The Good, the Bad, and the Lethal: Gene Expression and Metabolomics Reveal Physiological Mechanisms Underlying Chronic Thermal Effects in Mayfly Larvae (Neocloeon triangulifer)
- 1Graduate Toxicology Program, Department of Biological Sciences, North Carolina State University, Raleigh, NC, United States
- 2NIH Common Fund Eastern Regional Comprehensive Metabolomics Resource Core, Nutrition Research Institute, Department of Nutrition, University of North Carolina at Chapel Hill, Kannapolis, NC, United States
- 3Center of Human Health and the Environment, North Carolina State University, Raleigh, NC, United States
- 4Bioinformatics Research Center, North Carolina State University, Raleigh, NC, United States
- 5Stroud Water Research Center, Avondale, PA, United States
Temperature dictates the performance of aquatic ectotherms. However, the physiological and biochemical processes that drive thermally-mediated life history patterns (and limits) remain poorly understood because they are rarely studied simultaneously. In our previous work, we have established life history outcomes (e.g., survivorship, development time, growth rates, and fitness) in mayflies (Neocloeon triangulifer) reared at static temperatures ranging from 14 to 30°C at 2°C intervals. In this study, we conducted biochemical measurements (RT-qPCR of select genes and targeted, quantitative metabolomic profiling) on N. triangulifer mature larvae reared at temperatures associated with excellent survival and fitness (22–24°C), compromised survival and fitness (28°C), and chronic lethality (30°C—larvae survived for a few weeks but failed to emerge to adulthood). Patterns of gene expression were similar to those observed in acute ramping experiments reported previously: larvae reared at 30°C resulted in significant upregulation in the thermally responsive gene HEAT SHOCK PROTEIN 90 (HSP90) but no significant changes in hypoxia responsive genes [EGG LAYING DEFECTIVE 9 (EGL-9) and LACTATE DEHYDROGENASE (LDH)]. Additionally, primers for genes associated with energy: INSULIN RECEPTOR (IR), mechanistic TARGET OF RAPAMYCIN (mTOR), and TREHALOSE 6 PHOSPHATE SYNTHASE (T6PS) were developed for this study. IR and mTOR were significantly upregulated while T6PS showed trend of downregulation in larvae reared at 30°C. Metabolomic profiles revealed general depletion of lipids and acylcarnitines in larvae exposed to chronic thermal stress, suggesting that larvae were energetically challenged despite continuous access to food. For example, concentrations of lysoPhosphatidylcholine (lysoPC) a C20:3 decreased as fitness decreased with increasing temperature (2.3- and 2.4-fold at 28 and 30°C relative to controls). Tissue concentrations of the biogenic amine histamine increased 2.1- and 3.1-fold with increasing temperature, and were strongly and negatively correlated with performance. Thus, both histamine and lysoPC a C20:3 are potential biomarkers of thermal stress. Taken together, our results primarily associate energetic challenge with thermally mediated fitness reduction in N. triangulifer.
Introduction
A myriad of human activities and global climate change are altering the thermal regimes of freshwater ecosystems. Temperature dictates life history outcomes (Hynes, 1970; Sweeney and Vannote, 1978, 1984; Ward and Stanford, 1982; Sweeney et al., 1990; Newbold et al., 1994; Atkinson and Sibly, 1997; Mccauley et al., 2015) and geographic distributions of species (Vannote and Sweeney, 1980; Sweeney et al., 1990) through physiological processes that are not fully understood and likely vary across the diversity of life. Understanding how thermally mediated physiological processes determine the thermal limits of species requires that we address processes that occur over different time scales (Angilletta, 2009; Schulte et al., 2011; Dillon and Frazier, 2013; Verberk et al., 2013; Sweeney et al., 2018) across different phylogenetic lineages.
