Integrated CO2 Capture and Utilization Using Non-Thermal Plasmolysis
- UK Centre for Carbon Dioxide Utilisation, Department of Chemical and Biological Engineering, University of Sheffield, Sheffield, United Kingdom
In this work, two simple processes for carbon dioxide (CO2) such as capture and utilization have been combined to form a whole systems approach to carbon capture and utilization (CCU). The first stage utilizes a pressure swing adsorption (PSA) system, which offers many benefits over current amine technologies. It was found that high selectivity can be achieved with rapid adsorption/desorption times while employing a cheap, durable sorbent that exhibits no sorbent losses and is easily regenerated by simple pressure drops. The PSA system is capable of capturing and upgrading the CO2 concentration of a waste gas stream from 12.5% to a range of higher purities. As many CCU end processes have some tolerance toward impurities in the feed, in the form of nitrogen (N2), for example, this is highly advantageous for this PSA system since CO2 purities in excess of 80% can be achieved with only a few steps and minimal energy input. Non-thermal plasma is one such technology that can tolerate, and even benefit from, small N2 impurities in the feed, therefore a 100% pure CO2 stream is not required. The second stage of this process deploys a nanosecond pulsed corona discharge reactor to split the captured CO2 into carbon monoxide (CO), which can then be used as a chemical feedstock for other syntheses. Corona discharge has proven industrial applications for gas cleaning and the benefit of pulsed power reduces the energy consumption of the system. The wire-in-cylinder geometry concentrates the volume of gas treated into the area of high electric field. Previous work has suggested that moderate conversions can be achieved (9%), compared to other non-thermal plasma methods, but with higher energy efficiencies (>60%).
Introduction
Research into carbon dioxide capture and recovery for geological storage (CCS) as a greenhouse mitigation technique has seen a rise in interest over recent years. CCS is a potential mitigation option and could lead to significant reduction of greenhouse gas emissions in the electricity supply system, however, to effectively reduce global CO2 emissions this technology must be used in conjunction with other methods (Viebahn et al., 2007; Pehnt and Henkel, 2009; Pires et al., 2011). Rather than treating CO2 as a waste, the ideal solution would be to treat it as a commodity and utilize the ample quantity of CO2 available for the production of marketable products, or for use in applications where the CO2 feedstock is generated from fresh sources (Edwards, 1995; Olah et al., 2009; Peters et al., 2011). There are numerous chemical reactions for synthesizing organic molecules from CO2, some of which are shown in Figure 1.
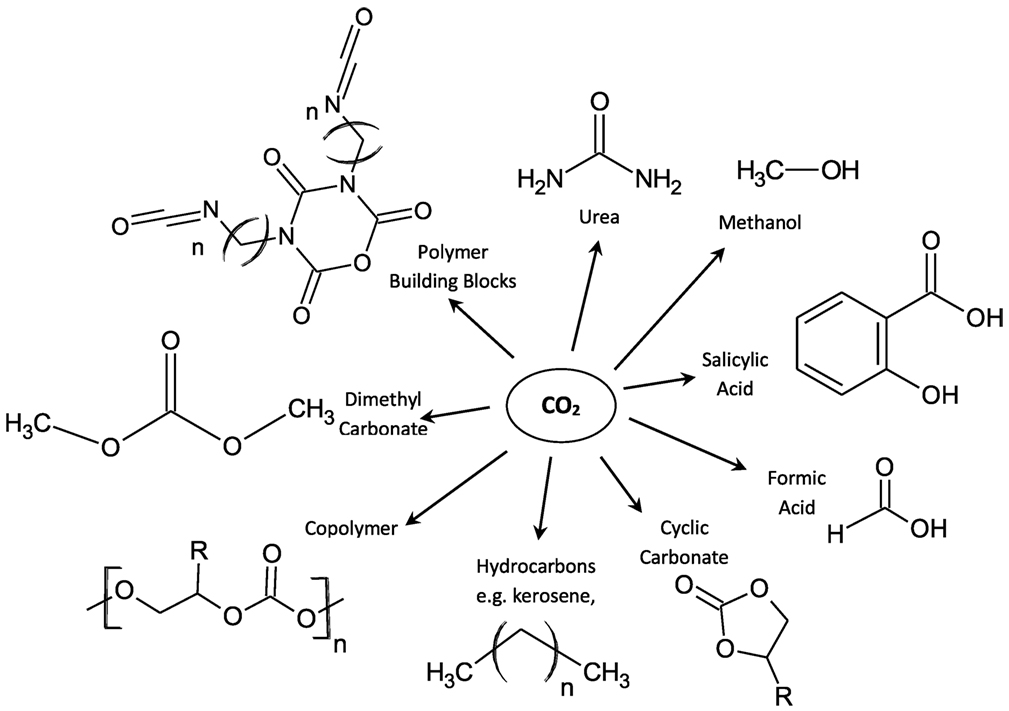
Figure 1. An overview of potential pathways for use of CO2 as a C1 building block [adapted from Styring et al. (2011)] (CO2Chem Media & Publishing have granted permission for the adaptation of this image for use in this study).
Regardless of the CO2 end use, be it CCS or carbon capture and utilization (CCU), in most cases the CO2 must first be captured. The capture step contributes to 75% of the overall cost if CCS is to be used and will increase electricity production cost by around 50%, so finding the most efficient capture method is extremely important (Yang et al., 2008). Targeted large point source emitters such as power stations would benefit from the convenience of a post-combustion capture process due to the ease of retrofitting. Technologies such as cryogenics, membrane separation, and algal-based systems are all potential options; however, they are currently in their infancy and not considered economically viable at this stage (Yang et al., 2008; MacDowell et al., 2010; Bhown and Freeman, 2011). The current state of the art technology for CO2 capture is by amine absorption in a temperature swing absorption (TSA) process, but this is not without its drawbacks. High sorbent regeneration costs and sorbent losses due to evaporation and degradation mean that further advancements are required. TSA process generally produce a humid stream of pure CO2 which will require drying before it can be stored or utilized as an intermediate; this drying step is also very energy intensive. More recent research into pressure swing adsorption (PSA) for CO2 capture using solid sorbents has generated promising results (Ho et al., 2009; Wang et al., 2011; Grande, 2012). PSA has many advantages over traditional systems in that it has a much smaller plant footprint, can accept changing feed compositions and is a stop–start technology making it very flexible with regard to the plant that is supplying it.
