Dishevelled Paralogs in Vertebrate Development: Redundant or Distinct?
- 1Molecular Analysis—Mass Spectrometry, Center for Molecular and Cellular Bioengineering (CMCB), TU Dresden, Dresden, Germany
- 2Developmental Biology, Biology Department, Friedrich-Alexander University Erlangen-Nuremberg, Erlangen, Germany
Dishevelled (DVL) proteins are highly conserved in the animal kingdom and are important key players in β-Catenin-dependent and -independent Wnt signaling pathways. Vertebrate genomes typically comprise three DVL genes, DVL1, DVL2, and DVL3. Expression patterns and developmental functions of the three vertebrate DVL proteins however, are only partially redundant in any given species. Moreover, expression and function of DVL isoforms have diverged between different vertebrate species. All DVL proteins share basic functionality in Wnt signal transduction. Additional, paralog-specific interactions and functions combined with context-dependent availability of DVL isoforms may play a central role in defining Wnt signaling specificity and add selectivity toward distinct downstream pathways. In this review, we recapitulate briefly cellular functions of DVL paralogs, their role in vertebrate embryonic development and congenital disease.
Introduction
The Dishevelled (dsh1) phenotype has been described the first time in Drosophila close to 60 years ago (Fahmy and Fahmy, 1959) and the diverse molecular functions of Dishevelled (DVL) proteins still stimulate intensive research. To date DVL is considered the central intracellular effector of Wnt signaling pathways, which play key roles in establishing and patterning of the body axes and in the control of proliferation, differentiation, planar cell polarity (PCP), and cell movements throughout the animal kingdom. Although evidence is accumulating that Wnt pathways should be considered as a signaling network, individual pathways have been subdivided into the Wnt/β-Catenin pathway and the β-Catenin–independent pathways including Wnt/PCP, Wnt/Ca2+, and Wnt/STOP signaling, all of which involve DVL (reviewed in Kühl et al., 2000; Kohn and Moon, 2005; Macdonald et al., 2009; Niehrs and Acebron, 2012; van Amerongen, 2012; Collu and Mlodzik, 2015, Figure 1A).
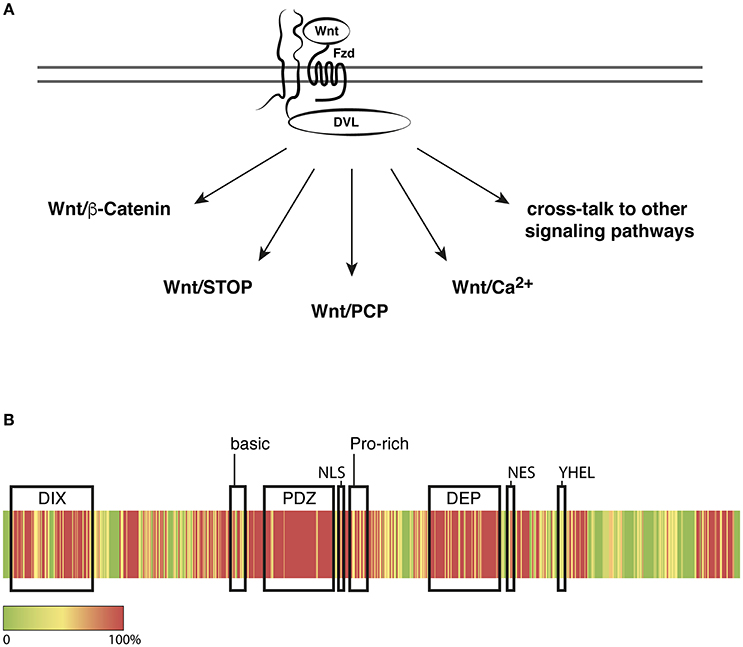
Figure 1. Dishevelled in Wnt/Frizzled signaling. (A) DVL relays Wnt/Frizzled signals to multiple signaling pathways to regulate cellular functions including mitosis, transcription, polarity, and migration. (B) Heatmap representation of the conservation of all three DVL proteins in human, rat, mouse, and frog. Sequence alignments were calculated using Clustal Omega with the following input sequences: Homo sapiens DVL1: O14640, DVL2: O14641, DVL3: Q92997, Rattus norvegicus DVL1: Q9WVB9, DVL2: D3ZB71, DVL3: D4ADV8, Mus musculus DVL1: P51141, DVL2: Q60838, DVL3: Q61062, Xenopus laevis DVL2: P51142, DVL3: Q6DKE2 (Uniprot Accession numbers), Xenopus laevis DVL1: NCBI XP_018081523. Red indicates 100% identity and Green indicates no identity of amino acids at the respective position. Conservation scores were calculated according to Livingstone and Barton (1993). Functional domains or motifs are indicated by correspondingly labeled boxes; for details and references see text.
DVL and its core functions in β-Catenin-dependent and -independent Wnt pathways are highly conserved. Notably, the genomes of Drosophila and most other invertebrates harbor only a single DVL gene. By contrast vertebrate genomes comprise genes for three DVL paralogs (DVL1-3), which have most likely arisen from two rounds of genome duplication (reviewed in Kasahara, 2007; Dillman et al., 2013). The still high degree of conservation among the three vertebrate DVL paralogs raises the question to which extent they have undergone functional diversification since obviously a single protein is sufficient to mediate DVL functions in invertebrates.
Cellular Functions of Dishevelled Paralogs
All DVL proteins share an N-terminal DIX domain, a PDZ and a DEP domain (Axelrod et al., 1998; Boutros et al., 1998; Li et al., 1999). Additional sequence motifs have been identified that provide interfaces for protein-protein interactions like the basic and proline-rich regions, or functional motifs as the nuclear export sequence (NES), a putative nuclear localization sequence (NLS), the—YHEL—motif that is required for internalization of the activated receptor complex and motifs that mediate the association of DVL with the cytoskeleton or with phospholipids (Capelluto et al., 2002; Penton et al., 2002; Itoh et al., 2005; Yu et al., 2007, Figure 1B). The lack of striking differences in the primary structure with no obvious additional or missing functional motifs and domains poses a challenge to predict functional differences and to develop assays that would reveal potential dissimilarities.