Insects are critical ecological components of freshwater ecosystems (Hynes, 1970) and widely used as ecological indicators (Cairns and Pratt, 1993; Johnson et al., 1993; Rosenberg and Resh, 1993; Bonada et al., 2006; Rosenberg et al., 2008). Available data suggest that insects generally follow the temperature size rule (TSR), with faster growth but smaller adult body size under warm temperatures and slower growth but larger adult body size under cold temperatures (Atkinson, 1994; Kingsolver and Huey, 2008). The trend that aquatic insects exhibit faster growth but achieve smaller adult body size (and fecundity) was first described by Sweeney and Vannote (1978). The authors studied four mayfly “winter-spring species”—Leptophlebia cupida, Ameletus ludens, Ephemerella subvaria, and Ephemerella funeralis and found that all four species produced smaller adults with fewer eggs when reared at warm temperatures than controls reared at ambient temperatures. The findings were reiterated by Atkinson (1994) (though aquatic insect exceptions were noted). Recently, Sweeney et al. (2018) used whole life cycle rearing experiments in the mayfly Cloeon dipterum to definitively demonstrate that thermal influences on larval development period (which are reduced) are stronger than thermal influences on growth rates (which are increased). However, the physiological processes that drive these patterns still remain unclear (Angilletta and Dunham, 2003). While a negative fitness trajectory associated with warming is obviously implied by the TSR, the processes that explicitly determine the chronic upper thermal limits of aquatic insect remain unknown.
The oxygen- and capacity-limited thermal tolerance (OCLTT) hypothesis proposes that the mismatch between oxygen demand and supply determines thermal limits in all ectotherms (Pörtner, 2001, 2002, 2010; Pörtner et al., 2006; Kingsolver and Huey, 2008; Klok and Harrison, 2013). While the global applicability of this hypothesis has been questioned (Klok et al., 2004; Stevens et al., 2010; McCue and De Los Santos, 2013; Verberk et al., 2016), the applicability of OCLTT to aquatic insects has been demonstrated in the context of acute thermal tolerance (Verberk and Bilton, 2011, 2013, 2015; Verberk et al., 2013). However, Kim et al. (2017) questioned whether the OCLTT applied to chronic thermal limits in the mayfly Neocloeon triangulifer. Kim et al. (2017) identified hypoxia responsive genes that were not activated by short term exposure to chronically lethal temperatures and showed no change in aerobic scope at temperatures spanning those chronic limits. Importantly, that study used whole life cycle rearing experiments across several temperatures to establish chronic upper thermal limits.
Metabolomic analyses to study insect physiology have been widely used in the last decade (Snart et al., 2015). Verberk et al. (2013) was the first to apply these techniques on critical maximal temperatures (CTmax) acute thermal ramping challenges. Chou et al. (2017) conducted an exploratory study on metabolic changes under acute thermal ramping challenges in N. triangulifer. Temperatures chosen for that study were based on chronic thermal limits, but the exposure was a single 8 h, 8°C thermal ramp. Metabolites identified as affected by thermal challenge under non-targeted metabolomic analyses were largely related but not limited to energetics and maintenance, which focused our efforts in this paper.
The parthogenetic mayfly N. triangulifer is an emerging lab model for environmental studies due its established lab rearing methods (e.g., Weaver et al., 2015), multiple generations per year and established cDNA sequence for molecular work (Kim et al., 2017). N. triangulifer are sensitive to environmental challenges and are therefore ideal for environmental studies. In the last decade, an increasing number of physiological and ecological studies have been conducted using N. triangulifer (Conley et al., 2009, 2011, 2013, 2014; Kim et al., 2012; Kunz et al., 2013; Wesner et al., 2014; Johnson et al., 2015; Soucek and Dickinson, 2015; Struewing et al., 2015; Lopez et al., 2016).
In this study, we characterize biochemical (targeted metabolomics) and gene expression (RT-qPCR) changes in larvae reared from newly hatched eggs to mature larvae under thermal conditions associated with excellent survival and fitness, poor survival and fitness, and 100% chronic lethality. In addition to study genes that have been reported by Kim et al. (2017), we developed new primers for three energetically associated genes. This study asks three primary questions: (1) Are gene expression patterns associated with short term thermal change [thermally responsive genes HEAT SHOCK PROTEIN (HSP) 40, 90, hypoxia responsive gene EGG LAYING DEFECTIVE 9 (EGL-9), and LACTATE DEHYDROGENASE (LDH)] similar to gene expression patterns associated with chronic rearing across temperatures? (2) How do energetically associated genes INSULIN RECEPTOR (IR), mechanistic TARGET OF RAPAMYCIN (mTOR), and TREHALOSE 6 PHOSPHATE SYNTHASE (T6PS) respond to chronically stressful rearing temperatures? (3) How do metabolomic profiles vary in mayfly larvae chronically reared across thermal regimes associated with altered (reduced) fitness? By linking biochemical data to thermally-driven, life history outcomes of N. triangulifer, we attempt to deepen our understanding of the physiological mechanisms underlying long term thermal effects on development.