Upon capturing CO2, the main challenge facing utilization is overcoming its thermodynamic stability to form further products. Reactions of CO2 generally require elevated temperatures and pressures to bring about a reaction and thus can incur large energy penalties, reducing the profitability of the CO2-derived products.
Centi and Perathoner (2009) have stated that although there are many options to utilize CO2, including but not limited to, mineralization, chemical production, fuel production, carbonation, and microalgae, the underlying problem is that often these processes require a pure or high purity CO2 feedstock before conversion can take place. All technologies have their own advantages and disadvantages, but few have successfully made the leap from small to industrial scale. Mineralization is the one of the most widely adopted methods of CCU with an estimated 50 Mt/year of CO2 used in the production of inorganic carbonates in 2013, according to Aresta et al. (2013). Combined with mineralization, urea production together accounted for over 90% of all utilized CO2 in 2013; however, they represent a small dent in the global emissions of around 35 Gt/year. It is clear that CO2 can be used to create all of the products shown in Figure 1 but cheaper, less energy intensive alternative processes are currently more favorable. If a low energy capture and utilization process could be found, with the added advantage of being able to turned on and off at will, then surplus renewable energy could be used to power the system as suggested by Goede (2015). If this work, which targets CO as its product, can produce an activated form of CO2 with minimum energy inputs it goes a long way to addressing the high energy costs associated with CCU.
To overcome the energy barrier to react CO2 an alternative is to first reduce CO2 to carbon monoxide (CO); a more reactive molecule. The energy barrier for this reduction can be reduced through the use of non-thermal plasma. Under thermal conditions temperatures in excess of 3,000 K are required to split CO2 into CO. However, in non-thermal plasma only the electron temperature in the gas is elevated so the reaction can be performed at atmospheric temperature and pressure (Fridman, 2008). Plasma offers an advantage over other utilization methods as it relatively low energy cost to activate CO2. It is also highly flexible and has been proven to accept a wide range of gas mixtures with conditions tunable to match the provided load. In conjunction with PSA, plasma technology can be easily switched on and off when in demand rather than operate as a continuous process, although it is capable also of doing this. Various studies into CO2 reduction in non-thermal plasmas have been conducted in different plasma systems (Savinov et al., 2002; Indarto et al., 2007; Aerts et al., 2012; Bogaerts et al., 2015; van Rooij et al., 2015; Sun et al., 2017). However, to-date, there have been no literature reports of any integrated capture and utilization processes detailed in their published work.
To the best of our knowledge, this new whole system approach to CCU has never been reported in the literature and combines together two highly promising technologies that have demonstrated in previous work to be low in energy costs. On paper, both technologies are individually suited to accepting a variable feed composition and intermittent load (which could match fluctuating energy supply if renewable energy was used). Although, in this work, both are currently operated on a small scale, they have good potential for scale-up to process a large quantity of gas. Corona discharge is already established on an industrial scale in electrostatic precipitators, and the PSA system is expected to scale linearly with size; however, further investigation of the ability to manufacture sorbents on large scale must be undertaken (Roth, 2001). This work aims to provide a foundation for future CCU processes and advocates a whole systems approach to assessing these technologies, paying close attention to the purity of CO2 required for each utilization step.
In this work, a high PSA system is used to first capture, then upgrade the composition of flue gas to high purity. After capture the CO2 is released and passed through a pulsed corona discharge reactor wherein electron initiated dissociation converts the CO2 to CO, other products are formed but as the aim of this paper is to provide a proof of concept the detailed kinetical pathway is not discussed further. Using non-thermal plasma technology, this process aims to provide a source of non-fossil fuel derived carbon for chemical feedstocks.
Method
Pressure Swing Adsorption
First, a bench scale single bed PSA system was used to extract CO2 from a simulated flue gas stream containing 12.5% CO2 and 87.5% N2. This composition sits well within the range expected as an output from coal fired power stations, for example, found in the UK (Naims, 2016; von der Assen et al., 2016). This PSA system makes use of a cheap and robust solid sorbent, which is based on previously reported poly-ionic liquid sorbents by this group (Supasitmongkol and Styring, 2010; Dowson et al., 2016), which selectively adsorbs CO2 on pressurization of the gas stream. As the pressure is released, the N2 and CO2 desorb from the sorbent surface at different rates, thus creating two separate streams; one rich in N2 followed by one rich in CO2. An experimental diagram of the process is shown schematically in Figure 2. High-pressure adsorption experiments were carried out in a bespoke stainless steel packed-bed adsorber column constructed using Swagelok™ 1/2″ tubing 100 mm in length. A cross section of which is shown in Figure 3. The column is compression sealed and can withstand pressures up to 200 bar. The sorbent is packed inside with quartz wool filters used to avoid sorbent carry over during testing.
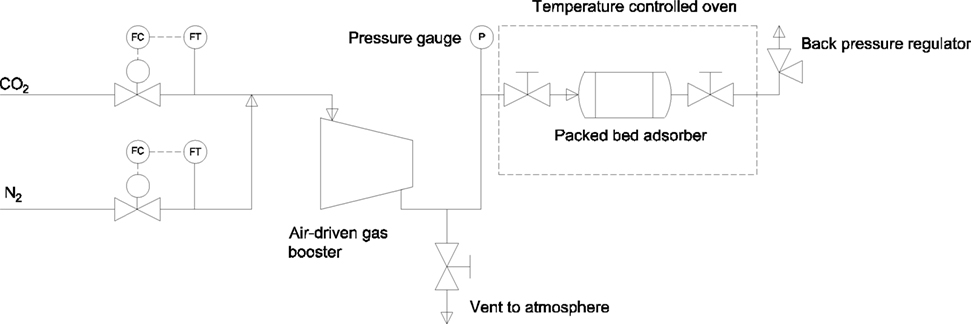
Figure 2. Pressure swing adsorption experimental rig used for measuring sorbent capacity, rate, and separation potential.