DVL interacts with the cytoplasmic interface of Frizzled receptors (Tauriello et al., 2012), regulates receptor internalization and maintenance of Frizzled membrane equilibrium through protein-protein interaction (Yu et al., 2007; Jiang et al., 2015) and serves as scaffold for numerous proteins including multiple protein kinases (reviewed in Gao and Chen, 2010; Mlodzik, 2016). Depending on the composition of the receptor complex and its interaction partners, DVL contributes to β-Catenin stabilization, inhibition of GSK3β or activation of β-Catenin-independent signaling cascades (Gao and Chen, 2010). DVL also enters the nucleus and interacts with TCF/c-Jun/β-Catenin or FOXK1/2 transcription factor complexes (Itoh et al., 2005; Gan et al., 2008; Wang et al., 2015). In addition, DVL proteins play a role in microtubule stabilization (Ciani et al., 2004), centrosome positioning and separation (Sepich et al., 2011; Cervenka et al., 2016) and mediate cross-talk with other signaling pathways such as Notch or NF-κB (Deng et al., 2010; Collu et al., 2012).
Notably, for most of the abovementioned DVL functions no preference for a DVL paralog has been detected, although some studies suggest dose-dependent effects (e.g., Cervenka et al., 2016). A different picture was observed for the role of DVL paralogs in PCP in ciliated cells, which is required to position the basal bodies. DVL1 was required for intact PCP signaling and localized asymmetrically in multiciliated cells in the epidermis of Xenopus tadpoles. DVL2 was concentrated in dots in vicinity to the basal bodies that led to a local concentration of active RhoA and was required for basal body positioning. Localization of DVL2 itself was mediated by Inturned and according to current knowledge neither of both proteins plays a role in ciliogenesis in the fly (Park et al., 2008). In the mouse node, DVL2 and DVL3 were apically localized and polarized to the posterior side. Positioning of the basal body and directional flow was disturbed or absent in DVL1/DVL2 or DVL1/DVL3 deficient embryos (Hashimoto et al., 2010). Moreover, polarized localization of DVL, planar polarity of basal bodies and their docking could be separated experimentally although the detailed mechanism remains elusive (Park et al., 2008; Vladar et al., 2009; Hashimoto et al., 2010).
DVL Paralogs in Vertebrate Embryonic Development
Embryonic Expression Patterns
In early Xenopus embryos, DVL2 and DVL3 are present maternally, whereas DVL1 expression is upregulated after the mid-blastula transition (Tadjuidje et al., 2011). At early gastrula stages, all three DVL paralogs are expressed in the prospective mesoderm including Spemann's organizer and, although weaker, in the ectoderm. Post-gastrula expression of DVL1 and DVL2 largely overlaps and is strongest in the neural tube, premigratory and migrating neural crest, as well as in the otic placode, the presomitic and somitic mesoderm. Notably, DVL3 was not expressed in the neuroectoderm but restricted to the paraxial mesoderm, the heart, cranial placodes, and at tadpole stages to the branchial arches (Gray et al., 2009).
In chicken embryos, only two DVL genes, DVL1 and DVL3, were identified. DVL3 was already expressed in day 2 embryos and showed broad expression in most embryonic tissues whereas DVL1 was upregulated only after day 2, i.e., after completion of neurogenesis, and showed a spatially restricted expression in the brain, strongest in the optic stalk, the olfactory bulb, and the ventral forebrain and spinal chord (Gray et al., 2009).
All murine DVL genes are maternally expressed in mouse oocytes and pre-implantation embryos, but interestingly individual protein levels differ considerably and dynamically from oocyte to blastocyst (Na et al., 2007). Post-implantation, DVL1 expression was detected in the mesoderm, but not in the node, at stage E7.5. Post-gastrula, DVL1 was expressed strongest in the neuroectoderm and later in the CNS, cranial and dorsal root ganglia, somites, the liver, kidney, intestine, and lung (Sussman et al., 1994). For DVL2, ubiquitous expression has been reported during embryogenesis in the mouse (Klingensmith et al., 1996). At E 7.5, DVL3 has also been detected ubiquitously, but shortly after showed elevated expression in the CNS and the somitic mesoderm, the notochord, heart, dorsal root ganglia, and branchial arches and in the limb buds (Tsang et al., 1996; Diez-Roux et al., 2011).
Phylogenetic analyses suggest that DVL1 separated first from the common ancestor of DVL2/3, which split into DVL2 and DVL3 in a second round of duplication (Gray et al., 2009). Consistent with corresponding functional divergence, expression of at least one DVL2/3 paralog in the oocyte and pre-blastula embryo and of DLV1 in the central nervous system appear to be conserved among vertebrates. Except for these conserved patterns, developmental expression of the three DVL genes is highly divergent within a species and among different species with DVL2 expression showing the highest variability.
Developmental Function and Human Congenital Disease
Both, animal models and human congenital disease provide insights into the developmental function of vertebrate DVL paralogs. To date, DVL has predominantly been studied in the mouse and, to a much lesser extent, in Xenopus. Transgenic, single, and compound knock-out mouse models have been discussed comprehensively and in detail in a review by Wynshaw-Boris (2012, see also Supplementary Table 1), therefore we will focus here on human congenital disease and related phenotypes in animal models. In humans, mutations in DVL genes have been associated with neural tube closure defects (NTD) and autosomal-dominant Robinow Syndrome (ADRS) (De Marco et al., 2013; Bunn et al., 2015; White et al., 2015, 2016).
Neural Tube Defects
During embryonic development, the neural tube is formed by elevation, convergence and fusion of the lateral neural folds to form a hollow tube. Morphogenesis and closure of the neural tube is affected by nutritional, environmental and genetic factors including Wnt/PCP signaling, which is illustrated by genetic association between NTD in humans and mutations in the PCP genes VANGL1, VANGL2, CELSR1, FZD6, and DVL2 (Cai and Shi, 2014; reviewed in De Marco et al., 2014). Notably, also mutations in DVL1 or DVL3 have been identified in humans with NTD (Figure 2), although the correlation was not significant (De Marco et al., 2013; Merello et al., 2013; Chen et al., 2016).