Materials and Methods
Mayfly Rearing and Life History
At the Stroud Water Research Center (SWRC; Avondale, PA), newly hatched eggs of the parthenogenetic mayfly N. triangulifer [WCC-2 clone isolated from White Clay Creek, (Patent US5665555)] were partitioned into rearing jars at several temperatures [14–30°C (±0.05°C) at 2°C increments] using natural stream water from White Clay Creek (WCC) and natural WCC periphyton as a food source as described elsewhere (Funk et al., 2006; Kim et al., 2017; Sweeney et al., 2018). All replicate jars contained 50 larvae, with 36 jars earmarked for full life history assessment (see Sweeney et al., 2018). For survivorship measurements, one way ANOVA was performed and Tukey's multiple comparisons test was used to analyze the differences of survivorship between temperatures. The details of life history results are not the major focus in this study and will be given full attention elsewhere. The remaining 14 jars were earmarked for larval samples for the gene expression and metabolomics studies. However, larvae reared at 30°C failed to complete larval development (100% mortality, day 34), while larvae reared at 28°C also did not perform well (88.2% mortality). Therefore, we re-established rearing jars at 28°C for metabolomics and 30°C for both gene expression and metabolomics with 300 individuals each (50 larvae per jar) to produce sufficient larval tissue biomass for these studies. Because temperature had a profound influence on development time, larvae were collected based on developmental stage (close to emergence to the subimago stage). The median development time for larvae reared at 22, 24, and 28°C were 24.8 (± 0.26), 20.1 (±0.1), and 21.0 (±0.57) days, respectively. Larvae reared at these three temperatures were collected 3–5 days before its corresponding median emergence day. Larvae reared at 30°C showed delayed development and were collected on the median emergence day of larval reared at 28°C (day 21). For gene expression studies, depending on the body mass, 2 or 3 larvae were pooled for one biological replicate. For metabolomics studies, number of individuals pooled for one biological replicate varied from 14 to 35 larvae. Upon collection, all samples were flash frozen in liquid nitrogen and stored at −80°C freezer. All samples were packaged with dry ice and sent with overnight shipping to North Carolina State University (NCSU; Raleigh, NC) for gene expression and for metabolomics studies at the NIH Common Fund Eastern Regional Comprehensive Metabolomics Resource Core (ERCMRC). Biological replicates for gene expression were n = 7 (22 and 28°C) and n = 5 (30°C). Biological replicates for metabolomics were n = 4 for all temperatures (24, 28, 30°C).
RNA Isolation and qPCR Analysis
To compare the expression of genes of interest associated with poor thermal performance, primers (Table 1) were designed based on a de novo assembly of compiled N. triangulifer cDNA sequence data (both 454 and Illumina platforms) resulting in ~23,000 contigs with associated bioinformatics. Based on these sequences, we selected potential genes of interest (HSP90, HSP40, LDH, and EGL-9) (see Kim et al., 2017) and developed additional qPCR probes for the INSULIN RECEPTOR (IR), mechanistic TARGET OF RAPAMYCIN (mTOR), TREHALOSE 6 PHOSPHATE SYNTHASE (T6PS), and ELONGATION FACTOR 1 α (EF1α) gene expression (Table 1). Both EF1α and 18s rRNA were evaluated and EF1α was selected as a reference gene. Each gene of interest was inserted into a pCR2.1®-TOPO® TA vector (Life technologies) expression vector and produced sequences that were independently confirmed. All primers were designed with IDTSciTools (http://www.idtdna.com/SciTools/SciTools.aspx) and were synthesized by Life technologies, USA.
For gene expression studies, total RNA was isolated from N. triangulifer following the SV Total RNA Isolation System protocol (Promega; Madison, WI). First strand cDNA was synthesized from the same amount of each total RNA by MultiScribe™MuLV reverse transcriptase using random primers [Applied Biosystems (ABI); Carlsbad, CA] and all thermocycling was done using a Bio-Rad iCycler (Bio-Rad; Hercules, CA). Quantitative real-time PCR was performed on an ABI Prism 7700 Sequence Detection System [Applied Biosystems (ABI); Carlsbad, CA] using default parameters. Amplification mixtures were consist of 5 μL of SsoAdvanced Universal SYBR Green Supermix (Bio-Rad; Hercules, CA), 10 μM primers, 20 ng template cDNA and nuclease free water in a total volume of 10 μL. qRT- PCR conditions were 2 min at 94°C, followed by 40 cycles at 95°C for 30 s, 60°C for 30 s, and 72°C for 30 s. After the PCR reactions, the melting curve for each PCR product was determined following the manufacturer's protocols. Relative expression of each amplicon was calculated by the corrected delta delta Ct method (Pfaffl, 2001), with all expression normalized to EF1α levels in initial control samples. Relative levels of EF1α were confirmed to be approximately equal across all treatments. All temperatures were compared to 22°C (control group) and Wilcoxon Rank Sum test was performed using built-in analysis in GraphPad Prism (GraphPad Software, La Jolla, CA) to analyze the differences of gene expression between treatments and control.