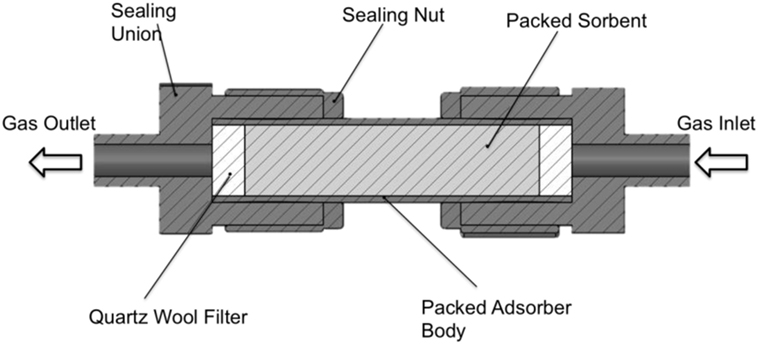
Figure 3. Cross-sectional view of the packed-bed adsorber used for CO2 separation from simulated flue gas streams.
Flue gas streams were pressurized to 15 bar and allowed to adsorb for 15-min cycles. Full methodology was as described previously for solid ionic liquid sorbents (Dowson et al., 2016). A two-stage depressurization of the adsorber leads to two gas streams. This CO2-rich stream would be sent directly to the corona reactor for reduction to CO.
Pulsed Corona Discharge
A simplified experimental diagram of the downstream plasma utilization section is presented in Figure 4. Experiments were performed at atmospheric pressure and ambient temperature. The reactor used was a wire-to-cylinder corona discharge reactor constructed using a stainless steel cylinder as the outer ground electrode (R = 17 mm), and a narrow tungsten wire as the inner live electrode (r0 = 125 µm). The total reactor volume was approximately 300 cm3.
Plasma ignition was provided by means of a high voltage pulse generator (NPG18-3500N Megaimpulse Ltd.) (Lyublinsky et al., 2013). Electrical measurements were taken using a high voltage probe (Tektronix P6015A) connected to the live electrode to measure the voltage and a wide band current transformer (Pearson 6595) to measure the current. These two parameters enabled the energy and power used during the utilization process to be calculated. The unique aspect of this power supply is the ability to send a series of short pulses approximately 40 ns in width over a microsecond at a repetition rate that can be varied up to 3,200 Hz. These short pulses remove the need for a dielectric layer, prevent gas heating (thus increasing the efficiency of CO2 splitting), and reduce the possibility of plasma arc development. The applied voltage signal is shown in Figure 5. It is characterized by a primary short pulse of very high amplitude proceeded by a series of smaller short pulses of lower amplitude. The corresponding output current is also shown in Figure 6.
Following capture from the PSA rig, the CO2-rich flue gas was passed to the pulsed plasma reactor wherein it was subjected a corona discharge for varying residence times. The residence time was altered by changing the gas flow rate (Q) into the plasma reactor between 100 and 400 mL/min by using mass flow meters (Bronkhorst) which corresponds to residence times of 37.5, 75, and 150 s. Gaseous products from the corona reactor were measured by means of a Fourier transform infrared (FTIR) spectrometer (Varian 660-IR Agilent) post discharge. No liquid products were observed. A typical absorbance spectrum is shown in Figure 7. The method used to quantify the production of CO was performed by calibrating the FTIR with known quantities of CO to generate a calibration curve. Before plasma ignition a background spectrum of the CO2 or CO2/N2 mixture was taken. After being exposed to the plasma, another spectrum was taken, and the background spectrum subtracted to determine the absolute IR absorbance. This absorbance was then compared to a known value along the calibration curve, and the quantity of CO was found. The main products detected were CO, NO, NO2, N2O, N2O5, and N2O3 with CO the dominant product. As the most important species as far as utilization is concerned, only the conversion of CO2 to CO was calculated. In addition, the values of other products remained in the parts per million range so were viewed as minor importance. Consideration into separation of all the product gases requires further work and is outside the scope of the article. This is in contrast to the works of Heijkers et al. (2015) who observed effective conversions up to 15% of N2 to other products, although in that work the specific energy input (SEI) peaked at 7.1 eV/molecule compared to the maximum of 0.43 eV/molecule used in this work.
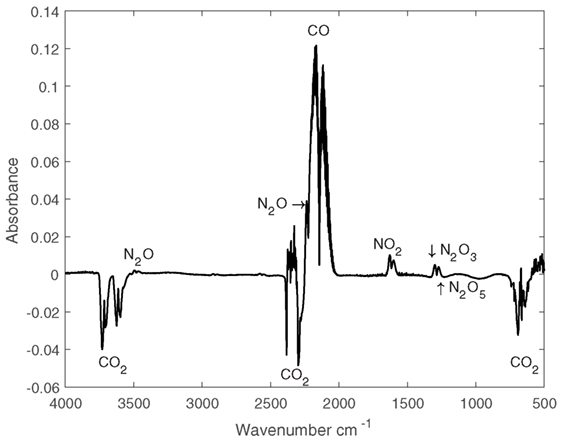
Figure 7. Sample Fourier transform infrared absorbance spectrum subtracted from background spectrum for 50:50 CO2:N2 mixture.
To evaluate the effectiveness of CO2 utilization, two important and commonly used parameters will be deployed. These are the conversion factor (X) and energy efficiency (η) as defined by Eqs 1 and 2, respectively. The conversion is defined as the change in CO2 concentration over the initial CO2 concentration. As the production of other carbon containing species is negligible, this can be said to approximate the CO yield. From this, effective conversion (Xeff), which is also used in literature, can be found as a product of X and the relative CO2 content in the feed. For example, if a gas contains 80% CO2, the conversion is multiplied by 0.8.
The energy efficiency is defined as a ratio between the dissociation enthalpy of CO2 (ΔHR) as shown in Eq. 3 to the energy penalty to produce one molecule of CO (ECO).
Equation 2 is usually expressed in the literature in terms of the SEI, or the ratio of discharge power to the gas flow rate (SEI = W/Q) (J/cm3) (Eq. 4). Discharge power is defined as the total energy supplied over the residence time (W = E/tg) (J/s). For calculation of total energy, see Eq. 6.
To calculate ECO (J), the total energy (E) must be calculated during the residence time of the gas as a ratio to the number of CO molecules that have been subjected to corona discharge (NCO),
where NCO can be expressed as follows:
The total energy deposited is calculated as shown in Eq. 6. It is the product of the energy applied during a single pulse (Epulse) (J), the gas residence time (tg) and the pulse repetition frequency (f) (Hz). Epulse can be further calculated from the integral of the applied voltage and current over the duration of a pulse (τ).