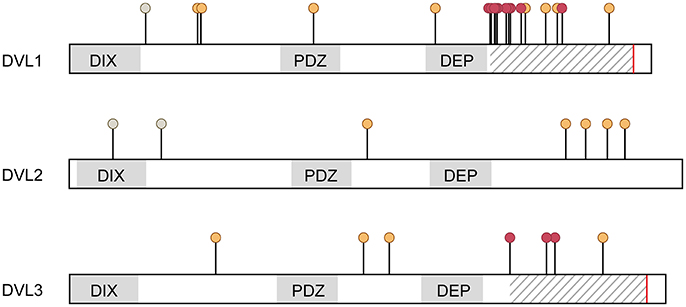
Figure 2. Mutations identified in the three DVL genes in humans. Mutations are indicated at the positions of amino acid changes. Changes detected in individuals with NTDs (De Marco et al., 2013; Merello et al., 2013; Chen et al., 2016) are color coded orange (predicted pathogenic) and gray (predicted benign, in all cases A>V). All ADRS mutations are (−1)-frameshift mutations resulting in altered amino acid sequences in the C-terminus and a premature stop (Bunn et al., 2015; White et al., 2015, 2016), which are indicated by hatched area and red line respectively. Positions of individual mutations associated with ADRS are labelled with red dots.
Consistently, neural tube closure also requires the same PCP factors in mouse, frog, and zebrafish (Darken et al., 2002; Hamblet et al., 2002; Jessen et al., 2002; Curtin et al., 2003; Formstone and Mason, 2005; Wang et al., 2006). DVL2−/− mice show NTD while single and compound DVL1 and DVL3 deficient mice do not, suggesting that among the three DVL genes, DVL2 is necessary and sufficient to mediate neural tube closure. However, DVL2−/− DVL3+/− and DVL1−/− DVL2−/− mice display much more severe NTD than DVL2−/− mice (Hamblet et al., 2002; Wang et al., 2006; Etheridge et al., 2008), which strongly suggests that DVL1 and DVL3 contribute directly or indirectly to neural tube closure. Along this line, maternal single knock-down of either DVL2 or DVL3 in Xenopus caused NTD (Tadjuidje et al., 2011), supporting a contribution of DVL2 and DVL3.
Autosomal-Dominant Robinow Syndrome
Robinow syndrome is a rare genetic disorder characterized by mesomelic limb shortening, short stature, cranio-facial malformations, microgenitalia, and occasional cardiac outflow tract defects with either autosomal dominant or recessive inheritance (reviewed in Robinow et al., 1969; Patton and Afzal, 2002). Notably and despite the multifaceted clinical presentation of affected individuals, NTD have neither been described in ADRS nor the more severe recessive Robinow syndrome (RRS). Two recent studies have identified mutations in exon 14 of DVL1 and DVL3 as causative for ADRS (Bunn et al., 2015; White et al., 2015, 2016, Figure 2). In addition, ADRS is also associated with WNT5A whereas RRS is caused by loss-of-function mutations in ROR2 (Afzal et al., 2000; van Bokhoven et al., 2000; Person et al., 2010). Thus, the features of Robinow syndrome are generally considered as consequences of defective WNT5A/ROR2-mediated PCP signaling in multiple tissues (Wang et al., 2011) and are partially recapitulated in DVL deficient animal models.
Short stature and defects of the axial skeleton are likely related to impaired convergent extension movements of the paraxial mesoderm and defects in somitogenesis, which have also been reported for DVL2−/−, DVL1−/−;DVL2−/−, and DVL2+/−;DVL3−/− mice as well as for Xenopus embryos deficient of any DVL paralog (Hamblet et al., 2002; Etheridge et al., 2008; Gray et al., 2009; Gentzel et al., 2015), indicating that all DVL paralogs contribute to the development of the axial skeleton although DVL2 seems of particular importance.
The characteristic cranio-facial deformations seen in ADRS or RRS indicate defective development of the neural crest (NC), which gives rise to the majority of cranial cartilage and bone. In addition, a subpopulation of the NC contributes to the cardiac outflow tract (OFT). Cranio-facial malformations are also visible in DVL1 and DVL2 morphant Xenopus embryos and the abovementioned mice. The latter and additionally DVL3−/− animals also show cardiac OFT defects. In Xenopus, DVL1 or DVL2 morphant embryos showed normal NC induction but defects in NC migration. The NC is present in DVL3−/− mice whereas the cardiac NC markers PITX2 and PLEXINA2 were decreased in mice lacking DVL2 (Hamblet et al., 2002; Etheridge et al., 2008; Gray et al., 2009), indicating differential roles of DVL2 and DVL3 in NC and cardiac development.
Interestingly, DVL1 mutations in humans affect predominantly cranio-facial development with little or no aberrations in body height, whereas in DVL3 and WNT5A associated ADRS craniofacial malformations are accompanied by short stature (Person et al., 2010; Bunn et al., 2015; White et al., 2015, 2016). In mouse, DVL1 is predominantly expressed in the neuroectoderm (Sussman et al., 1994) and as discussed above, knock-out models suggest dominant roles of DVL2 and DVL3 in the development of the axial skeleton and the heart. Although the spatial expression of the three DVL isoforms in human embryos is unknown, the differential prevalence of defects in the axial skeleton in DVL1 and DVL3 associated ADRS supports a prevailing role of DVL2/3 in the paraxial mesoderm in mammals.
DVL Signaling in Embryonic Development
The recently characterized mutations in DVL1 and DVL3 are frameshift mutations, which alter and shorten the C-termini in the corresponding proteins (White et al., 2015, 2016). This C-terminal domain has been shown to interact with ROR2, a major receptor in β-Catenin independent Wnt signaling and affected in RRS, and is required for ROR2-mediated inhibition of Wnt/β-Catenin signaling (Witte et al., 2010). An initial study suggests a gain of Wnt/β-Catenin activity in ADRS (Bunn et al., 2015), thus it is conceivable that ADRS mutations in DVL1 and DVL3 might result in defective β-Catenin independent Wnt signaling and concomitantly upregulate Wnt/β-Catenin activity.
