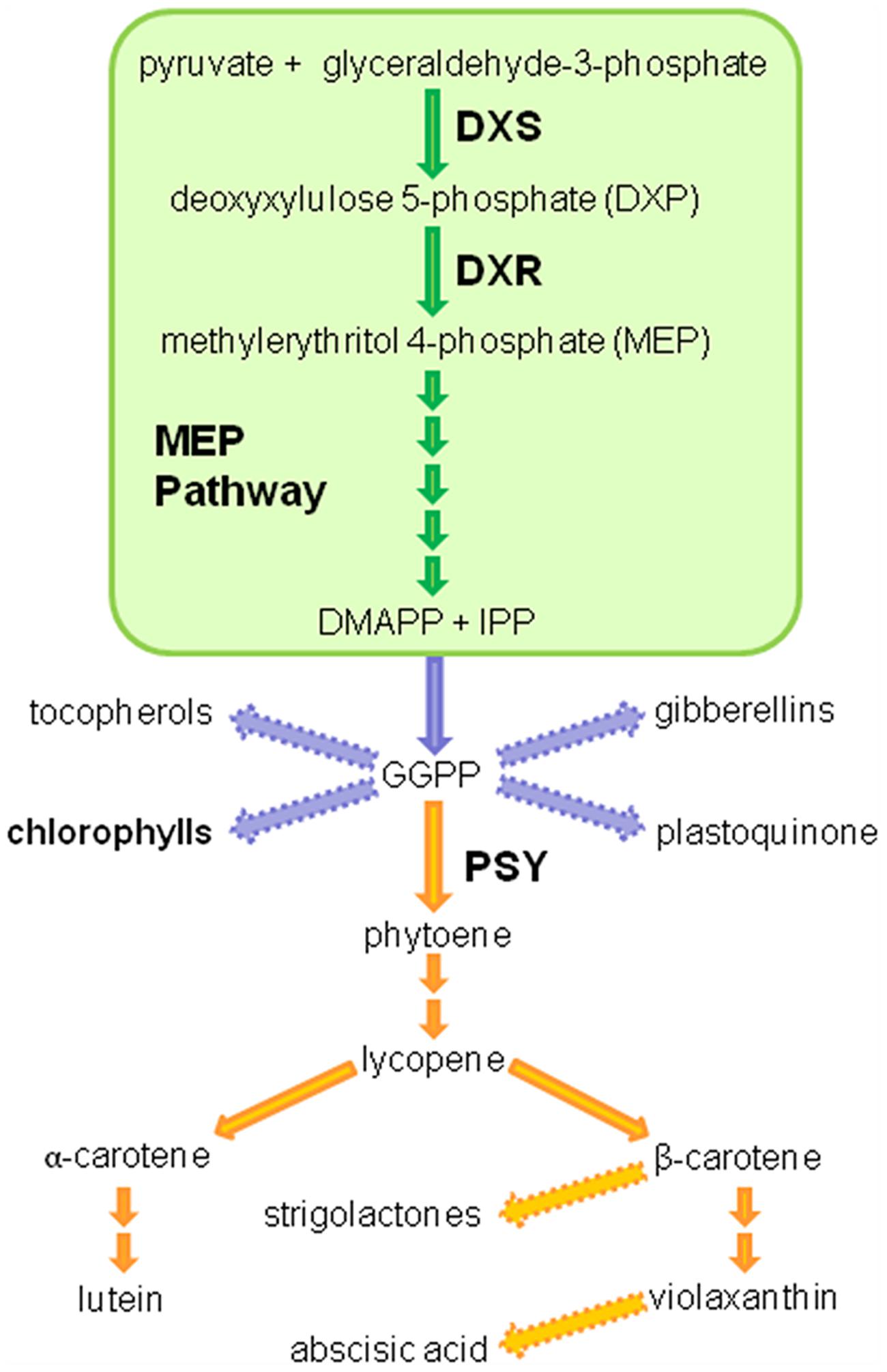
FIGURE 1. Isoprenoid and carotenoid pathway in plants. A simplified schematic representation of the plastidial methylerythritol 4-phosphate (MEP; 2-C-methyl-D-erythritol-4-P) and carotenoid pathways. DXS, deoxyxylulose 5-phosphate synthase; DXR, deoxyxylulose 5-phosphate reductoisomerase; IPP, isopentenyl pyrophosphate; DMAPP, dimethylallyl pyrophosphate; GGPP, geranylgeranyl pyrophosphate; PSY, phytoene synthase.
Like the vast majority of plants, carrot (Daucus carota) produces MEP-derived photosynthetic pigments (chlorophylls and carotenoids) in leaves. Both isoprenoids also accumulate at high levels in the root when this organ is exposed to light (Stange et al., 2008; Fuentes et al., 2012; Rodriguez-Concepcion and Stange, 2013). In the dark, however, the storage root of carrot plants only produces carotenoids but at concentrations that are unique among plants. At present, diverse carrot varieties with different carotenoid composition in the storage root exist (Surles et al., 2004; Rodriguez-Concepcion and Stange, 2013; Stange and Rodriguez-Concepcion, 2015). Orange carrots, the most consumed cultivars, get their coloration due to a massive accumulation of carotenoids, principally α- and β-carotene (Surles et al., 2004). The synthesis of carotenoids begins with the addition of three IPP molecules to one DMAPP molecule to produce C20 geranylgeranyl diphosphate (GGPP) by the enzyme GGPP synthase. The condensation of two GGPP molecules catalyzed by the phytoene synthase (PSY) enzyme leads to the production of C40 phytoene in the first committed step of the carotenoid biosynthetic pathway (Figure 1). Although it is widely accepted that the production of phytoene represents the first and main rate-limiting step in the biosynthesis of carotenoids (Fraser et al., 2002; Lu and Li, 2008; Maass et al., 2009; Rodriguez-Villalon et al., 2009a; Ruiz-Sola and Rodriguez-Concepcion, 2012), work in model systems such as Arabidopsis thaliana and tomato (Solanum lycopersicum) has led to propose that the limiting nature of PSY activity largely depends on the availability of the metabolic precursors synthesized in the MEP pathway (Lois et al., 2000; Enfissi et al., 2005; Carretero-Paulet et al., 2006; Rodriguez-Villalon et al., 2009a).
While DXR is often encoded by a single gene in plants, DXS is typically encoded by small gene families with members of at least two functionally specialized classes (Walter et al., 2002; Cordoba et al., 2009; Rodriguez-Concepcion and Boronat, 2015). Type I DXS enzymes supply the precursors for housekeeping and photosynthetic isoprenoids, including chlorophylls and carotenoids, whereas type II isoforms are usually specialized in the production of secondary isoprenoids. A third type of DXS-like sequences is usually present in plant genomes but their enzymatic role is still unclear (Rodriguez-Concepcion and Boronat, 2015). In carrot, one gene for DXR (DCAR_026133) and four DXS-encoding genes (type I DCAR_030576, type II DCAR_009911 and DCAR_014178, and type III DCAR_022887) were recently annotated (Iorizzo et al., 2016). Interestingly, only the expression of the type I DXS gene was correlated with high carotenoid content (Iorizzo et al., 2016). In this work, we aimed to experimentally evaluate the role of DXS and DXR activities in regulating the production of MEP-derived isoprenoids in leaves and storage roots. Because carrot genes encoding these enzymes have only recently become available (Iorizzo et al., 2016), we used the corresponding Arabidopsis DXS/CLA1 (type I) and DXR genes cloned in plant expression vectors under the control of the constitutive 35S promoter (Pulido et al., 2013; Perello et al., 2016). Analysis of the generated transgenic carrot lines confirmed a limiting role for DXS in the production of both carotenoids and chlorophylls in leaves and roots, whereas DXR appeared to only marginally affect the production of these plastidial isoprenoids in leaves. Most interestingly, DXS overexpression led to increased levels of PSY-encoding transcripts, highlighting the central role of these two enzymes for the control of carotenoid biosynthesis in plants.