From previous work (Moss et al., 2017), it is known that the shape of the applied voltage waveform heavily influences the energy deposited within the plasma reactor and it can affect the chemical kinetic pathway of CO2 utilization. Under all experimental conditions, the repetition frequency was fixed at 1,700 Hz, as higher values led to instabilities in the discharge, increasing the temperature of the gas and thus reducing the energy efficiency of utilization. In this work, the energy deposited in the gas was altered by modifying the residence time of the gas only and estimated to be between 1 and 2 mJ across all gas mixtures.
Coupling of the Two Processes
Bringing the two processes together to create a single utilization system is a simple matter with only the addition of an intermediate storage vessel between the two systems. The proposed system is shown schematically in Figure 8.
As separate systems, these two processes effectively work as stop–start technologies, i.e., they can readily be turned on and off. Joining them into a continuous process does not change this and allows the overall system to be highly operationally flexible. Not only operational time but also flexibility is provided in the form of the feed gas accepted into the process. The PSA system can accept variable feed flow rates and compositions with little effect to the process; similarly, the corona reactor can accept various feed flow rates and compositions while still effectively producing the desired product; albeit at the cost of varying separation. The addition of an intermediate storage vessel between the two systems is required to act as a buffer for the cyclic nature of the PSA product stream, thus allowing a constant feed to the corona reactor to be maintained.
Results and Discussion
Outputs from the Capture Process
To generate the product streams for the corona reactor, the PSA system uses a poly-ionic liquid solid sorbent, which allows separation of CO2 from a simulated flue gas stream due to the difference in desorption rate of CO2 and N2 from the sorbent. N2 is desorbed instantly, whereas CO2 is held for longer periods of time giving a clear separation of the two gas streams. Although the exact mechanism of this desorption behavior is not fully understood, the physical nature of the adsorption in this case leads to weak physical interactions with the CO2 molecule. Although there is some interaction with N2, it is almost negligible and decreasing the system pressure fully reverses it, allowing almost instantaneous release of N2 from the system. It is likely that CO2 desorption rate is a function of the system pressure, and as the pressure drops, the weak interactions formed between the molecules and the surface of the sorbent are continually broken as the pressure is reduced with the most weakly bound molecules leaving the system first. Desorption rate was determined for each gas individually by first allowing gas adsorption for 15 min at 15 bar. Previous work (Dowson et al., 2016) has shown that after this 15-min period, the sorbent will be fully saturated at 100% and will adsorb no more as shown in Figure 9. In fact, adsorption rates for some of the ionic liquid sorbents were found to be mere seconds. The pressure was then released from the adsorber and the sorbent weight was tracked until a steady state was reached and desorption was completed.
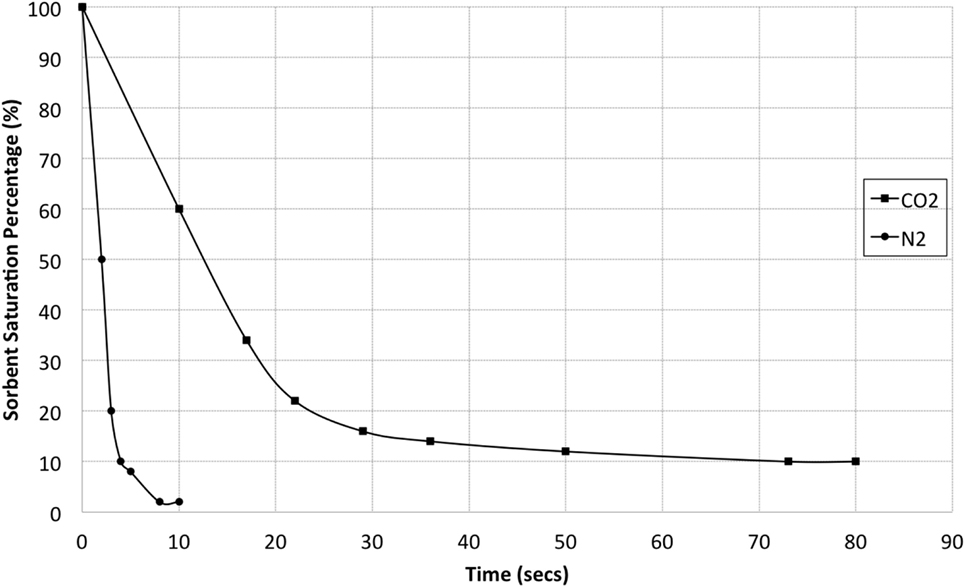
Figure 9. Rate of desorption for carbon dioxide and nitrogen from the sorbent over time during a single pressure drop from 15 bar down to 1 bar.
As can be seen from Figure 10 during certain points of the desorption step, both gasses will be released at the same time therefore, after one pass, it is clear that a 100% stream of both N2 and CO2 cannot be achieved. Further passes would be required to gradually increase the CO2 concentration to higher percentages. It is also thought that the instantaneous desorbing N2 will in fact pull some of the weakly bound CO2 with it as it flows through the packed bed. To determine the CO2 composition of the gas in the outlet, a mixed gas stream of 12.5% CO2 and 87.5% N2 was produced using Bronkhorst mass flow controllers. This gas mixture was then pressurized using a Midwest Pressure Systems 40 series air driven gas booster to 15 bar and left to adsorb for 15 min with the reactor held at a steady temperature of 25°C. The pressure within the adsorber was then released gradually using a JASCO MODEL BP-1580-81 programmable electronic back pressure regulator with a gas flow rate of approximately 50 mL/min and allowed to flow through a COZIR Sprint IR inline infrared sensor to determine the CO2 composition.