LC-MS Targeted Metabolomics and Multivariate Statistical Analysis
For the metabolomics study, samples were homogenized in ice-cold, ethanol:water (85:15) solution (10 μL per 1 mg tissue) using MagNa Lyser tubes and ceramic beads for 30 s pulses at 3,500 rpm for three times. Homogenates were vortexed for 2 min at 5,000 rpm and stored in −20°C for 1 h then transferred into Lo-Bind Eppendof™ tube and centrifuged for 4 min at 16,000 rcf. Supernatant (200 μL) was transferred into a labeled Lo-Bind Eppendorf tube, frozen and lyophilized overnight. Samples were reconstituted with Ethanol:Water (85:15) to a concentration of 3 μL/mg tissue. Samples were vortexed for 10 min at 5,000 rpm, centrifuged for 4 min at 16,000 rcf and 20 μL of each was used for the Biocrates AbsoluteIDQ™ p180 kit analysis.
Targeted metabolomics was conducted using electrospray ionization liquid chromatography–mass spectrometry (ESI-LC-MS/MS) and MS/MS measurements using the AbsoluteIDQ™ p180 kit (Biocrates Life Sciences AG, Innsbruck, Austria). This kit allows for the simultaneous quantification of 188 metabolites from different compound classes. These compound classes include 40 acylcarnitines (Cx:y), 21 amino acids (19 proteinogenic amino acids, citrulline and ornithine), 21 biogenic amines, hexose (sum of hexoses–about 90–95% glucose), 90 glycerophospholipids [14 lysophosphatidylcholines (lysoPC) and 76 phosphatidylcholines (PC diacyl (aa) and acyl-alkyl (ae)], and 15 sphingolipids (SMx:y).
The AbsoluteIDQ™ p180 kit was prepared as described by the manufacturer. Briefly, 20 μL of whole larvae extract was pipetted onto the filter inserts of the 96 well kit plate (containing 13C-isotope labeled internal standards). Samples were dried under nitrogen stream and amino acids and biogenic amines were derivatized with 5% phenylisothiocyanate reagent (PITC). Samples were then dried again and metabolites and internal standards were extracted with 5 mM ammonium acetate in methanol. Next, samples were centrifuged through filter membrane and diluted with Mass spectrometric (MS) running solvent. MS analyses were carried out on an API 4000 LC-MS/MS System (ABSciex, Framingham, MA) equipped with 1100 Series HPLC (Agilent Technologies, Palo Alto, CA) using an Agilent Eclipse XDB-C18 (3.5 μm) 3.0 × 100 mm column controlled by Analyst 1.6.2 software. Multiple Reaction Monitoring (MRM) was used for the detection of analytes (amino acids and biogenic amines) and stable labeled internal standards, the latter were used as quantification reference. Lipids, sugars and acylcarnitines were semi-quantified using flow injection analysis (FIA)-MS. The acquired data was processed using Analyst 1.6.2 (AB Sciex, Framingham, MA) and MetIDQ (Biocrates Life Sciences AG, Innsbruck, Austria) software. Concentrations of all metabolites were calculated in μM.
Targeted LC-MS concentration data were used to conduct multivariate analysis with unit variance (UV) scaling and mean centering for each of the measured metabolites and custom ratios. Multivariate analysis [orthogonal partial least squares discriminant analysis (OPLS-DA)] was performed by using SIMCA 14.1 (Umetrics, Umeå, Sweden). Analytes important to the differentiation of study groups were based on Variable Influence on Projection scores (VIP), a measure of a variable's importance in the OPLS-DA model (VIP ≥ 1 and jack-knife confidence interval not including 0) and with a magnitude of fold change ≥ 2.0. Exact Wilcoxon Rank Sum test was performed for calculating p-values. Statistical analyses were performed using SAS 9.4 (SAS Institute Inc, Cary, NC).