Materials and Methods
Plant Material
Seeds of commercially acquired carrot (Daucus carota L.) cultivar Nantaise were surface sterilized in a solution of 95% ethanol for 1 min and washed once with sterile water for 3 min. Then, the seeds were incubated under agitation in a solution of sodium hypochlorite (2.62% v/v) for 45 min, washed three times with sterile water and finally dried on sterile absorbent paper. The sterile seeds were deposited in sterile flasks with solid MS medium (Murashige and Skoog, 1962) supplemented with 0.44% vitamins, 2% sucrose, 0.01% myo-inositol, 0.7% Agar and pH adjusted to 5.8. The seeds were kept in a growth chamber 3 weeks with a 16 h long day photoperiod illuminated with cool-white fluorescent light (115 μmol m-2 s-1) at 22°C. Hypocotyls and stems of 3-week-old in vitro wild-type carrot plantlets were utilized in Agrobacterium-mediated transformation experiments. Transformed carrots were transferred to pots (20 × 10) and cultivated in the greenhouse, as described above.
Agrobacterium tumefaciens-Mediated Transformation of Daucus carota
Binary vectors for constitutive expression of Arabidopsis genes encoding DXS or DXR proteins fused to GFP were previously reported (Pulido et al., 2013; Perello et al., 2016). Agrobacterium tumefaciens (strain GV3101) cells were transformed with these vectors and used for D. carota transformation following the protocol described by (Chen and Punja, 2002). Briefly, hypocotyl segments of 3 weeks-old seedlings were co-cultivated with Agrobacterium carrying the vector of interest, and placed on solidified MS media (4.4 g/L MS salts, 20 g/L sucrose and 0.7% agar) in darkness. After 2 days, the explants were transferred to solid MS medium containing 1 mg/L 2.4D for somatic embryogenesis induction and supplemented with 0.5 mg/L Basta® and 300 mg/L cefotaxime. After 4 weeks in darkness, the explants were placed on solidified MS medium containing 0.5 mg/L 2.4D, 1 mg/L Basta® and 300 mg/L cefotaxime in photoperiod conditions (16 h light, 115 μmol/m2/s). Herbicide-resistant embryos were transferred to MS media in the absence of hormones to induce the development of shoots. After 6 months, transformed plantlets were transferred to soil in a temperature and photoperiod controlled greenhouse (16 h light, 115 μmol/m2/s). Different stages of the procedure are shown in Supplementary Figure S1. We obtained several lines per construct and PCR analyses to confirm the presence of the transgenes in the transgenic lines were performed with primers AtDXRF, AtDXSF, and eGFPR (Supplementary Figure S2; Supplementary Table S1). Transgenic lines developed normally and were visually undistinguishable from non-transgenic controls (Supplementary Figure S3). Four of the lines confirmed to contain the corresponding transgene by PCR (Supplementary Figures S2 and S3) were then picked for quantification of transcript and pigment levels. From those, three representative lines per construct were selected for more detailed analysis.
Pigment Extraction and High Performance Liquid Chromatography (HPLC) Analysis
Photosynthetic pigments from leaves and roots of wild-type and transgenic plants transferred to soil and grown in the greenhouse for 6 months were extracted from 100 mg of fresh weight with 1 ml of hexane/acetone/ethanol (2:1:1 v/v) as described (Fuentes et al., 2012). The extract was dried with N2. To quantify the concentration of chlorophyll a, chlorophyll b and total carotenoids present in the pigment extracts of leaves and roots of D. carota, the pigments extracted were resuspended in 2 mL of acetone and using a spectrophotometer the absorbance was measured at 750, 662, 645, and 470 nm in quartz cuvettes. Absorbance at 662, 645, and 470 nm is used to determine the concentration of chlorophyll a, chlorophyll b and total carotenoids, respectively. Further, the absorbance at 750 nm was measured to determine the turbidity of the sample because the turbid samples may result in underestimation of the concentration of the pigments concentration. With absorbance measurements, the concentration of chlorophyll a, chlorophyll b and total carotenoids determined by the equations described (Lichtenthaler and Buschmann, 2001). For α-carotene and β-carotene measurements, the pigments were separated by a HPLC using a RP-18 Lichrocart125-4 reverse phase column (Merck®), utilizing a acetonitrile: methanol: isopropanol (85:10:5 v/v) mix as a mobile phase with a 1 ml/min flow rate at room temperature in isocratic conditions. The elution spectra of each maximum were obtained using a diode array detector. The carotenoids were identified according to their absorption spectra, retention time and comparison with specific pigment standards, which was corroborated by comparison with the Carotenoids Handbook (Britton, 1995; Britton et al., 2004). All operations were carried out in triplicate, on ice and dark conditions to avoid photodegradation, isomerization and structural changes of carotenoids.
RNA Extraction and Quantitative RT-PCR
A frozen powder of 100 mg of D. carota leaves from plants transferred to soil and grown in the greenhouse for 6 months was used for total RNA extraction using TRIzol® reagent (Invitrogen) and following the manufacturer’s instructions. For cDNA synthesis, 2 μg of total DNA-free RNA was mixed with 1mM of oligodT primer and Impron II reverse transcriptase (Promega). The expression of the DXS and DXR transgenes was estimated by RT-PCR using primers qDXRF, qDXSF and qeGFP R (Supplementary Table S1). Quantitative RT-PCR (qRT) experiments were performed in a Stratagene Mx3000P thermocycler, using SYBR Green double strand DNA binding dye as described previously (Stange et al., 2008). Specific primers for carrot genes were designed targeting the 5′ UTR of PSY1 (AB032797) and PSY2 (DQ192187) and the coding sequence of the 18S gene, selected as the normalizer (Supplementary Table S1). Final data were obtained introducing fluorescence results in the equation described by Pfaffl (2001). Each qRT-PCR reaction was performed with three biological replicates and each sample was analyzed in duplicate (technical replicate). In all cases, the reaction specificities were tested with melting gradient dissociation curves and electrophoresis gels. To test for significant differences in gene expression, results were analyzed using the General Linear Models option in the statistical software package Graphpad Prism. The one and two tailed Student t-test (p < 0.05, confidence interval 95%), were used.
Results
The Constitutive Expression of DXS Increases Carotenoid Levels in D. carota Roots
In order to determine the significance of the supply of MEP-derived metabolic precursors for the synthesis of carotenoids (and chlorophylls) in carrot, we generated transgenic plants expressing GFP-tagged versions of the Arabidopsis DXS/DXS1/CLA1 (At4g15560) or DXR (At5g62790) genes under the control of the constitutive 35S promoter (Supplementary Figures S1 and S2). The presence of the corresponding transgene in the generated S (35S:DXS) and R (35S:DXR) lines was confirmed by PCR (Supplementary Figure S2). After semi-quantitative RT-PCR analyses to estimate transgene expression levels in 6-month-old carrot plants, three representative lines of each construct were selected for further experiments (Figure 2). First, we aimed to quantify the accumulation of carotenoids in the root of transgenic plants and untransformed wild-type (WT) controls. As shown in Figure 3A, the constitutive expression of DXS produced a substantial increment in α-carotene (up to 3.6-fold) and β-carotene (up to 2.7-fold) levels in the storage root. As a consequence, total carotenoids were also significantly higher than in untransformed controls, reaching 2600 μg/g DW (dry weight) in line S29, the one also showing the highest levels of transgene expression (Figure 2). On the other hand, the constitutive expression of DXR only led to a slight increase in β-carotene in line R32 (the one with highest transgene expression levels) but no significant changes in the levels of α-carotene and total carotenoids in the root (Figure 3B). When calculating the mean of all transgenic lines together, the constitutive expression of the DXS gene resulted in an average increase of 114% of α-carotene, 75% of β-carotene, and 78% of total carotenoids with respect to WT plants, whereas DXR overexpression had no impact on root carotenoid levels (Figure 3). These results suggest that DXS, but not DXR, plays an important role in controlling the flow of metabolic precursors toward carotenoid synthesis in the storage root of D. carota plants.