Malformations of the axial skeleton seen in DVL2−/− and DVL1−/−;DVL2−/− mice are reminiscent of the phenotypes in ROR2 or WNT5A deficient embryos that can be attributed to aberrant PCP signaling in the paraxial mesoderm (reviewed in Stricker et al., 2017). Genetic interactions between DVL2 and DVL3 with VANGL2 in the mouse further suggest that DVL2 acts in Wnt/PCP signaling in neural tube closure and in cochlea development (Wang et al., 2006; Etheridge et al., 2008). In addition, NTDs and OFT defects in DVL1−/−;DVL2−/− mice were similar to defects in VANGL2 mutants and rescued by a DVL2ΔDIX transgene, which is defective in β-Catenin signaling but retains activity in PCP signaling (Wang et al., 2006; Sinha et al., 2012). Notably, also DVL3 KO mice develop OFT defects, but no genetic interaction with VANGL2 in OFT morphogenesis could be demonstrated, indicating a non-redundant function of DVL3 (Etheridge et al., 2008).
Wnt/β-Catenin signaling also contributes to the development of the paraxial mesoderm, heart and neural crest, and patterns the neural tube. However, defective Wnt/β-Catenin signaling results in patterning defects of the dorsal mesoderm and affects proliferation, expansion, or specification of dorsal neural tube progenitors and neural crest (NC) cells (Greco et al., 1996; Ikeya et al., 1997; Pinson et al., 2000; Lou et al., 2008; Seldin et al., 2008; Valenta et al., 2011). By contrast, in either single or compound DVL knock-out mice, Wnt/β-Catenin signaling, and early dorsal mesoderm markers were unaffected. Only in triple knock-out mice a marked downregulation of Wnt/β-catenin signaling has been observed (Etheridge et al., 2008; Hashimoto et al., 2010). Still, defective β-Catenin signaling in smaller cell populations cannot be excluded. One such example might be the cardiac neural crest in DVL2−/− embryos, in which the β-Catenin target PITX2 is downregulated (Hamblet et al., 2002; Kioussi et al., 2002). PITX2 is required for proliferation of cardiac NC, but also for the interaction between cardiac NC and second heart field cells (Kioussi et al., 2002; Ma et al., 2013) and, indirectly, for OFT morphogenesis via its target gene WNT11 (Zhou et al., 2007). OFT defects in DLV1−/−, DVL2−/− embryos were rescued by a DVL2ΔDIX transgene (Sinha et al., 2012), however this does not exclude a role of DVL2 in β-Catenin signaling upstream of PITX2 since the transgene could also restore OFT morphogenesis downstream of WNT11.
Overall it appears that DVL function in β-Catenin-independent Wnt signaling is more sensitive to the loss or dysfunction of one or two paralogs and accounts for most of the developmental phenotypes in knock-out animal models and also for the features of ADRS.
Induced Heart Defects
A number of studies indicate a specific role of DVL1 in cardiac remodeling and regeneration. DVL1 and CamKII are upregulated after induced myocardial infarction and heart failure indicating a role of Wnt/Ca2+ signaling in regeneration (Chen et al., 2004; Ai et al., 2005; Bossuyt et al., 2008). Persistent pressure overload induced cardiac hypertrophy was attenuated in DVL1 knock-out mice, which has been attributed to lower Wnt/β-Catenin activity as well as decreased AKT activation (van de Schans et al., 2007). Consistently, DVL1 gain-of-function induced progressive cardiomyopathy (Malekar et al., 2010). Interestingly, evidence is accumulating that DVL1 is functionally associated with Wnt/Ca2+ and CamKII signaling in cardiomyopathy (Malekar et al., 2010; Zhang et al., 2015), in excitory synapses in the rat spinal chord (Ciani et al., 2011) and in convergent extension movements in Xenopus gastrulation (Gentzel et al., 2015), indicating a functional specification of DVL1.
Perspectives
Striking differences between different DVLs and species have been observed in temporal and spatial expression patterns. Loss-of-function phenotypes of each single paralog in mouse as well as ADRS features associated with DVL1 or DVL3 mutations also differ, indicating some degree of divergence but also overlapping functions. In addition, expression of transgenes in a single knock-out background further supported partial redundancy and indicated a dose dependency. If the DVL paralogs would be fully redundant in function it might be speculated that the summed abundance of all paralogs is important for cell survival. But even in cell culture models, any single knock-down is effective and the cells apparently do not sense overall “DVL concentration” and do not compensate the down-regulation of one protein by upregulation of the others (Cervenka et al., 2016).
Functional redundancies however, do not connote biochemical identity. The observed differences could reflect differential expression levels, epistatic relations, or differential biochemical properties such as protein-protein interaction affinity and consequently also molecular function, which would also be consistent with dose-dependencies. The developmental phenotypes further indicate that β-Catenin independent Wnt pathways are more sensitive to the dose of individual DVL paralogs than β-Catenin signaling. This hypothesis is further supported by comparison of triple knock-out and triple-RNAi knock-down embryos. Whereas, in triple KO embryos early β-Catenin signaling is strongly reduced resulting in axis and mesodermal mispatterning, in the knock-down, in which ~25–30% of each paralog were retained, early β-Catenin signaling was not affected, but the embryos showed strong morphogenetic defects in the dorsal mesoderm and neuroectoderm. Consistently, specific and different molecular functionality of the three DVL paralogs has been observed in ciliogenesis and the Wnt/Ca2+ pathway, which were revealed in intact tissue or tissue models (Park et al., 2008; Gentzel et al., 2015).
Overall, the currently available data indicate that DVL expression and function have diverged to some degree apparently and consistent with phylogenetic models mostly between DVL1 and DVL2/3. Thus, depending on the cellular context, DVL paralogs exhibit both redundant and distinct functionality.