Linking Metabolites to Life History Outcomes
To investigate how changes in metabolite concentrations associated with life history outcomes, 6 metabolites significantly affected at both 28 and 30°C high temperatures were chosen for further examination. Absolute concentrations of the 6 metabolites at different rearing temperatures from cold to warm (14, 24, 26, 28, and 30°C) were plotted with body mass or development rate. To measure body mass, adults were dried in an oven at 50°C for ≥ 2 days, then weighed on a Mettler XS105 balance (Mettler Toledo, Columbus, OH) to the nearest 0.01 mg. Development rate was calculated as 1 divided by number of days to emerge, and represents an average. Pearson's correlation were calculated to determine the strength between metabolites and body mass. Detailed methods for predicted fecundity and other life history measurements can be found in Sweeney et al. (2018).
Results
Life cycle rearing experiments established thermal reaction norms and identified temperatures favorable for survival and fitness (e.g., 22 or 24°C) and those associated with poor performance (28°C) and chronically lethality (30°C). For example, full life cycle survival was 88.9 and 86.6% for 22 or 24°C, respectively, but fell to 12.8% at 28°C (Figure 1). The details of life history results are not the major focus in this study and will be given full attention elsewhere (Funk et al., in preparation). Briefly, mean instantaneous growth rates (see Sweeney et al., 2018 for methods) increased in a linear fashion between 14 and 24°C, (y = 0.0467x+0.0698, R2 = 0.99) but began to decrease at warmer temperatures. Mean adult body mass (and associated fecundity) decreased in a linear fashion from 14 to 26°C (y = −0.0577x+2.574, R2 = 0.98). At 28°C however adult mass was 42% lower than would be predicted from this line. Thus, 28°C samples represent processes associated with large reductions in survival and fecundity.
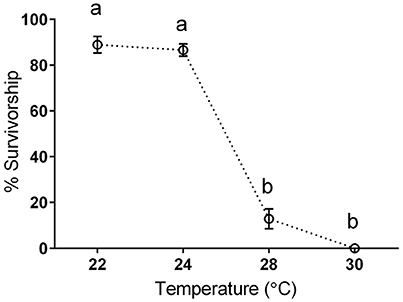
Figure 1. Survivorship of N. triangulifer full life cycle rearing at different temperatures (modified from Kim et al., 2017). Each point represents the mean survival from rearing jars [22°C (n = 11) 24°C (n = 10), 28°C (n = 5), and 30°C (n = 11)] with 50 larvae/jar to begin with. Error bars represent standard deviations from the means. One way ANOVA was performed and Tukey's multiple comparisons test was used to analyze the differences of survivorship between temperatures. Different lowercase letters suggest significant differences of survivorship between temperatures.
Gene Expression Studies
We compared mRNA expression in mayfly larvae reared at favorable (22°C), chronically stressful (28°C), and chronically lethal (30°C) temperatures (Figure 2). Genes evaluated broadly fall into three categories -indicators of thermal stress [HEAT SHOCK PROTEINs (HSPs- 40 and 90), oxygen sensing/hypoxia signaling (EGG LAYING DEFECTIVE 9 (EGL-9) and anaerobic respiration/aerobic glycolysis (LACTATE DEHYDROGENASE (LDH)], and energetics [INSULIN RECEPTOR, mechanistic TARGET OF RAPAMYCIN (mTOR), and TREHALOSE 6-PHOSPHATE SYNTHASE (T6PS)]. Genes related to thermal stress response were modestly upregulated in larvae reared at 28°C. Only HSP-90 was significantly upregulated (~3.3-fold higher than control, p = 0.005) in larvae reared at 30°C. EGL-9 and LDH were completely unresponsive to chronic thermal stress. Elevated mRNA expression was observed for the IR (~2-fold, p = 0.048) and mTOR (~2.6-fold, p = 0.003) only in larvae reared at 30°C. T6PS, though not statistically significant, was downregulated 54 and 45% in larvae reared at 28 and 30°C, respectively.