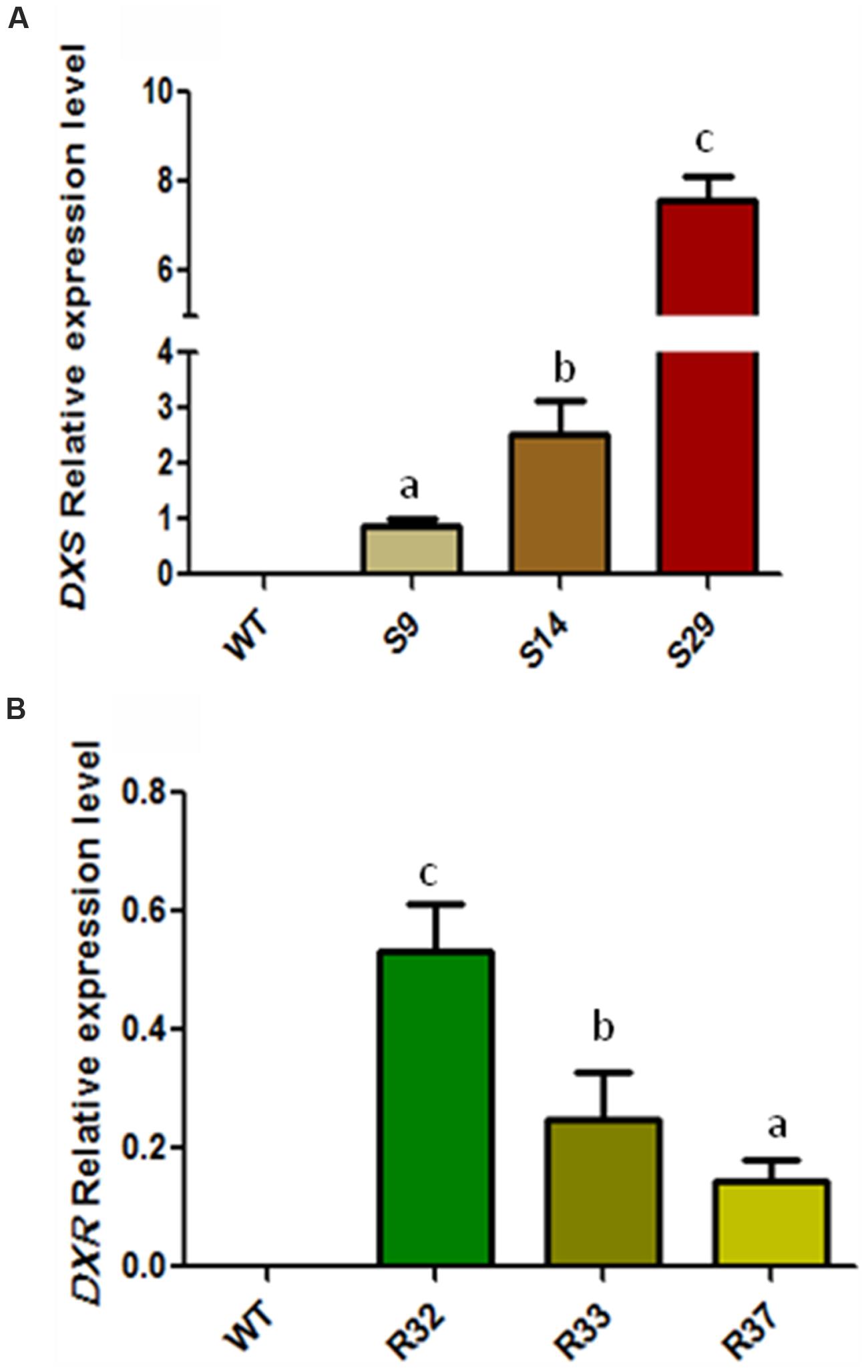
FIGURE 2. Relative transcript expression of AtDXS and AtDXR in leaves of transgenic lines. Relative transcript abundance of (A) AtDXS and (B) AtDXR in representative transgenic carrot lines determined by means of semi quantitative RT-PCR. Assay was carried out in triplicate and normalized to RNAr18S expression. Letters indicate significant differences between transgenic lines determined by one-tailed ANOVA and Tukey post-test, p < 0.05.
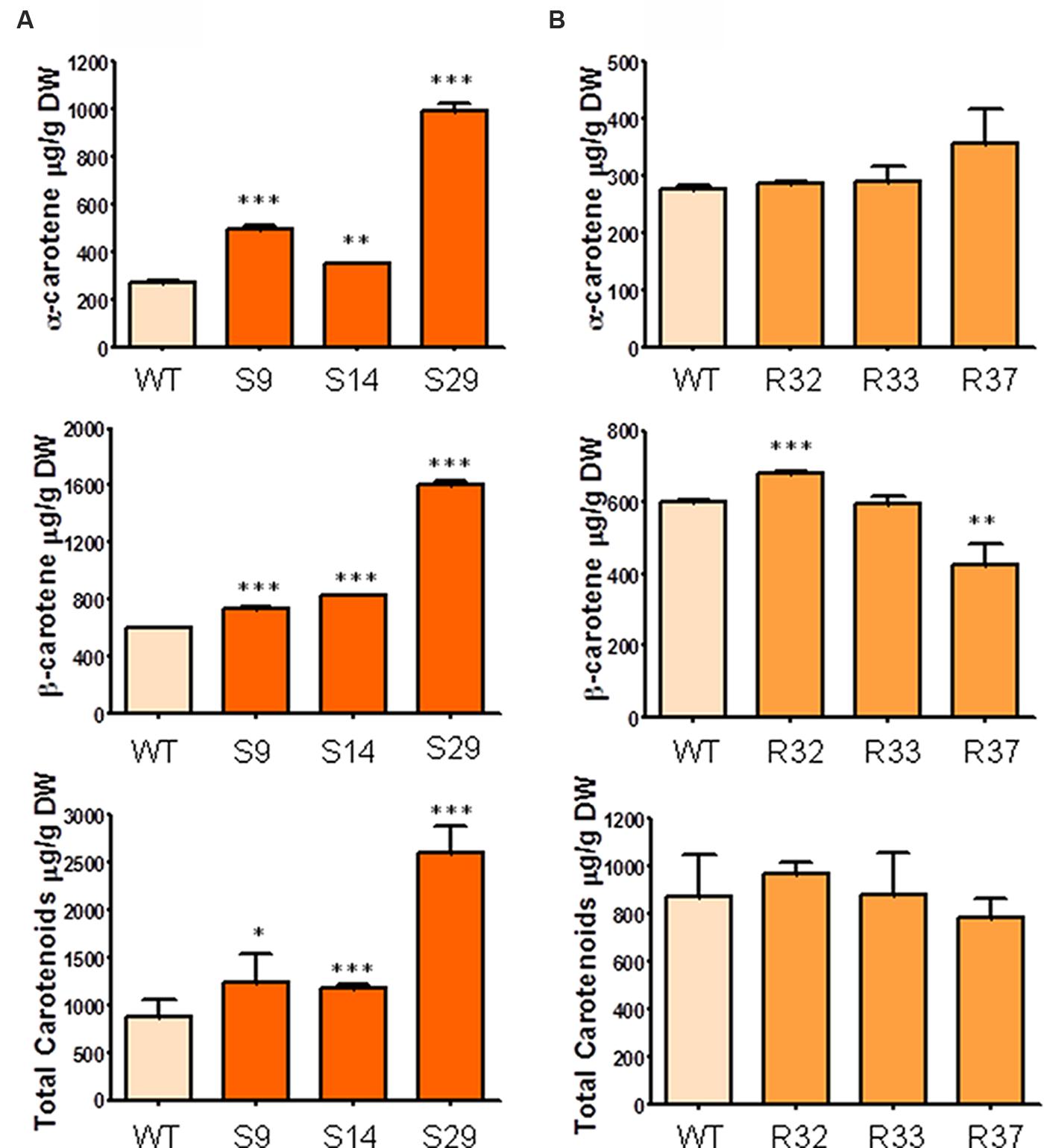
FIGURE 3. Carotenoid composition in storage roots of carrot transgenic lines expressing AtDXS and AtDXR. (A) Quantification of α-carotene, β-carotene and total carotenoids in storage roots of three DXS transgenic lines, (B) Quantification of α-carotene, β-carotene and total carotenoids in the storage root of three DXR transgenic lines. Asterisks indicate significant differences between transgenic lines and wt determined two-tailed unpaired Student’s t-test. ∗p < 0.05; ∗∗p < 0.01; ∗∗∗p < 0.001.