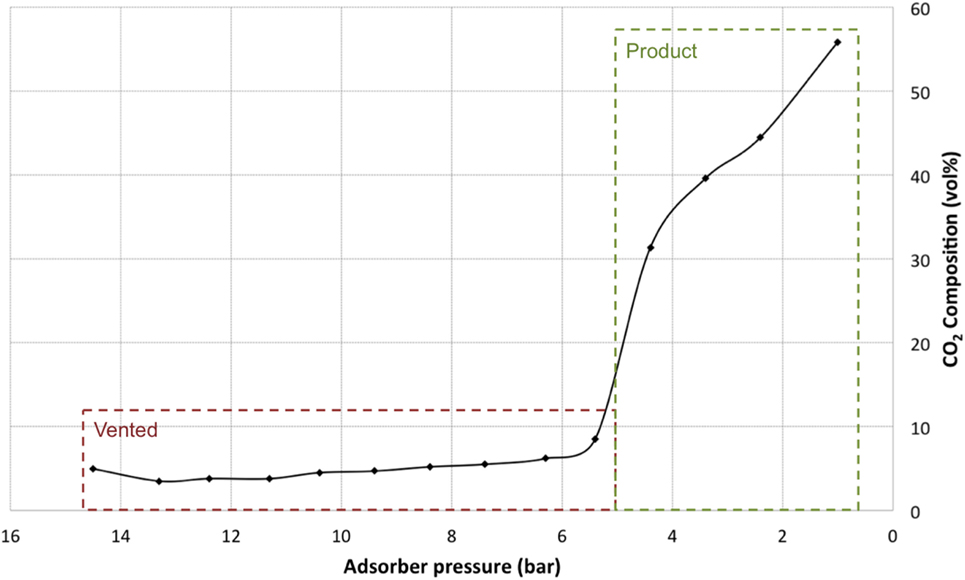
Figure 10. CO2 composition at the adsorber outlet with a gradual gas desorb of 50 mL/min with pressure dropping from 15 to 1 bar with an initial starting CO2 concentration of 12.5%.
These initial tests show that there are two distinct stages within the pressure drop, as the pressure drops from 15 down to approximately 5 bar, the CO2 concentration is low and N2 desorption dominates, this stage would is vented as a “waste” gas. At 5 bar, there is a sharp gradient shift as CO2 starts to desorb, this stage is product gas. The peak concentration of the product gas is around 55% CO2 with an average composition of around 44% CO2 in the total volume of gas released. This product gas can then be sent to the corona reactor directly, or can be recycled for a second pass of the adsorber. Figure 11 shows measured gas compositions with ranging CO2 inputs and outputs to show the flexibility of the system. To generate these data, the pressure was dropped instantaneously using the back pressure regulator to mimic the two stages previously mentioned allowing for a combined adsorption/desorption cycle time of around 5 min to be achieved. The output results shown are the average composition from stage 2 of the pressure drop.
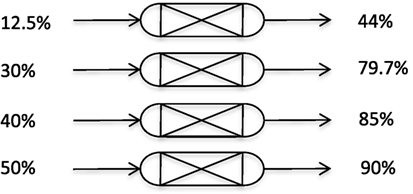
Figure 11. Various composition range inputs and outputs from the pressure swing adsorption adsorber.
Higher concentrations of product gas were obtained by doing multiple passes of the packed-bed adsorber. It is shown that with some optimization, a product output over 90% CO2 could be achieved with just two passes of the adsorber if the utilization system required it.
Results from Pulsed Corona Reactor
Figure 12 shows the absolute and effective CO2 conversion produced during pulsed corona discharge against the gas residence time for various gas mixtures of CO2 and N2. It is evident that for all gas mixtures increasing, the residence time has a positive effect on the conversion. Initial observation suggests that there is no immediate correlation between gas mixture and conversion; however, it can be seen that a small quantity of N2 enhances CO2 conversion, but further increase leads to a reduction in conversion. Examining effective conversion shows raw flue gas (12.5% CO2) and 100% CO2 perform the worst across all gas mixtures, which is highly beneficial for the proposed process as it proves raw flue gas to be unsuitable for utilization and that pure CO2 is not required. Ideally, an upgraded flue gas feed is most suitable in terms of conversion for this non-thermal plasma system, which is exactly what the PSA system used in this work provides.
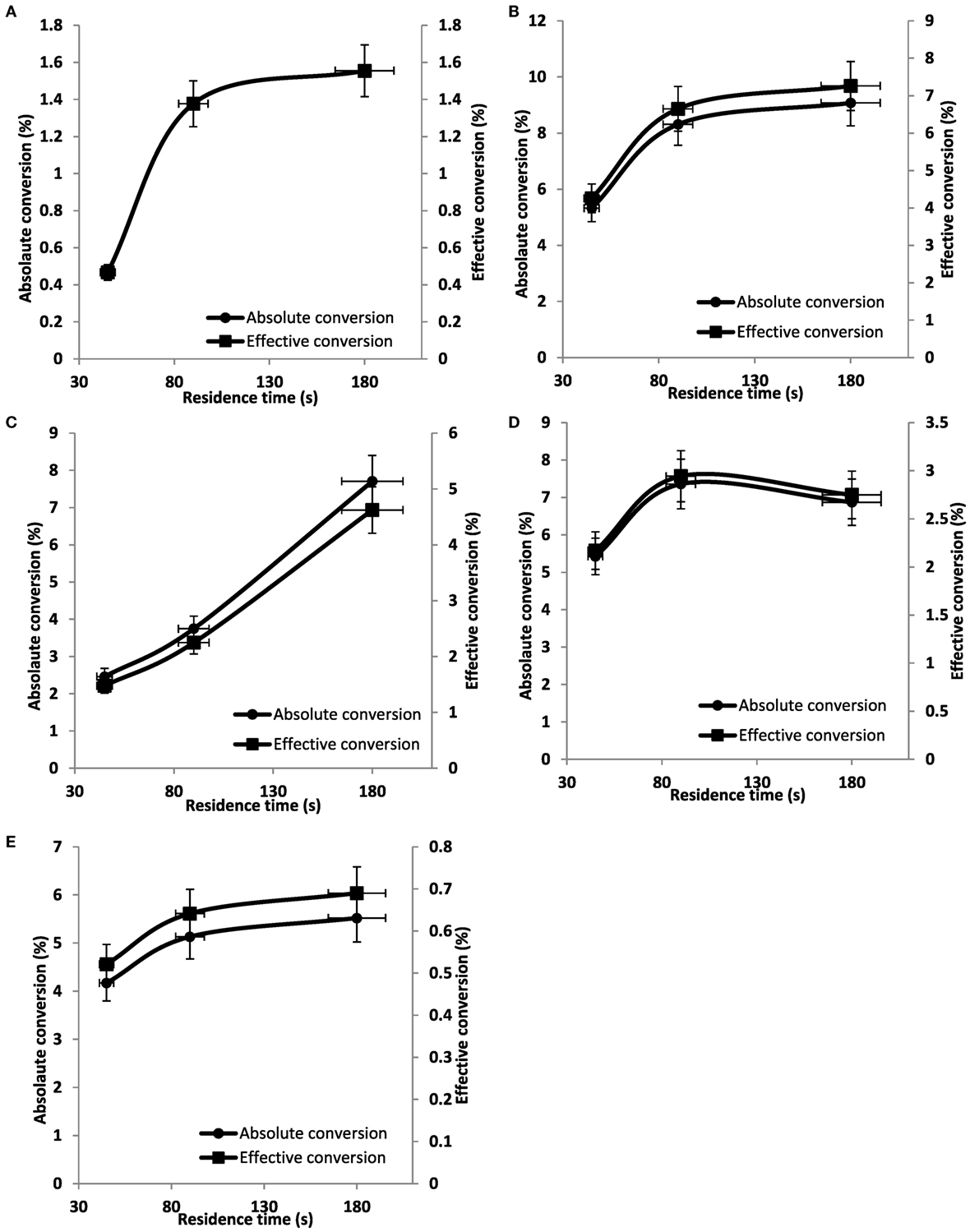
Figure 12. Effec tive and absolute conversion of CO2 to CO as a function of residence time: (A) 100% CO2, (B) 80% CO2, (C) 60% CO2, (D) 40% CO2, and (E) 12.5% CO2.