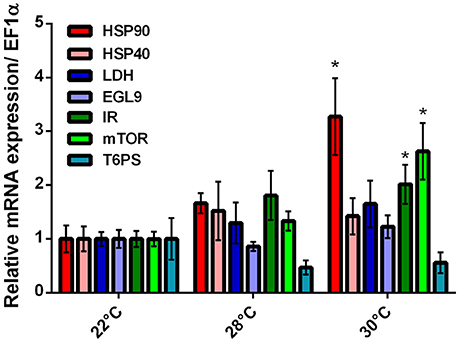
Figure 2. Relative mRNA expression of N. triangulifer larvae subjected to chronic thermal stress treatment. Genes selected for this study include thermally responsive genes HEAT SHOCK PROTEIN (HSP) 90, 40, an oxygen sensing gene EGG LAYING DEFECTIVE 9 (EGL-9), LACTATE DEHYDROGENASE (LDH), and energetically associated genes INSULIN RECEPTOR (IR), mechanistic TARGET OF RAPAMYCIN (mTOR), and TREHALOSE 6 PHOSPHATE SYNTHASE (T6PS). All data are normalized to control (22°C) ELONGATION FACTOR 1 α (EF1α). Error bars represent standard deviations from the mean (N = 7 for 22 and 28°C, N = 5 for 30°C). Wilcoxon rank sum test was performed and *Indicates significant difference compared to control (22°C).
Metabolomics
We compared metabolite profiles in mayfly larvae reared at comfortable (24°C), chronically stressful (28°C), and chronically lethal (30°C) temperatures. Multivariate data analysis (OPLS-DA) was performed to evaluate how well different groups were separated (Figure 3). Horizontal separation suggests variation between groups; vertical separation suggests variation within groups. The R2Y value in Figure 3 indicates how well the separation is between treatments. The result shows clear separation (99 and 100%) between treatment (28 or 30°C) and control (24°C) groups (Figure 3). Only the metabolites that met all three criteria (VIP ≥ 1, fold change ≥ 2.0, p < 0.05; see methods for detail) were considered as being strongly affected by chronic thermal stress and are listed in Tables 2, 3 (Datasheets 1, 2). A total of 10 metabolites were found significantly affected by thermal stress in mayfly larvae reared at 28°C (Table 2). These metabolites include increase of biogenic amine histamine (2.1-fold), decrease of 8 glycerophopholipids (2.1~3.2-fold) and decrease of 1 acylcarnitine tetradecenoylcarnitine (C14:1) (2.3-fold). A total of 24 metabolites were identified to have met the three criteria in larvae reared at 30°C (Table 3). These metabolites include 5 biogenic amines, 9 glycerophospholipids, 3 sphingolipids, 6 acylcarnitines, and 1 amino acid-ornithine. From the 24 metabolites identified in larvae reared at 30°C, all 6 acylcarnitines were less abundant (2.1 to 4.8-fold). Most of the lipids (two-thirds of both gylcerophospholipids and sphingolipids) were less abundant (2.1 to 4.6-fold) in larvae reared at 30°C.The amino acid ornithine increased 2.3-fold in response to chronic heat stress. Biogenic amine hydroxyproline (both cis- and trans-) decreased (33.5- and 2.1-fold, respectively) whereas histamine, dopamine and DOPA increased (3.1-, 14.3-, and 3.5-fold, respectively) in larvae reared at 30°C.
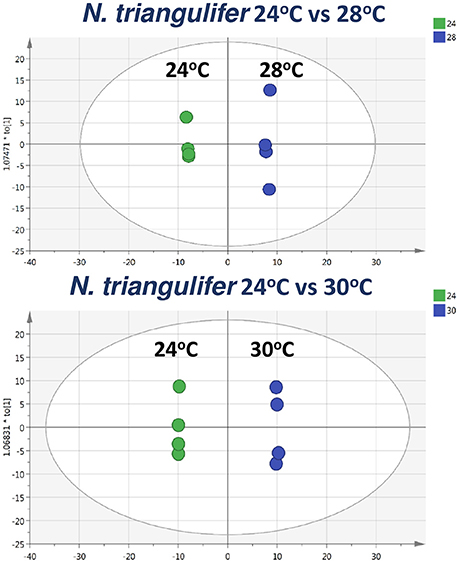
Figure 3. Scores plots of OPLS-DA of larvae reared at 24 vs. 28°C (Upper) and larvae reared at 24 vs. 30°C (Lower). The plots show the differentiating of the heat treated larvae metabolomics profiles from control larvae profiles.