DXS and, to a Lower Extent, DXR Can Influence Carotenoid and Chlorophyll Content in D. carota Leaves
We next asked whether the upregulation of DXS or DXR levels could have a different effect on photosynthetic tissues of carrot plants. To address this question, we quantified the levels of both carotenoids and chlorophylls in leaves of the selected transgenic lines. As shown in Figure 4A, the constitutive overexpression of DXS produced a significant raise in the concentration of total carotenoids. In particular, α- and β-carotene increased in leaves of all lines tested, reaching levels that were up to fourfold and twofold higher, respectively, than those found in untransformed controls. Levels of lutein, however, were only significantly higher in line S29 (Figure 4A). On the other hand, the overexpression of DXR produced modest but statistically significant increments in α-carotene, β-carotene and lutein in leaves of some of the transgenic lines tested, giving rise to a slight increment in total carotenoids in all transgenic lines (Figure 4B). On average, the expression of the Arabidopsis DXS gene in D. carota produced an increase of 91, 69, 30, and 80% in the concentration of α-carotene, β-carotene, lutein and total carotenoids, respectively, while increasing DXR levels only led to statistically significant increments in lutein (17%) and total carotenoids (19%; Figure 4).
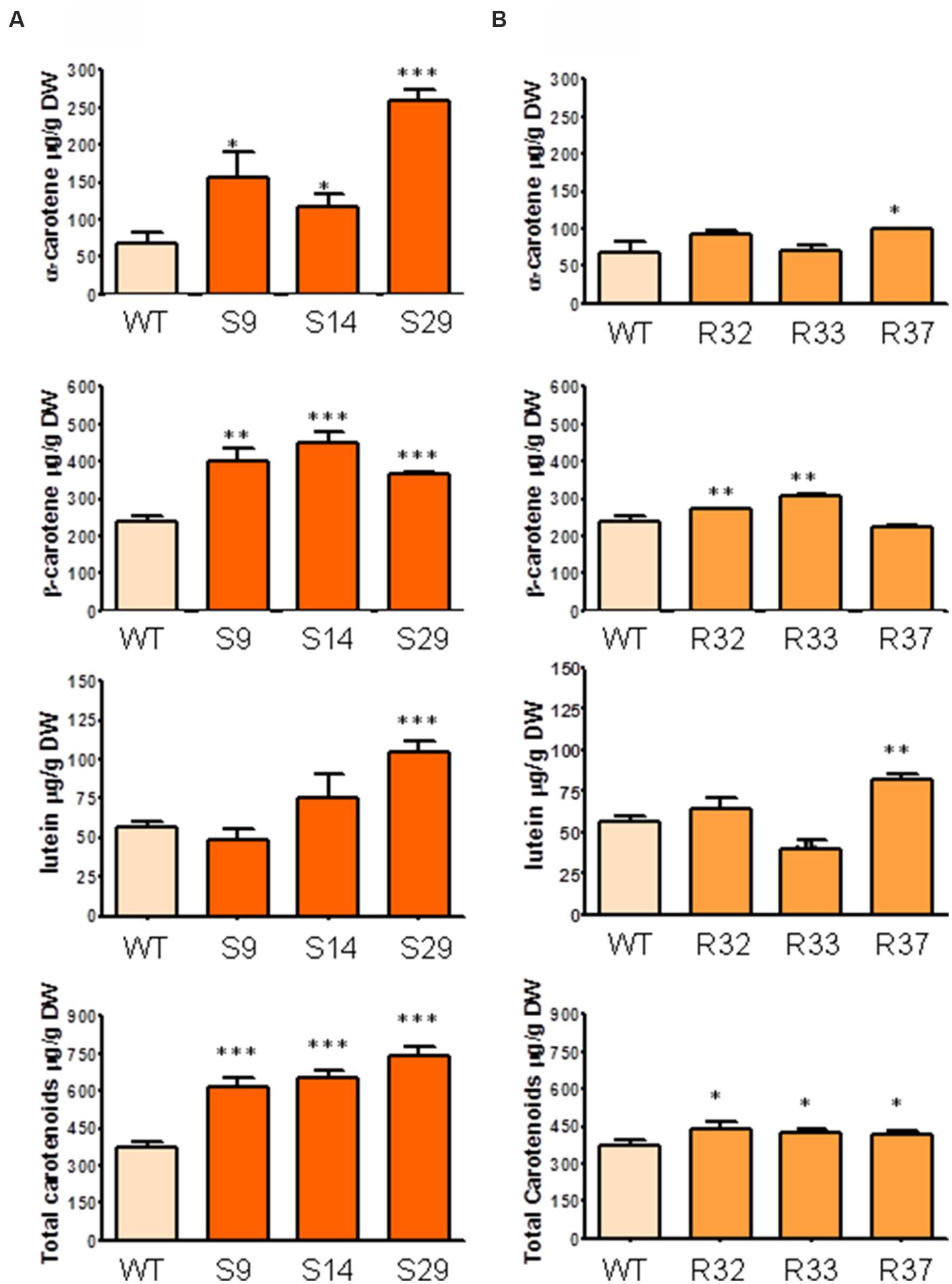
FIGURE 4. Carotenoid composition in leaves of carrot transgenic lines expressing AtDXS and AtDXR. (A) Quantification of α-carotene, β-carotene, lutein and total carotenoids in leaves of three DXS transgenic lines, (B) Quantification of α-carotene, β-carotene, lutein and total carotenoids in leaves of three DXR transgenic lines., Asterisks indicate significant differences between transgenic lines and wt determined by two-tailed unpaired Student’s t-test. ∗p < 0.05; ∗∗p < 0.01; ∗∗∗p < 0.001.
Similar results were found for chlorophylls. Thus, the constitutive overexpression of DXS significantly increased the accumulation of chlorophylls in the leaves of all transgenic lines tested (Figure 5A). In particular, the levels of chlorophyll a in the best performing line (S29) were about twofold higher compared to the WT, whereas a 1.4-fold rise in chlorophyll b levels was detected in the same line (Figure 5A). The overexpression of DXR resulted in only minor changes in chlorophyll levels. While chlorophyll a did not change in any of the lines tested, modest but significant increases in the concentration of chlorophyll b and total chlorophylls were detected in some lines (Figure 5B). On average, increasing DXS activity led to 78% more chlorophyll a, 40% more chlorophyll b and 63% more total chlorophylls in carrot leaves, while increasing DXR activity did not change the levels of chlorophyll a but produced 38% more chlorophyll b and 21% more total chlorophylls compared to untransformed leaves (Figure 5).