Author Contributions
All authors listed, have made substantial, direct and intellectual contribution to the work, and approved it for publication.
Conflict of Interest Statement
The authors declare that the research was conducted in the absence of any commercial or financial relationships that could be construed as a potential conflict of interest.
Acknowledgments
The authors' original work was funded by German Research foundation (DFG) grants to AS (grant numbers SCHA965/6-1 and 6-2), MG was funded by the Max Planck Society (MPG).
Supplementary Material
The Supplementary Material for this article can be found online at: https://www.frontiersin.org/article/10.3389/fcell.2017.00059/full#supplementary-material
References
Afzal, A. R., Rajab, A., Fenske, C. D., Oldridge, M., Elanko, N., Ternes-Pereira, E., et al. (2000). Recessive Robinow syndrome, allelic to dominant brachydactyly type B, is caused by mutation of ROR2. Nat. Genet. 25, 419–422. doi: 10.1038/78107
Ai, X., Curran, J. W., Shannon, T. R., Bers, D. M., and Pogwizd, S. M. (2005). Ca2+/calmodulin-dependent protein kinase modulates cardiac ryanodine receptor phosphorylation and sarcoplasmic reticulum Ca2+ leak in heart failure. Circ. Res. 97, 1314–1322. doi: 10.1161/01.RES.0000194329.41863.89
Axelrod, J. D., Miller, J. R., Shulman, J. M., Moon, R. T., and Perrimon, N. (1998). Differential recruitment of Dishevelled provides signaling specificity in the planar cell polarity and Wingless signaling pathways. Genes Dev. 12, 2610–2622.
Bossuyt, J., Helmstadter, K., Wu, X., Clements-Jewery, H., Haworth, R. S., Avkiran, M., et al. (2008). Ca2+/calmodulin-dependent protein kinase IIdelta and protein kinase D overexpression reinforce the histone deacetylase 5 redistribution in heart failure. Circ. Res. 102, 695–702. doi: 10.1161/CIRCRESAHA.107.169755
Boutros, M., Paricio, N., Strutt, D. I., and Mlodzik, M. (1998). Dishevelled activates JNK and discriminates between JNK pathways in planar polarity and wingless signaling. Cell 94, 109–118.
Bunn, K. J., Daniel, P., Rösken, H. S., O'Neill, A. C., Cameron-Christie, S. R., Morgan, T., et al. (2015). Mutations in DVL1 cause an osteosclerotic form of Robinow syndrome. Am. J. Hum. Genet. 96, 623–630. doi: 10.1016/j.ajhg.2015.02.010
Cai, C., and Shi, O. (2014). Genetic evidence in planar cell polarity signaling pathway in human neural tube defects. Front. Med. 8, 68–78. doi: 10.1007/s11684-014-0308-4
Capelluto, D. G. S., Kutateladze, T. G., Habas, R., Finkielstein, C. V., He, X., and Overduin, M. (2002). The DIX domain targets dishevelled to actin stress fibres and vesicular membranes. Nature 419, 726–729. doi: 10.1038/nature01056
Cervenka, I., Valnohova, J., Bernatik, O., Harnoš, J., Radsetoulal, M., Sedová, K., et al. (2016). Dishevelled is a NEK2 kinase substrate controlling dynamics of centrosomal linker proteins. Proc. Natl. Acad. Sci. U.S.A. 113, 9304–9309. doi: 10.1073/pnas.1608783113
Chen, L., Wu, Q., Guo, F., Xia, B., and Zuo, J. (2004). Expression of Dishevelled-1 in wound healing after acute myocardial infarction: possible involvement in myofibroblast proliferation and migration. J. Cell. Mol. Med. 8, 257–264. doi: 10.1111/j.1582-4934.2004.tb00281.x
Chen, S., Zhang, Q., Bai, B., Ouyang, S., Bao, Y., Li, H., et al. (2016). MARK2/Par1b insufficiency attenuates DVL gene transcription via histone deacetylation in lumbosacral spina bifida. Mol. Neurobiol. doi: 10.1007/s12035-016-0164-0. [Epub ahead of print].
Ciani, L., Boyle, K. A., Dickins, E., Sahores, M., Anane, D., Lopes, D. M., et al. (2011). Wnt7a signaling promotes dendritic spine growth and synaptic strength through Ca2+/Calmodulin-dependent protein kinase II. Proc. Natl. Acad. Sci. U.S.A. 108, 10732–10737. doi: 10.1073/pnas.1018132108
Ciani, L., Krylova, O., Smalley, M. J., Dale, T. C., and Salinas, P. C. (2004). A divergent canonical WNT-signaling pathway regulates microtubule dynamics: dishevelled signals locally to stabilize microtubules. J. Cell Biol. 164, 243–253. doi: 10.1083/jcb.200309096
Collu, G. M., and Mlodzik, M. (2015). Planar polarity: converting a morphogen gradient into cellular polarity. Curr. Biol. 25, R372–R374. doi: 10.1016/j.cub.2015.03.011
Collu, G. M., Hidalgo-Sastre, A., Acar, A., Bayston, L., Gildea, C., Leverentz, M. K., et al. (2012). Dishevelled limits Notch signalling through inhibition of CSL. Development 139, 4405–4415. doi: 10.1242/dev.081885
Curtin, J. A., Quint, E., Tsipouri, V., Arkell, R. M., Cattanach, B., Copp, A. J., et al. (2003). Mutation of Celsr1 disrupts planar polarity of inner ear hair cells and causes severe neural tube defects in the mouse. Curr. Biol. 13, 1129–1133. doi: 10.1016/S0960-9822(03)00374-9
Darken, R. S., Scola, A. M., Rakeman, A. S., Das, G., Mlodzik, M., and Wilson, P. A. (2002). The planar polarity gene strabismus regulates convergent extension movements in Xenopus. EMBO J. 21, 976–985. doi: 10.1093/emboj/21.5.976