Peak effective conversions of approximately 8% were found in an 80% CO2/20% N2 mixture. Snoeckx et al. (2016) observed a similar effect using a dielectric barrier discharge (DBD) plasma reactor although the reported conversions were slightly higher, peaking at 12% for a 25% CO2/75% N2 mixture. However, it should be noted that the conversions reported in that work were achieved at significantly higher SEIs approximately one order of magnitude higher than this work. Transfer of energy from vibrationally excited N2 has been proven to be effective in enhancing the conversion of CO2 in DBD systems and it is theorized that the same effects are exhibited in a pulsed corona, although the extent of this requires further investigation. Pontiga et al. (2011) also investigated mixtures of CO2 and N2 in a DC corona discharge. However, in that work, the conversion to CO was only in the order of a few percent. They attributed the lower conversion upon increasing N2 concentration to the formation of nitrous oxides (NOx), which inhibit the formation of CO through reactions such as Eq. 7, which could prove a limiting factor.
Heijkers et al. (2015) observed a large conversion of N2, in addition to CO2, in a microwave plasma. N2 conversions up to 15%, at peak SEI of 7.1 eV/molecule, was observed, which is significantly higher than those reported in this work where the total NOx products formed was in the order of hundreds of parts per million. This observation can be attributed to the lower specific input energy used during pulsed corona discharge operation which is typically one order of magnitude lower than other plasma technologies. As less total energy is applied the amount available to convert N2 is also less; this combined with a higher energy threshold to ionize N2 over CO2 results in low NOx production.
Figure 13 shows the energy efficiency of CO2 conversion under plasma conditions as a function of the SEI. For all mixtures, except 60% CO2/40% N2, the efficiency decreases as the SEI is raised, i.e., the residence time is increased. As demonstrated in previous work (Moss et al., 2017), the efficiency is substantially higher than other reported works at low SEIs, and this remains the case in CO2/N2 mixtures, peaking at approximately 90% albeit with a large error. Again compared to the works of Snoeckx et al. (2016), the highest reported efficiency was 13% in a 75% CO2/25% N2 mixture. It is noted that the gas mixture containing 60% CO2 exhibits a different trend compared to the others and this is attributed to a different kinetic pathway of reduction but to validate this would require an in-depth kinetical analysis.
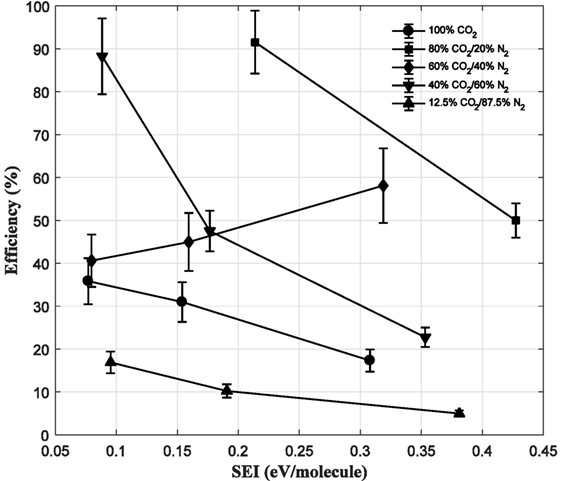
Figure 13. Energy efficiency as a function of specific energy input for different gas mixtures (1 eV/molecule = ca. 3.933 J/cm3 under standard temperature and pressure).
Taking the case of pure CO2, the energy efficiency is again much higher than other works with a maximum around 35% being achieved at the lowest SEI/longest residence time. Except for raw flue gas, N2 addition has a positive effect on efficiency, and at higher values of SEI, as the N2 content is decreased the efficiency increases. It is theorized that up to 20% N2 content in the gas mixture has a positive effect on CO2 conversion and efficiency owning to the formation of N2 metastables, which are effective at transferring energy to lower energy states of CO2. Further increase in N2 quenches these metastables, and the beneficial effect is no longer observed. Furthermore, as the gas content contains more N2, a higher energy requirement is needed to ignite and sustain the plasma, and more energy is directed toward reactions of N-containing species and diverted away from CO2 dissociation. This effect becomes dominating as more N2 is added as demonstrated in other works (Heijkers et al., 2015).
The main reason for higher efficiencies being achieved has been stated in previous work and proven by means of numerical modeling (Moss et al., 2017). The unique form of the applied voltage pulse, characterized by an initial large amplitude pulse followed by a series of smaller amplitude pulses, allows a two-step pathway of CO2 utilization to be established. During the high amplitude pulse, ionization and vibrational excitation of the gas are the dominant reactions; however, during the series of smaller amplitude pulses, dissociation of neutral and vibrationally excited CO2 reactions dominates, which is the most direct route to produce CO. In contrast to other works, the role of vibrational excitation and subsequent dissociation is not expected to play a major role in the formation of CO; as the relaxation times of such species are shorter than the inter-pulse duration. N2 vibrational species may play a role in enhancing CO2 conversion as shown in the literature but without a detailed kinetic model of this type of plasma discharge it cannot be conclusively stated. Small quantities of N-containing and O-containing molecules are expected to lower the overall conversion and efficiency as energy will be diverted toward reactions of these components but as the quantity of these is small their effects on conversion and efficiency are minimal.