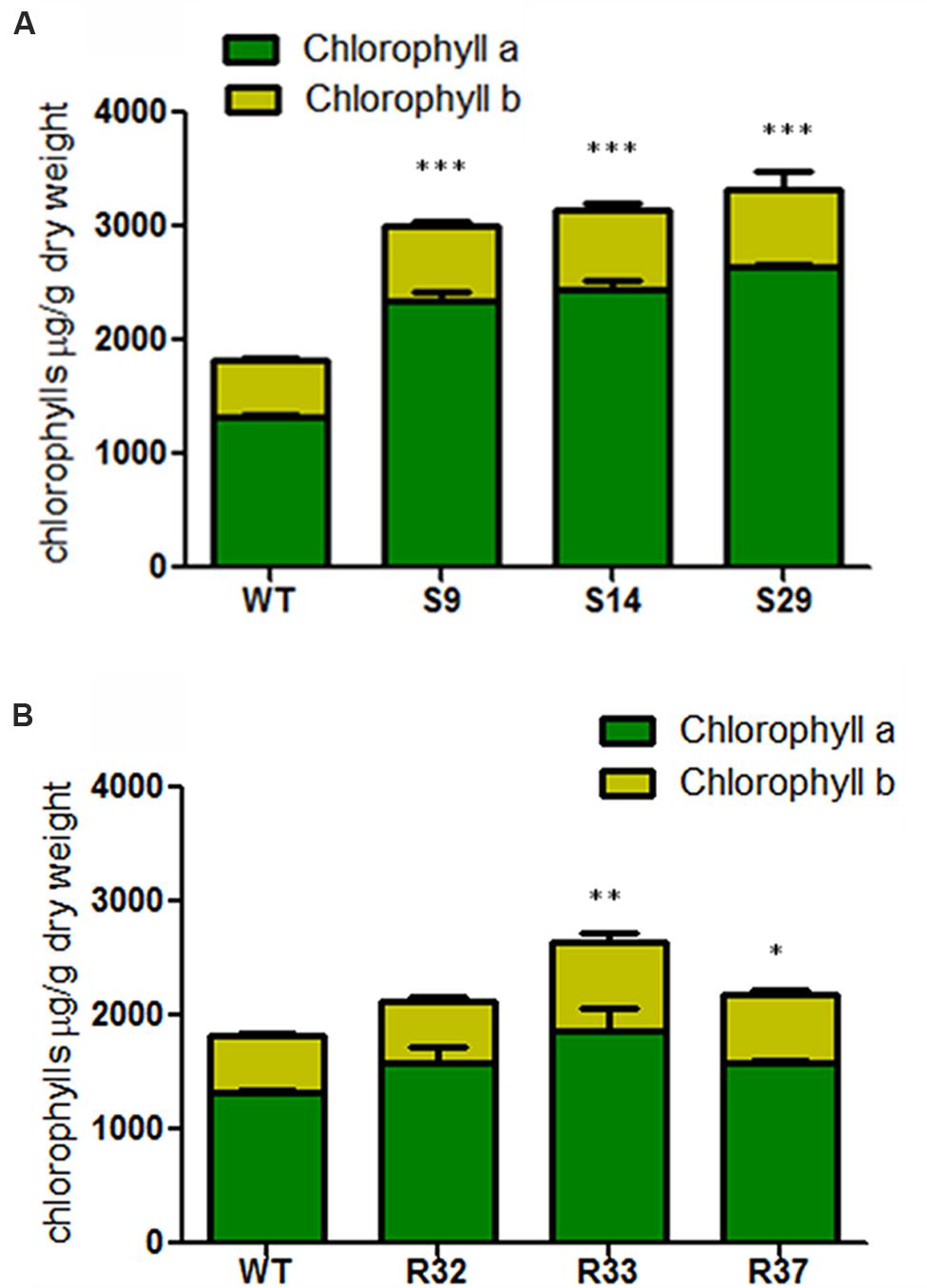
FIGURE 5. Chlorophyll composition in leaves of carrot transgenic lines expressing AtDXS and AtDXR. (A) Quantification of chlorophyll a and chlorophyll b in leaves of three DXS transgenic lines, (B) Quantification of chlorophyll a and chlorophyll b in leaves of three DXR transgenic lines. The sum of both chlorophyll a and chlorophyll b represents the total chlorophylls in wild-type (WT), and transgenic lines, Asterisks indicate significant differences between transgenic lines and wt determined two-tailed unpaired Student’s t-test. ∗p < 0.05; ∗∗p < 0.01; ∗∗∗p < 0.001.
The Constitutive Overexpression of Arabidopsis DXS Increases the Transcript Abundance of PSY-Encoding Carrot Genes in Leaves
While DXS has been shown to be the main rate-determining enzyme of the MEP pathway, PSY plays a similar role in the carotenoid pathway (Fraser et al., 2002; Wright et al., 2014). In fact, an increased DXS activity would only result in increased carotenoid biosynthesis if PSY activity is high enough to channel the extra supply of MEP-derived precursors to the carotenoid pathway. Consistent with the central role of these two enzymes in the control of the carotenoid pathway flux, a recent genome-scale analysis (Iorizzo et al., 2016) showed that carrot genes encoding DXS (DCAR_030576, named DXS1) and PSY (DCAR_023043 and DCAR_010057, respectively, named PSY1 and PSY2) were differentially expressed in storage roots from populations with different carotenoid levels, showing increased transcript levels in accessions with higher carotenoid levels (Supplementary Figure S4). By contrast, no changes were found for the only carrot gene encoding DXR (DCAR_026133). Because transgene-mediated alterations of the carrot carotenoid pathway have been associated with a concomitant alteration of the transcript levels of endogenous PSY1 and PSY2 genes (Moreno et al., 2013), we next analyzed whether the transcript abundance of these two PSY-encoding genes was changed in leaves of representative lines overexpressing the Arabidopsis DXS and DXR genes. As shown in Figure 6A, leaves from transgenic plants overexpressing DXS showed a dramatic increase in the relative abundance of PSY1 transcripts compared to WT controls. A lower increase was observed for PSY2 transcripts (Figure 6A). In general terms, a fairly good correlation was found between levels of exogenous DXS transcripts (Figure 2) and endogenous PSY-encoding gene expression (Figure 6A) in leaves. Unexpectedly, however, we observed that the constitutive overexpression of DXR did not result in higher but lower levels of endogenous PSY1 and PSY2 transcripts in leaves from lines R32 and R37 and no changes in line R33 (Figure 6B).