De Marco, P., Merello, E., Consales, A., Piatelli, G., Cama, A., Kibar, Z., et al. (2013). Genetic analysis of disheveled 2 and disheveled 3 in human neural tube defects. J. Mol. Neurosci. 49, 582–588. doi: 10.1007/s12031-012-9871-9
De Marco, P., Merello, E., Piatelli, G., Cama, A., Kibar, Z., and Capra, V. (2014). Planar cell polarity gene mutations contribute to the etiology of human neural tube defects in our population. Birth Defects Res. A Clin. Mol. Teratol. 100, 633–641. doi: 10.1002/bdra.23255
Deng, N., Ye, Y., Wang, W., and Li, L. (2010). Dishevelled interacts with p65 and acts as a repressor of NF-κB-mediated transcription. Cell Res. 20, 1117–1127. doi: 10.1038/cr.2010.108
Diez-Roux, G., Banfi, S., Sultan, M., Geffers, L., Anand, S., Rozado, D., et al. (2011). A high-resolution anatomical atlas of the transcriptome in the mouse embryo. PLoS Biol. 9:e1000582. doi: 10.1371/journal.pbio.1000582.s018
Dillman, A. R., Minor, P. J., and Sternberg, P. W. (2013). Origin and evolution of dishevelled. G3 (Bethesda). 3, 251–262. doi: 10.1534/g3.112.005314
Etheridge, S. L., Ray, S., Li, S., Hamblet, N. S., Lijam, N., Tsang, M., et al. (2008). Murine dishevelled 3 functions in redundant pathways with dishevelled 1 and 2 in normal cardiac outflow tract, cochlea, and neural tube development. PLoS Genet. 4:e1000259. doi: 10.1371/journal.pgen.1000259
Formstone, C. J., and Mason, I. (2005). Combinatorial activity of Flamingo proteins directs convergence and extension within the early zebrafish embryo via the planar cell polarity pathway. Dev. Biol. 282, 320–335. doi: 10.1016/j.ydbio.2005.03.026
Gan, X.-Q., Wang, J.-Y., Xi, Y., Wu, Z.-L., Li, Y.-P., and Li, L. (2008). Nuclear Dvl, c-Jun, β-catenin, and TCF form a complex leading to stabilization of β-catenin-TCF interaction. J. Cell Biol. 180, 1087–1100. doi: 10.1083/jcb.200710050
Gao, C., and Chen, Y.-G. (2010). Dishevelled: the hub of Wnt signaling. Cell. Signal. 22, 717–727. doi: 10.1016/j.cellsig.2009.11.021
Gentzel, M., Schille, C., Rauschenberger, V., and Schambony, A. (2015). Distinct functionality of dishevelled isoforms on Ca2+/calmodulin-dependent protein kinase 2 (CamKII) in Xenopus gastrulation. Mol. Biol. Cell 26, 966–977. doi: 10.1091/mbc.E14-06-1089
Gray, R. S., Bayly, R. D., Green, S. A., Agarwala, S., Lowe, C. J., and Wallingford, J. B. (2009). Diversification of the expression patterns and developmental functions of the dishevelled gene family during chordate evolution. Dev. Dyn. 238, 2044–2057. doi: 10.1002/dvdy.22028
Greco, T. L., Takada, S., Newhouse, M. M., McMahon, J. A., McMahon, A. P., and Camper, S. A. (1996). Analysis of the vestigial tail mutation demonstrates that Wnt-3a gene dosage regulates mouse axial development. Genes Dev. 10, 313–324.
Hamblet, N. S., Lijam, N., Ruiz-Lozano, P., Wang, J., Yang, Y., Luo, Z., et al. (2002). Dishevelled 2 is essential for cardiac outflow tract development, somite segmentation and neural tube closure. Development 129, 5827–5838. doi: 10.1242/dev.00164
Hashimoto, M., Shinohara, K., Wang, J., Ikeuchi, S., Yoshiba, S., Meno, C., et al. (2010). Planar polarization of node cells determines the rotational axis of node cilia. Nat. Cell Biol. 12, 170–176. doi: 10.1038/ncb2020
Ikeya, M., Lee, S. M., Johnson, J. E., McMahon, A. P., and Takada, S. (1997). Wnt signalling required for expansion of neural crest and CNS progenitors. Nature 389, 966–970. doi: 10.1038/40146
Itoh, K., Brott, B. K., Bae, G.-U., Ratcliffe, M. J., and Sokol, S. Y. (2005). Nuclear localization is required for Dishevelled function in Wnt/β-catenin signaling. J. Biol. 4, 3. doi: 10.1186/jbiol20
Jessen, J. R., Topczewski, J., Bingham, S., Sepich, D. S., Marlow, F., Chandrasekhar, A., et al. (2002). Zebrafish trilobite identifies new roles for Strabismus in gastrulation and neuronal movements. Nat. Cell Biol. 4, 610–615. doi: 10.1038/ncb828
Jiang, X., Charlat, O., Zamponi, R., Yang, Y., and Cong, F. (2015). Dishevelled promotes Wnt receptor degradation through recruitment of ZNRF3/RNF43 E3 ubiquitin ligases. Mol. Cell 58, 522–533. doi: 10.1016/j.molcel.2015.03.015
Kasahara, M. (2007). The 2R hypothesis: an update. Curr. Opin. Immunol. 19, 547–552. doi: 10.1016/j.coi.2007.07.009
Kioussi, C., Briata, P., Baek, S. H., Rose, D. W., Hamblet, N. S., Herman, T., et al. (2002). Identification of a Wnt/Dvl/β-Catenin –> Pitx2 pathway mediating cell-type-specific proliferation during development. Cell 111, 673–685. doi: 10.1016/S0092-8674(02)01084-X
Klingensmith, J., Yang, Y., Axelrod, J. D., Beier, D. R., Perrimon, N., and Sussman, D. J. (1996). Conservation of dishevelled structure and function between flies and mice: isolation and characterization of Dvl2. Mech. Dev. 58, 15–26.