Conclusion
Carbon dioxide capture by pressure swing absorption and conversion by non-thermal plasma has been demonstrated to be a viable process for the production of CO albeit on a small scale, a valuable precursor to many chemicals and key feedstocks for the chemicals industry. CO2 can be captured from raw flue gas and upgraded to ca. 40% purity in only one stage and fed into the corona discharge reactor to achieve approximately 7% absolute conversion to CO across a range of feed rates and with an average energy efficiency of 54%. Alternatively, the upgraded flue gas can enter a second stage in the capture process and be further refined to around 80%. This high purity CO2 when fed into the corona reactor is capable of producing a product gas containing approximately 8% CO with an average energy efficiency of 71%.
The main advantage over this route to CO, although conversions are relatively low and even for plasma technology this conversion is average but the fact that linking the two processes together allows a variable waste gas stream to be captured and then upgraded to obtain a higher concentration of CO2 with some conversion to a useful product. For the utilization stage, it has been discovered that, unlike other technologies, a pure CO2 feed is not only not required but it is unfavorable. Flexibility of feed gases and flexible operating conditions are an additional benefit over most other utilization technologies as they favor continuous/continuous-batch operation. It should be remembered that low conversions are common in the process industries and simply require effective recycling of the unreacted reagents to improve performance. The Haber–Bosch process, which is the main global route to ammonia production, is a prime example of this approach.
Although there needs to be consideration for the separation of CO from the product gas, this process suggests that a low energy route to CO may be achievable. Both the PSA process and pulsed corona reactor are non-intensive in terms of energy usage; however, for the process to be realized on a larger scale, recycling of the unreacted CO2 in the product gas must be deployed. Membrane separation technology offers a potential solution to this problem.
There will always be concerns about how the effects of impurities such as SOx and water vapor will have on capture and utilization processes and the sensitivity of the system toward this. Future work examining these effects must be completed before the process can truly be considered for industrial application. The scale-up to pilot scale of these technologies is another step to overcome before a realistic, industrially viable process can be envisaged. It is envisaged that for the PSA system, the scale-up is linearly related to the size of the equipment; however, consideration into the feasibility of manufacturing ionic liquid sorbents on large scales must be undertaken. To successfully scale up the utilization step, it is likely that the corona reactor would employ a modular configuration of multiple reactors; however, it should be noted that increasing the reactor size would exponentially increase the energy costs.
Although this process shows promise, in terms of a complete capture and utilization system, careful consideration into the recycling and separation of the product gas and a techno-economic assessment of the whole process must be performed before larger scales can be achieved. However, it provides a useful conceptual process for forming a low energy route to a highly energetic CO product from a wide range of sources, containing various CO2 concentrations, including the potential for biogas conversion.
Author Contributions
MM was a research student working on the 4CU project and postdoctoral researcher on a CO2Chem Seedcorn Grant and specialized in plasmolysis. DR was a research student working on the 4CU project and specialized in PSA for carbon dioxide capture. PS and RA were research supervisors and investigators on the 4CU project. All the authors contributed to the writing of the paper.
Conflict of Interest Statement
The authors declare that the research was conducted in the absence of any commercial or financial relationships that could be construed as a potential conflict of interest.
Acknowledgments
The University of Sheffield and EPSRC are thanked for providing facilities and funding that enabled the work to be carried out.
Funding
This research has been funded by the EPSRC from the 4CU Programme Grant (EP/K001329/1), at the University of Sheffield. MM also wishes to acknowledge receipt of a CO2Chem Network (EP/K007947/1 and EP/H035702/1) Seedcorn grant, which enabled this work to take place.
References
Aerts, R., Martens, T., and Bogaerts, A. (2012). Influence of vibrational states on CO2 splitting by dielectric barrier discharges. J. Phys. Chem. C 116, 23257–23273. doi: 10.1021/jp307525t
Aresta, M., Dibenedetto, A., and Angelini, A. (2013). The changing paradigm in CO2 utilization. J. CO2 Util. 3, 65–73. doi:10.1016/j.jcou.2013.08.001
Bhown, A. S., and Freeman, B. C. (2011). Analysis and status of post-combustion carbon dioxide capture technologies. Environ. Sci. Technol. 45, 8624–8632. doi:10.1021/es104291d
Bogaerts, A., Kozák, T., van Laer, K., and Snoeckx, R. (2015). Plasma-based conversion of CO2: current status and future challenges. Faraday Discuss. 183, 217–232. doi:10.1039/c5fd00053j
Centi, G., and Perathoner, S. (2009). Opportunities and prospects in the chemical recycling of carbon dioxide to fuels. Catal. Today 148, 191–205. doi:10.1016/j.cattod.2009.07.075
Dowson, G. R. M., Reed, D. G., Bellas, J.-M., Charalambous, C., and Styring, P. (2016). Fast and selective separation of carbon dioxide from dilute streams by pressure swing adsorption using solid ionic liquids. Faraday Discuss. 192, 511–527. doi:10.1039/C6FD00035E
Edwards, J. H. (1995). Potential sources of CO2 and the options for its large-scale utilisation now and in the future. Catal. Today 23, 59–66. doi:10.1016/0920-5861(94)00081-C