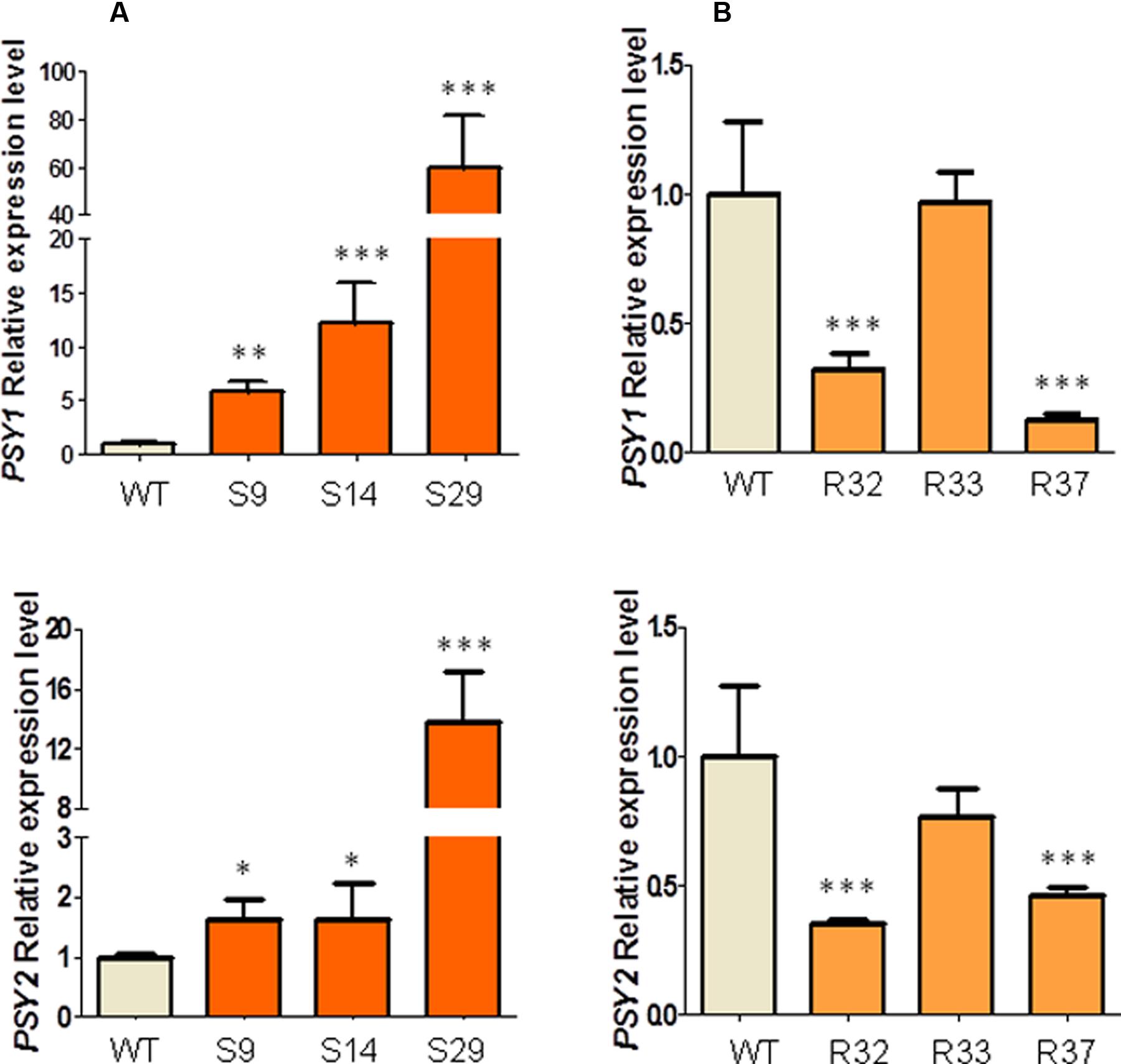
FIGURE 6. Relative transcript expression of DcPSY1 and DcPSY2 in leaves of AtDXS and AtDXR carrot transgenic lines. (A) DcPSY1 and DcPSY2 expression in leaves of three representative DXS transgenic lines. (B) DcPSY1 and DcPSY2 expression in leaves of three representative DXR transgenic lines. Assay was carried out in triplicate and normalized to RNAr18S expression. Expression of WT plants were used as calibrator and settled in 1. Asterisks indicate significant differences between transgenic lines and wt plants determined by two-tailed unpaired Student’s t-test. ∗p < 0.05; ∗∗p < 0.01; ∗∗∗p < 0.001.
Discussion
Different studies, including metabolic control analysis, have shown that DXS is the main rate-determining enzyme of the MEP pathway (Wright et al., 2014; Rodriguez-Concepcion and Boronat, 2015). Consistently, a higher production of MEP-derived isoprenoids has been associated with increased expression of DXS-encoding genes in many plant systems. As indicated above, the carrot genome contains four genes for DXS-like sequences but only the one encoding the type I DXS isoform (DXS1) is expressed in correlation with the carotenoid content of carrot accessions displaying differentially pigmented roots (Iorizzo et al., 2016). While this observation suggested that DXS activity might regulate root carotenoid content, no experimental evidence was provided. Here show that increasing the levels of DXS, but not DXR, indeed results in an enhanced accumulation of carotenoids in dark-grown storage roots of transgenic carrot plants (Figure 3).
Unlike that described for roots, an upregulation of DXR levels in carrot leaves resulted in a modest increase in the total content of carotenoids (Figure 4) but also of other MEP-derived isoprenoids such as chlorophylls (Figure 5). A number of overexpression studies (Mahmoud and Croteau, 2001; Carretero-Paulet et al., 2006; Hasunuma et al., 2008; Yang et al., 2012; Chang et al., 2014; Zhang et al., 2015) support the conclusion that DXR contributes to the control of the MEP pathway flux but typically to a lower extent compared to DXS (Cordoba et al., 2009; Rodriguez-Concepcion, 2010). The increase in the levels of carotenoids and chlorophylls in the leaves of some of our transgenic DXR-overexpressing lines, however, was not a general effect. Moreover, the lack of correlation between transgene expression levels (Figure 2) and the accumulation of carotenoids (Figure 4) and chlorophylls (Figure 5) in transgenic lines suggests that the observed changes of isoprenoid pigment content might not be the direct consequence of altering DXR levels. Our results parallel similar observations in DXR-overexpressing spike lavender (Lavandula latifolia) plants (Mendoza-Poudereux et al., 2014). Together, we conclude that DXS plays a central regulatory function for the production of MEP-derived precursors in most plant systems, including carrot leaves and dark-grown roots, whereas the rate-determining activity of DXR appears not to be a general trend.
Our work further provides compelling evidence that the steps catalyzed by DXS and PSY enzymes represent regulatory nodes that coordinate the MEP pathway and the carotenoid pathway to ensure that the isoprenoid precursors required for carotenoid biosynthesis will be supplied when needed (Lois et al., 2000; Rodriguez-Concepcion et al., 2001; Fraser et al., 2007; Cordoba et al., 2009; Rodriguez-Concepcion, 2010). Increased PSY activity has been found to promote a post-transcriptional accumulation of DXS enzymes in Arabidopsis (Guevara-Garcia et al., 2005; Rodriguez-Villalon et al., 2009b) and DXS activity in tomato (Fraser et al., 2007). A possible mechanism involving the feedback regulation of DXS activity and turnover by IPP and DMAPP contents has been recently proposed to adjust the MEP pathway flux according to product (i.e., carotenoid) demand under normal growth conditions (Pokhilko et al., 2015). On the other hand, increased DXS activity in tomato fruits and potato tubers produces an increment in the expression of PSY-encoding genes (Lois et al., 2000; Morris et al., 2006) similar to that reported here in carrot leaves (Figure 6). Interestingly, a good correlation was found between the expression level of the DXS-encoding transgene (Figure 2) and the upregulation of carrot PSY1 and PSY2 genes (Figure 6) in individual lines, suggesting a direct effect. We speculate that the DXS-mediated induction of endogenous PSY-encoding genes in carrot might contribute to the increased accumulation of carotenoids detected in these lines. The increase in the production of chlorophylls in the leaves of DXS-overexpressing plants might be the consequence of increasing both the supply of MEP derived precursors and the carotenoid-mediated protection against photooxidative damage. However, it is also possible that chlorophyll biosynthetic genes could also be upregulated after increasing DXS activity. Further work should also determine whether other MEP-derived plastidial isoprenoids with roles in photosynthesis such as tocopherols and plastoquinone, and even hormones (Figure 1), are also altered in carrot leaves and roots with increased DXS (or DXR) activity. Because carotenoids (including those with provitamin A activity) are health-promoting metabolites and industrially relevant natural pigments (Fraser et al., 2007; Rodriguez-Concepcion, 2010), promoting their accumulation in plant-derived products by biotechnological methods will contribute to improve their economic and nutritional value
Author Contibutions
KS: performed the experiments (carrot transformation, molecular analysis to select transgenic plants, HPLC quantification, qRT PCR) and wrote the manuscript, LQ: performed experiments (carrot transformation, molecular analysis to select transgenic plants). CS and MR-C: designed experiments and wrote the manuscript.