Kohn, A. D., and Moon, R. T. (2005). Wnt and calcium signaling: β-catenin-independent pathways. Cell Calcium 38, 439–446. doi: 10.1016/j.ceca.2005.06.022
Kühl, M., Sheldahl, L. C., Park, M., Miller, J. R., and Moon, R. T. (2000). The Wnt/Ca2+ pathway: a new vertebrate Wnt signaling pathway takes shape. Trends Genet. 16, 279–283. doi: 10.1074/jbc.275.17.12701
Li, L., Yuan, H., Xie, W., Mao, J., Caruso, A. M., McMahon, A., et al. (1999). Dishevelled proteins lead to two signaling pathways. Regulation of LEF-1 and c-Jun N-terminal kinase in mammalian cells. J. Biol. Chem. 274, 129–134.
Livingstone, C. D., and Barton, G. J. (1993). Protein sequence alignments: a strategy for the hierarchical analysis of residue conservation. Comput. Appl. Biosci. 9, 745–756.
Lou, D. Y., Dominguez, I., Toselli, P., Landesman-Bollag, E., O'Brien, C., and Seldin, D. C. (2008). The alpha catalytic subunit of protein kinase CK2 is required for mouse embryonic development. Mol. Cell. Biol. 28, 131–139. doi: 10.1128/MCB.01119-07
Ma, H.-Y., Xu, J., Eng, D., Gross, M. K., and Kioussi, C. (2013). Pitx2-mediated cardiac outflow tract remodeling. Dev. Dyn. 242, 456–468. doi: 10.1002/dvdy.23934
Macdonald, B. T., Tamai, K., and He, X. (2009). Wnt/β-catenin signaling: components, mechanisms, and diseases. Dev. Cell 17, 9–26. doi: 10.1016/j.devcel.2009.06.016
Malekar, P., Hagenmueller, M., Anyanwu, A., Buss, S., Streit, M. R., Weiss, C. S., et al. (2010). Wnt signaling is critical for maladaptive cardiac hypertrophy and accelerates myocardial remodeling. Hypertension 55, 939–945. doi: 10.1161/HYPERTENSIONAHA.109.141127
Merello, E., Kibar, Z., Allache, R., Piatelli, G., Cama, A., Capra, V., et al. (2013). Rare missense variants in DVL1, one of the human counterparts of the Drosophila dishevelled gene, do not confer increased risk for neural tube defects. Birth Defects Res. Clin. Mol. Teratol. 97, 452–455. doi: 10.1002/bdra.23157
Mlodzik, M. (2016). The dishevelled protein family: still rather a mystery after over 20 years of molecular studies. Curr. Top. Dev. Biol. 117, 75–91. doi: 10.1016/bs.ctdb.2015.11.027
Na, J., Lykke-Andersen, K., Torres Padilla, M. E., and Zernicka-Goetz, M. (2007). Dishevelled proteins regulate cell adhesion in mouse blastocyst and serve to monitor changes in Wnt signaling. Dev. Biol. 302, 40–49. doi: 10.1016/j.ydbio.2006.08.036
Niehrs, C., and Acebron, S. P. (2012). Mitotic and mitogenic Wnt signalling. EMBO J. 31, 2705–2713. doi: 10.1038/emboj.2012.124
Park, T. J., Mitchell, B. J., Abitua, P. B., Kintner, C., and Wallingford, J. B. (2008). Dishevelled controls apical docking and planar polarization of basal bodies in ciliated epithelial cells. Nat. Genet. 40, 871–879. doi: 10.1038/ng.104
Patton, M. A., and Afzal, A. R. (2002). Robinow syndrome. J. Med. Genet. 39, 305–310. doi: 10.1136/jmg.39.5.305
Penton, A., Wodarz, A., and Nusse, R. (2002). A mutational analysis of dishevelled in Drosophila defines novel domains in the dishevelled protein as well as novel suppressing alleles of axin. Genetics 161, 747–762.
Person, A. D., Beiraghi, S., Sieben, C. M., Hermanson, S., Neumann, A. N., Robu, M. E., et al. (2010). WNT5A mutations in patients with autosomal dominant Robinow syndrome. Dev. Dyn. 239, 327–337. doi: 10.1002/dvdy.22156
Pinson, K. I., Brennan, J., Monkley, S., Avery, B. J., and Skarnes, W. C. (2000). An LDL-receptor-related protein mediates Wnt signalling in mice. Nature 407, 535–538. doi: 10.1038/35035124
Robinow, M., Silverman, F. N., and Smith, H. D. (1969). A newly recognized dwarfing syndrome. Am. J. Dis. Child. 117, 645–651.