Grande, C. A. (2012). Advances in pressure swing adsorption for gas separation. ISRN Chem. Eng. 2012, 1–13. doi:10.5402/2012/982934
Heijkers, S., Snoeckx, R., Kozák, T., Silva, T., Godfroid, T., Britun, N., et al. (2015). CO2 conversion in a microwave plasma reactor in the presence of N2: elucidating the role of vibrational levels. J. Phys. Chem. C 119, 12815–12828. doi:10.1021/acs.jpcc.5b01466
Ho, M. T., Allinson, G. W., and Wiley, D. E. (2009). Factors affecting the cost of capture for Australian lignite coal fired power plants. Energy Procedia 1, 763–770. doi:10.1016/j.egypro.2009.01.101
Indarto, A., Yang, D. R., Choi, J. W., Lee, H., and Song, H. K. (2007). Gliding arc plasma processing of CO2 conversion. J. Hazard. Mater. 146, 309–315. doi:10.1016/j.jhazmat.2006.12.023
Lyublinsky, A. G., Korotkov, S. V., Aristov, Y. V., and Korotkov, D. A. (2013). Pulse power nanosecond-range DSRD-based generators for electric discharge technologies. IEEE Trans. Plasma Sci. 41, 2625–2629. doi:10.1109/TPS.2013.2264328
MacDowell, N., Florin, N., Buchard, A., Hallett, J., Galindo, A., Jackson, G., et al. (2010). An overview of CO2 capture technologies. Energy Environ. Sci. 3, 1645. doi:10.1039/c004106h
Moss, M. S., Yanallah, K., Allen, R. W. K., and Pontiga, F. (2017). An investigation of CO2 splitting using nanosecond pulsed corona discharge: effect of argon addition on CO2 conversion and energy efficiency. Plasma Sources Sci. Technol. 26, 35009. doi:10.1088/1361-6595/aa5b1d
Naims, H. (2016). Economics of carbon dioxide capture and utilization a supply and demand perspective. Environ. Sci. Pollut. Res. 23, 22226–22241. doi:10.1007/s11356-016-6810-2
Olah, G. A., Goeppert, A., and Prakash, G. K. S. (2009). Chemical recycling of carbon dioxide to methanol and dimethyl ether: from greenhouse gas to renewable, environmentally carbon neutral fuels and synthetic hydrocarbons. J. Org. Chem. 74, 487–498. doi:10.1021/jo801260f
Pehnt, M., and Henkel, J. (2009). Life cycle assessment of carbon dioxide capture and storage from lignite power plants. Int. J. Greenhouse Gas Control 3, 49–66. doi:10.1016/j.ijggc.2008.07.001
Peters, M., Köhler, B., Kuckshinrichs, W., Leitner, W., Markewitz, P., and Müller, T. E. (2011). Chemical technologies for exploiting and recycling carbon dioxide into the value chain. ChemSusChem 4, 1216–1240. doi:10.1002/cssc.201000447
Pires, J. C. M., Martins, F. G., Alvim-Ferraz, M. C. M., and Simões, M. (2011). Recent developments on carbon capture and storage: an overview. Chem. Eng. Res. Des. 89, 1446–1460. doi:10.1016/j.cherd.2011.01.028
Pontiga, F., Yanallah, K., Moreno, H., Hadji, K., and Castellanos, A. (2011). “Negative corona discharge in mixtures of CO2 and N2: modeling and experiments.” in Ispc_20, Viana do Castelo, 2–5.
Roth, J. R. (2001). Industrial Plasma Engineering: Volume 2-Applications to Nonthermal Plasma Processing (Vol. 2). BocaRaton:CRC press.
Savinov, S. Y., Lee, H., Song, H. K., and Na, B. K. (2002). The decomposition of CO2 in glow discharge. Korean J. Chem. Eng. 19, 564–566. doi:10.1007/BF02699296
Snoeckx, R., Heijkers, S., Van Wesenbeeck, K., Lenaerts, S., and Bogaerts, A. (2016). CO2 conversion in a dielectric barrier discharge plasma: N2 in the mix as a helping hand or problematic impurity? Energy Environ. Sci. 9, 999–1011. doi:10.1039/C5EE03304G
Styring, P., Jansen, D., De Coninck, H., Reith, H., and Armstrong, K. (2011). Carbon Capture and Utilisation in the Green Economy. >Oxford: Centre for Low Carbon Futures.
Sun, S. R., Wang, H. X., Mei, D. H., Tu, X., and Bogaerts, A. (2017). CO2 conversion in a gliding arc plasma: performance improvement based on chemical reaction modeling. J. CO2 Util. 17, 220–234. doi:10.1016/j.jcou.2016.12.009
Supasitmongkol, S., and Styring, P. (2010). High CO2 solubility in ionic liquids and a tetraalkylammonium-based poly(ionic liquid). Energy Environ. Sci. 3, 1961–1972. doi:10.1039/c0ee00293c
van Rooij, G. J., van den Bekerom, D. C. M., den Harder, N., Minea, T., Berden, G., Bongers, W. A., et al. (2015). Taming microwave plasma to beat thermodynamics in CO2 dissociation. Faraday Discuss. 183, 0.233–248. doi:10.1039/c5fd00045a
Viebahn, P., Nitsch, J., Fischedick, M., Esken, A., Schüwer, D., Supersberger, N., et al. (2007). Comparison of carbon capture and storage with renewable energy technologies regarding structural, economic, and ecological aspects in Germany. Int. J. Greenhouse Gas Control 1, 121–133. doi:10.1016/S1750-5836(07)00024-2
von der Assen, N., Müller, L. J., Steingrube, A., Voll, P., and Bardow, A. (2016). Selecting CO2 sources for CO2 utilization by environmental-merit-order curves. Environ. Sci. Technol. 50, 1093–1101. doi:10.1021/acs.est.5b03474
Wang, Q., Luo, J., Zhong, Z., and Borgna, A. (2011). CO2 capture by solid adsorbents and their applications: current status and new trends. Energy Environ. Sci. 4, 42–55. doi:10.1039/C0EE00064G
Keywords: CO2, capture, utilization, pressure swing, plasma, CO
Citation: Moss M, Reed DG, Allen RWK and Styring P (2017) Integrated CO2 Capture and Utilization Using Non-Thermal Plasmolysis. Front. Energy Res. 5:20. doi: 10.3389/fenrg.2017.00020
Received: 28 February 2017; Accepted: 11 July 2017;
Published: 02 August 2017
Edited by:
Renato Baciocchi, University of Rome Tor Vergata, ItalyReviewed by:
Richard Van De Sanden, Dutch Institute for Fundamental Energy Research, NetherlandsRafael Mattos Dos Santos, Sheridan College, Canada
Copyright: © 2017 Moss, Reed, Allen and Styring. This is an open-access article distributed under the terms of the Creative Commons Attribution License (CC BY). The use, distribution or reproduction in other forums is permitted, provided the original author(s) or licensor are credited and that the original publication in this journal is cited, in accordance with accepted academic practice. No use, distribution or reproduction is permitted which does not comply with these terms.
*Correspondence: Matthew Moss, mmoss1@sheffield.ac.uk