Funding
This work was funded by the Chilean Regular Fondecyt 1130245 of CS, CSIC 11/12 of CS and MR-C and grants from the Spanish Ministry of Economy and Competitiveness (BIO2014-59092-P) and Generalitat de Catalunya (2014SGR-1434) to MR-C. We also acknowledge the financial support of the Severo Ochoa Programme for Centres of Excellence in R&D 2016-2019 (SEV-2015-0533) to the CRAG.
Conflict of Interest Statement
The authors declare that the research was conducted in the absence of any commercial or financial relationships that could be construed as a potential conflict of interest.
Acknowledgment
Conicyt Scholarships to KS and LQ.
Supplementary Material
The Supplementary Material for this article can be found online at: http://journal.frontiersin.org/article/10.3389/fpls.2016.01344
References
Britton, G. (1995). Structure and properties of carotenoids in relation to function. Faseb J. 9, 1551–1558.
Britton, G., Liaaen-Jensen, H., and Pfander, H. (2004). Carotenoids Handbook. Basel: Birkhauser Verlag.
Carretero-Paulet, L., Cairo, A., Botella-Pavia, P., Besumbes, O., Campos, N., Boronat, A., et al. (2006). Enhanced flux through the methylerythritol 4-phosphate pathway in Arabidopsis plants overexpressing deoxyxylulose 5-phosphate reductoisomerase. Plant Mol. Biol. 62, 683–695. doi: 10.1007/s11103-006-9051-9
Chang, K., Qiu, F., Chen, M., Zeng, L., Liu, X., Yang, C., et al. (2014). Engineering the MEP pathway enhanced ajmalicine biosynthesis. Biotechnol. Appl. Biochem. 61, 249–255. doi: 10.1002/bab.1176
Chen, W. P., and Punja, Z. K. (2002). Transgenic herbicide- and disease-tolerant carrot (Daucus carota L.) plants obtained through Agrobacterium-mediated transformation. Plant Cell Rep. 20, 929–935. doi: 10.1007/s00299-001-0419-7
Cordoba, E., Salmi, M., and Leon, P. (2009). Unravelling the regulatory mechanisms that modulate the MEP pathway in higher plants. J. Exp. Bot. 60, 2933–2943. doi: 10.1093/jxb/erp190
Enfissi, E. M., Fraser, P. D., Lois, L. M., Boronat, A., Schuch, W., and Bramley, P. M. (2005). Metabolic engineering of the mevalonate and non-mevalonate isopentenyl diphosphate-forming pathways for the production of health-promoting isoprenoids in tomato. Plant Biotechnol. J. 3, 17–27. doi: 10.1111/j.1467-7652.2004.00091.x
Estevez, J. M., Cantero, A., Reindl, A., Reichler, S., and Leon, P. (2001). 1-deoxy-D-xylulose-5-phosphate synthase, a limiting enzyme for plastidic isoprenoid biosynthesis in plants. J. Biol. Chem. 276, 22901–22909. doi: 10.1074/jbc.M100854200
Fraser, P. D., Enfissi, E. M., Halket, J. M., Truesdale, M. R., Yu, D., Gerrish, C., et al. (2007). Manipulation of phytoene levels in tomato fruit: effects on isoprenoids, plastids, and intermediary metabolism. Plant Cell 19, 3194–3211. doi: 10.1105/tpc.106.049817
Fraser, P. D., Romer, S., Shipton, C. A., Mills, P. B., Kiano, J. W., Misawa, N., et al. (2002). Evaluation of transgenic tomato plants expressing an additional phytoene synthase in a fruit-specific manner. Proc. Natl. Acad. Sci. U.S.A. 99, 1092–1097. doi: 10.1073/pnas.241374598
Fuentes, P., Pizarro, L., Moreno, J. C., Handford, M., Rodriguez-Concepcion, M., and Stange, C. (2012). Light-dependent changes in plastid differentiation influence carotenoid gene expression and accumulation in carrot roots. Plant Mol. Biol. 79, 47–59. doi: 10.1007/s11103-012-9893-2
Grotewold, E. (2006). The genetics and biochemistry of floral pigments. Annu. Rev. Plant Biol. 57, 761–780. doi: 10.1146/annurev.arplant.57.032905.105248
Guevara-Garcia, A., San Roman, C., Arroyo, A., Cortes, M. E., de la Luz Gutierrez-Nava, M., and Leon, P. (2005). Characterization of the Arabidopsis clb6 mutant illustrates the importance of posttranscriptional regulation of the methyl-D-erythritol 4-phosphate pathway. Plant Cell 17, 628–643. doi: 10.1105/tpc.104.028860
Hasunuma, T., Takeno, S., Hayashi, S., Sendai, M., Bamba, T., Yoshimura, S., et al. (2008). Overexpression of 1-Deoxy-D-xylulose-5-phosphate reductoisomerase gene in chloroplast contributes to increment of isoprenoid production. J. Biosci. Bioeng. 105, 518–526. doi: 10.1263/jbb.105.518
Henriquez, M. A., Soliman, A., Li, G., Hannoufa, A., Ayele, B. T., and Daayf, F. (2016). Molecular cloning, functional characterization and expression of potato (Solanum tuberosum) 1-deoxy-d-xylulose 5-phosphate synthase 1 (StDXS1) in response to Phytophthora infestans. Plant Sci. 243, 71–83. doi: 10.1016/j.plantsci.2015.12.001
Iorizzo, M., Ellison, S., Senalik, D., Zeng, P., Satapoomin, P., Huang, J., et al. (2016). A high-quality carrot genome assembly provides new insights into carotenoid accumulation and asterid genome evolution. Nat. Genet. 48, 657–666. doi: 10.1038/ng.3565
Lichtenthaler, H. K., and Buschmann, C. (2001). “Chlorophylls and carotenoids: measurement and characterization by UV-VIS spectroscopy,” in Current Protocols in Food Analytical Chemistry, ed. R. E. Wrolstad (New York, NY: John Wiley and Sons, Inc.), 1–8.