Seldin, D. C., Lou, D. Y., Toselli, P., Landesman-Bollag, E., and Dominguez, I. (2008). Gene targeting of CK2 catalytic subunits. Mol. Cell. Biochem. 316, 141–147. doi: 10.1007/s11010-008-9811-8
Sepich, D. S., Usmani, M., Pawlicki, S., and Solnica-Krezel, L. (2011). Wnt/PCP signaling controls intracellular position of MTOCs during gastrulation convergence and extension movements. Development 138, 543–552. doi: 10.1242/dev.053959
Sinha, T., Wang, B., Evans, S., Wynshaw-Boris, A., and Wang, J. (2012). Disheveled mediated planar cell polarity signaling is required in the second heart field lineage for outflow tract morphogenesis. Dev. Biol. 370, 135–144. doi: 10.1016/j.ydbio.2012.07.023
Stricker, S., Rauschenberger, V., and Schambony, A. (2017). ROR-family receptor tyrosine kinases. Curr. Top. Dev. Biol. 123, 105–142. doi: 10.1016/bs.ctdb.2016.09.003
Sussman, D. J., Klingensmith, J., Salinas, P., Adams, P. S., Nusse, R., and Perrimon, N. (1994). Isolation and characterization of a mouse homolog of the Drosophila segment polarity gene dishevelled. Dev. Biol. 166, 73–86. doi: 10.1006/dbio.1994.1297
Tadjuidje, E., Cha, S.-W., Louza, M., Wylie, C., and Heasman, J. (2011). The functions of maternal Dishevelled 2 and 3 in the Early Xenopus embryo. Dev. Dyn. 240, 1727–1736. doi: 10.1002/dvdy.22671
Tauriello, D. V. F., Jordens, I., Kirchner, K., Slootstra, J. W., Kruitwagen, T., Bouwman, B. A. M., et al. (2012). Wnt/β-catenin signaling requires interaction of the Dishevelled DEP domain and C terminus with a discontinuous motif in Frizzled. Proc. Natl. Acad. Sci. U.S.A. 109, E812–E820. doi: 10.1073/pnas.1114802109
Tsang, M., Lijam, N., Yang, Y., Beier, D. R., Wynshaw-Boris, A., and Sussman, D. J. (1996). Isolation and characterization of mouse dishevelled-3. Dev. Dyn. 207, 253–262. doi: 10.1002/(SICI)1097-0177(199611)207:3<253::AID-AJA2>3.0.CO;2-G
Valenta, T., Gay, M., Steiner, S., Draganova, K., Zemke, M., Hoffmans, R., et al. (2011). Probing transcription-specific outputs of β-catenin in vivo. Genes Dev. 25, 2631–2643. doi: 10.1101/gad.181289.111
van Amerongen, R. (2012). Alternative Wnt Pathways and Receptors. Cold Spring Harb. Perspect. Biol. 4:a007914. doi: 10.1101/cshperspect.a007914
van Bokhoven, H., Celli, J., Kayserili, H., van Beusekom, E., Balci, S., Brussel, W., et al. (2000). Mutation of the gene encoding the ROR2 tyrosine kinase causes autosomal recessive Robinow syndrome. Nat. Genet. 25, 423–426. doi: 10.1038/78113
van de Schans, V. A. M., van den Borne, S. W. M., Strzelecka, A. E., Janssen, B. J. A., van der Velden, J. L. J., Langen, R. C. J., et al. (2007). Interruption of Wnt signaling attenuates the onset of pressure overload-induced cardiac hypertrophy. Hypertension 49, 473–480. doi: 10.1161/01.HYP.0000255946.55091.24
Vladar, E. K., Antic, D., and Axelrod, J. D. (2009). Planar cell polarity signaling: the developing cell's compass. Cold Spring Harb. Perspect. Biol. 1:a002964. doi: 10.1101/cshperspect.a002964
Wang, B., Sinha, T., Jiao, K., Serra, R., and Wang, J. (2011). Disruption of PCP signaling causes limb morphogenesis and skeletal defects and may underlie Robinow syndrome and brachydactyly type B. Hum. Mol. Genet. 20, 271–285. doi: 10.1093/hmg/ddq462
Wang, J., Hamblet, N. S., Mark, S., Dickinson, M. E., Brinkman, B. C., Segil, N., et al. (2006). Dishevelled genes mediate a conserved mammalian PCP pathway to regulate convergent extension during neurulation. Development 133, 1767–1778. doi: 10.1242/dev.02347
Wang, W., Li, X., Lee, M., Jun, S., Aziz, K. E., Feng, L., et al. (2015). FOXKs promote Wnt/β-catenin signaling by translocating DVL into the nucleus. Dev. Cell 32, 707–718. doi: 10.1016/j.devcel.2015.01.031
White, J. J., Mazzeu, J. F., Hoischen, A., Bayram, Y., Withers, M., Gezdirici, A., et al. (2016). DVL3 Alleles resulting in a -1 frameshift of the last exon mediate autosomal-dominant robinow syndrome. Am. J. Hum. Genet. 98, 553–561. doi: 10.1016/j.ajhg.2016.01.005
White, J., Mazzeu, J. F., Hoischen, A., Jhangiani, S. N., Gambin, T., Alcino, M. C., et al. (2015). DVL1 frameshift mutations clustering in the penultimate exon cause autosomal-dominant Robinow syndrome. Am. J. Hum. Genet. 96, 612–622. doi: 10.1016/j.ajhg.2015.02.015
Witte, F., Bernatik, O., Kirchner, K., Masek, J., Mahl, A., Krejci, P., et al. (2010). Negative regulation of Wnt signaling mediated by CK1-phosphorylated Dishevelled via Ror2. FASEB J. 24, 2417–2426. doi: 10.1096/fj.09-150615
Wynshaw-Boris, A. (2012). Dishevelled: in vivo roles of a multifunctional gene family during development. Curr. Top. Dev. Biol. 101, 213–235. doi: 10.1016/B978-0-12-394592-1.00007-7
Yu, A., Rual, J.-F., Tamai, K., Harada, Y., Vidal, M., He, X., et al. (2007). Association of Dishevelled with the clathrin AP-2 adaptor is required for Frizzled endocytosis and planar cell polarity signaling. Dev. Cell 12, 129–141. doi: 10.1016/j.devcel.2006.10.015
Zhang, M., Hagenmueller, M., Riffel, J. H., Kreusser, M. M., Bernhold, E., Fan, J., et al. (2015). Calcium/calmodulin-dependent protein kinase II couples Wnt signaling with histone deacetylase 4 and mediates dishevelled-induced cardiomyopathy. Hypertension 65, 335–344. doi: 10.1161/HYPERTENSIONAHA.114.04467
Keywords: Dishevelled, Wnt signaling, vertebrate embryonic development, embryonic expression, autosomal dominant robinow syndrome
Citation: Gentzel M and Schambony A (2017) Dishevelled Paralogs in Vertebrate Development: Redundant or Distinct? Front. Cell Dev. Biol. 5:59. doi: 10.3389/fcell.2017.00059
Received: 31 January 2017; Accepted: 12 May 2017;
Published: 26 May 2017.
Edited by:
Andrea Erika Münsterberg, University of East Anglia, United KingdomReviewed by:
Christine Hartmann, University of Münster, GermanyVictoria Sherwood, University of Dundee, United Kingdom
Copyright © 2017 Gentzel and Schambony. This is an open-access article distributed under the terms of the Creative Commons Attribution License (CC BY). The use, distribution or reproduction in other forums is permitted, provided the original author(s) or licensor are credited and that the original publication in this journal is cited, in accordance with accepted academic practice. No use, distribution or reproduction is permitted which does not comply with these terms.
*Correspondence: Marc Gentzel, marc.gentzel@tu-dresden.de