Lois, L. M., Rodriguez-Concepcion, M., Gallego, F., Campos, N., and Boronat, A. (2000). Carotenoid biosynthesis during tomato fruit development: regulatory role of 1-deoxy-D-xylulose 5-phosphate synthase. Plant J. 22, 503–513. doi: 10.1046/j.1365-313x.2000.00764.x
Lu, S., and Li, L. (2008). Carotenoid metabolism: biosynthesis, regulation, and beyond. J. Integr. Plant Biol. 50, 778–785. doi: 10.1111/j.1744-7909.2008.00708.x
Maass, D., Arango, J., Wust, F., Beyer, P., and Welsch, R. (2009). Carotenoid crystal formation in Arabidopsis and carrot roots caused by increased phytoene synthase protein Levels. PLoS ONE 4:e6373. doi: 10.1371/journal.pone.0006373
Mahmoud, S. S., and Croteau, R. B. (2001). Metabolic engineering of essential oil yield and composition in mint by altering expression of deoxyxylulose phosphate reductoisomerase and menthofuran synthase. Proc. Natl. Acad. Sci. U.S.A. 98, 8915–8920. doi: 10.1073/pnas.141237298
Mendoza-Poudereux, I., Munoz-Bertomeu, J., Arrillaga, I., and Segura, J. (2014). Deoxyxylulose 5-phosphate reductoisomerase is not a rate-determining enzyme for essential oil production in spike lavender. J. Plant Physiol. 171, 1564–1570. doi: 10.1016/j.jplph.2014.07.012
Moreno, J. C., Pizarro, L., Fuentes, P., Handford, M., Cifuentes, V., and Stange, C. (2013). Levels of lycopene beta-cyclase 1 modulate carotenoid gene expression and accumulation in Daucus carota. PLoS ONE 8:e58144. doi: 10.1371/journal.pone.0058144
Morris, W. L., Ducreux, L. J., Hedden, P., Millam, S., and Taylor, M. A. (2006). Overexpression of a bacterial 1-deoxy-D-xylulose 5-phosphate synthase gene in potato tubers perturbs the isoprenoid metabolic network: implications for the control of the tuber life cycle. J. Exp. Bot. 57, 3007–3018. doi: 10.1093/jxb/erl061
Munoz-Bertomeu, J., Arrillaga, I., Ros, R., and Segura, J. (2006). Up-regulation of 1-deoxy-D-xylulose-5-phosphate synthase enhances production of essential oils in transgenic spike lavender. Plant Physiol. 142, 890–900. doi: 10.1104/pp.106.086355
Murashige, T., and Skoog, F. (1962). A revised medium for rapid growth and bio assays with tobacco tissue cultures. Physiol. Plant. 15, 473–497. doi: 10.1111/j.1399-3054.1962.tb08052.x
Perello, C., Llamas, E., Burlat, V., Ortiz-Alcaide, M., Phillips, M. A., Pulido, P., et al. (2016). Differential subplastidial localization and turnover of enzymes involved in isoprenoid biosynthesis in chloroplasts. PLoS ONE 11:e0150539. doi: 10.1371/journal.pone.0150539
Pfaffl, M. W. (2001). A new mathematical model for relative quantification in real-time RT-PCR. Nucleic Acids Res. 29:e45. doi: 10.1093/nar/29.9.e45
Pokhilko, A., Bou-Torrent, J., Pulido, P., Rodriguez-Concepcion, M., and Ebenhoh, O. (2015). Mathematical modelling of the diurnal regulation of the MEP pathway in Arabidopsis. New Phytol 206, 1075–1085. doi: 10.1111/nph.13258
Pulido, P., Toledo-Ortiz, G., Phillips, M. A., Wright, L. P., and Rodriguez-Concepcion, M. (2013). Arabidopsis J-protein J20 delivers the first enzyme of the plastidial isoprenoid pathway to protein quality control. Plant Cell 25, 4183–4194. doi: 10.1105/tpc.113.113001
Rodriguez-Concepcion, M. (2010). Supply of precursors for carotenoid biosynthesis in plants. Arch. Biochem. Biophys. 504, 118–122. doi: 10.1016/j.abb.2010.06.016
Rodriguez-Concepcion, M., Ahumada, I., Diez-Juez, E., Sauret-Gueto, S., Lois, L. M., Gallego, F., et al. (2001). 1-Deoxy-D-xylulose 5-phosphate reductoisomerase and plastid isoprenoid biosynthesis during tomato fruit ripening. Plant J. 27, 213–222. doi: 10.1046/j.1365-313x.2001.01089.x
Rodriguez-Concepcion, M., and Boronat, A. (2002). Elucidation of the methylerythritol phosphate pathway for isoprenoid biosynthesis in bacteria and plastids. A metabolic milestone achieved through genomics. Plant Physiol. 130, 1079–1089. doi: 10.1104/pp.007138
Rodriguez-Concepcion, M., and Boronat, A. (2015). Breaking new ground in the regulation of the early steps of plant isoprenoid biosynthesis. Curr. Opin. Plant Biol. 25, 17–22. doi: 10.1016/j.pbi.2015.04.001
Rodriguez-Concepcion, M., and Stange, C. (2013). Biosynthesis of carotenoids in carrot: an underground story comes to light. Arch. Biochem. Biophys. 539, 110–116. doi: 10.1016/j.abb.2013.07.009
Rodriguez-Villalon, A., Gas, E., and Rodriguez-Concepcion, M. (2009a). Colors in the dark: a model for the regulation of carotenoid biosynthesis in etioplasts. Plant Signal. Behav. 4, 965–967. doi: 10.4161/psb.4.10.9672
Rodriguez-Villalon, A., Gas, E., and Rodriguez-Concepcion, M. (2009b). Phytoene synthase activity controls the biosynthesis of carotenoids and the supply of their metabolic precursors in dark-grown Arabidopsis seedlings. Plant J. 60, 424–435. doi: 10.1111/j.1365-313X.2009.03966.x
Ruiz-Sola, M. A., and Rodriguez-Concepcion, M. (2012). Carotenoid biosynthesis in Arabidopsis: a colorful pathway. Arabidopsis Book 10:e0158. doi: 10.1199/tab.0158
Stange, C., Fuentes, P., Handford, M., and Pizarro, L. (2008). Daucus carota as a novel model to evaluate the effect of light on carotenogenic gene expression. Biol. Res. 41, 289–301. doi: 10.4067/S0716-97602008000300006
Stange, C., and Rodriguez-Concepcion, M. (2015). “Carotenoids in carrot,” Pigments in Fruits and Vegetables: Genomics and Dietetics, ed. C. Chen (New York, NY: Springer), 277.
Surles, R. L., Weng, N., Simon, P. W., and Tanumihardjo, S. A. (2004). Carotenoid profiles and consumer sensory evaluation of specialty carrots (Daucus carota, L.) of various colors. J. Agric. Food Chem. 52, 3417–3421. doi: 10.1021/jf035472m
Walter, M. H., Hans, J., and Strack, D. (2002). Two distantly related genes encoding 1-deoxy-d-xylulose 5-phosphate synthases: differential regulation in shoots and apocarotenoid-accumulating mycorrhizal roots. Plant J. 31, 243–254. doi: 10.1046/j.1365-313X.2002.01352.x
Wright, L. P., Rohwer, J. M., Ghirardo, A., Hammerbacher, A., Ortiz-Alcaide, M., Raguschke, B., et al. (2014). Deoxyxylulose 5-Phosphate synthase controls flux through the methylerythritol 4-phosphate pathway in Arabidopsis. Plant Physiol. 165, 1488–1504. doi: 10.1104/pp.114.245191
Yang, J., Adhikari, M. N., Liu, H., Xu, H., He, G., Zhan, R., et al. (2012). Characterization and functional analysis of the genes encoding 1-deoxy-D-xylulose-5-phosphate reductoisomerase and 1-deoxy-D-xylulose-5-phosphate synthase, the two enzymes in the MEP pathway, from Amomum villosum lour. Mol. Biol. Rep. 39, 8287–8296. doi: 10.1007/s11033-012-1676-y
Zhang, H., Niu, D., Wang, J., Zhang, S., Yang, Y., Jia, H., et al. (2015). Engineering a platform for photosynthetic pigment, hormone and cembrane-related diterpenoid production in Nicotiana tabacum. Plant Cell Physiol. 56, 2125–2138. doi: 10.1093/pcp/pcv131
Keywords: carrot, storage root, deoxyxylulose 5-phosphate synthase (DXS), deoxyxylulose 5-phosphate reductoisomerase (DXR), carotenoids, chlorophylls
Citation: Simpson K, Quiroz LF, Rodriguez-Concepción M and Stange CR (2016) Differential Contribution of the First Two Enzymes of the MEP Pathway to the Supply of Metabolic Precursors for Carotenoid and Chlorophyll Biosynthesis in Carrot (Daucus carota). Front. Plant Sci. 7:1344. doi: 10.3389/fpls.2016.01344
Received: 14 June 2016; Accepted: 22 August 2016;
Published: 31 August 2016.
Edited by:
Soren K. Rasmussen, University of Copenhagen, DenmarkReviewed by:
Philipp W. Simon, United States Department of Agriculture and University of Wisconsin, USAMaria J. Rodrigo, Spanish National Research Council, Spain
Copyright © 2016 Simpson, Quiroz, Rodriguez-Concepción and Stange. This is an open-access article distributed under the terms of the Creative Commons Attribution License (CC BY). The use, distribution or reproduction in other forums is permitted, provided the original author(s) or licensor are credited and that the original publication in this journal is cited, in accordance with accepted academic practice. No use, distribution or reproduction is permitted which does not comply with these terms.
*Correspondence: Claudia R. Stange, cstange@uchile.cl