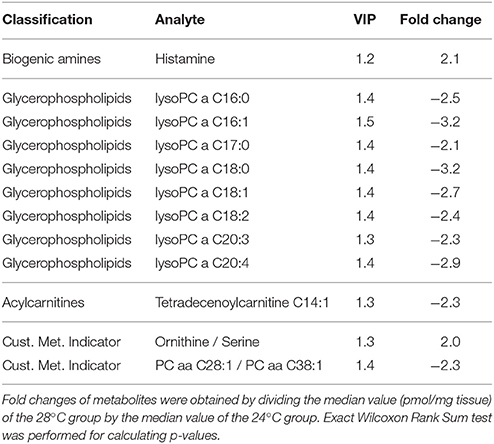
Table 2. List of metabolites identified with VIP and fold change value to have significantly changed at chronic thermal stress (28°C) when compared to control (24°C), p < 0.05.
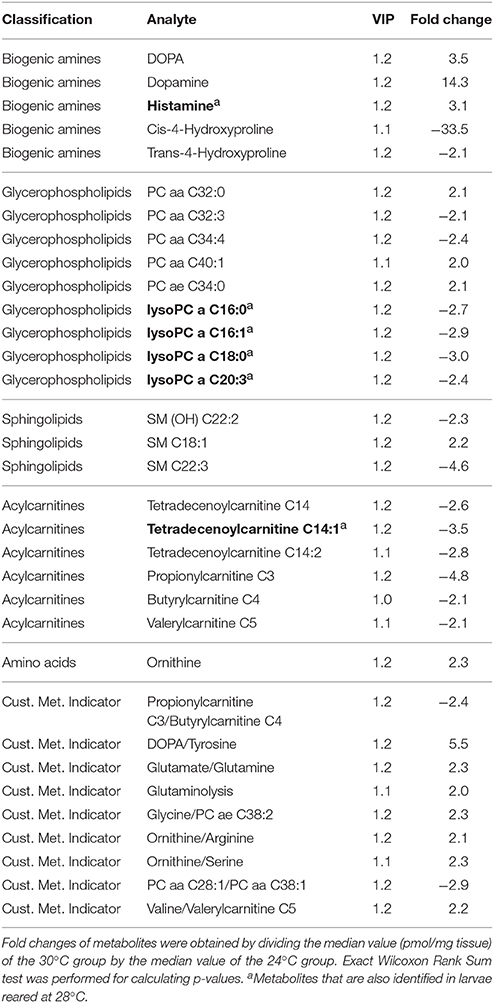
Table 3. List of metabolites identified with VIP and fold change value to have significantly changed at chronic thermal stress (30°C) when compared to control (24°C), p < 0.05.
The AbsoluteIDQ™ p180 kit used in this study for targeted metabolomics also provides custom metabolite indicators by calculating the ratio of two metabolites. The hydration of tyrosine to DOPA is the first and rate limiting step in dopamine synthesis (Neckameyer and Quinn, 1989; Vié et al., 1999). We found the ratio of DOPA/Tyr increased 5.5-fold (p = 0.029) in larvae reared at 30°C compared to 24°C. The ratio of DOPA/Tyr increased 2.5-fold in larvae reared at 28°C, although not statistically significant, it suggests that there is a trend of more dopamine synthesis at warmer temperatures. From the individual metabolites identified to have changed significantly in larvae reared at 28 and 30°C, 6 metabolites were found to be affected by both temperatures (Table 3, bold). These 6 metabolites are acylcarnitine tetradecenoylcarnitine (C14:1), biogenic amine histamine and glycerophospholipids lysoPC a C16:0, lysoPC a C16:1, lysoPC a C18:0, and lysoPC a C20:3.
Linking Metabolites to Life History Outcomes
We investigated whether metabolites significantly affected at high temperatures (the 6 metabolites that appeared in both 28 and 30°C when compared to 24°C) are associated with life history outcomes of N. triangulifer. We looked at the concentration of the 6 metabolites at both warm and cold ends (14°C; Datasheet 3) of rearing temperatures and found that histamine had a negative association with body mass (which its outcomes are directly associated with fecundity) (r = −0.96) (Figure 4A), whereas glycerophospholipid lysoPC a C20:3 had a positive association with body mass (r = 0.87) (Figure 4B). The other 4 metabolites, although significantly affected by chronic thermal challenge, did not respond linearly to temperatures spanning colder (14°C), optimal (24°C), and warmer (26 and 28°C) temperatures.
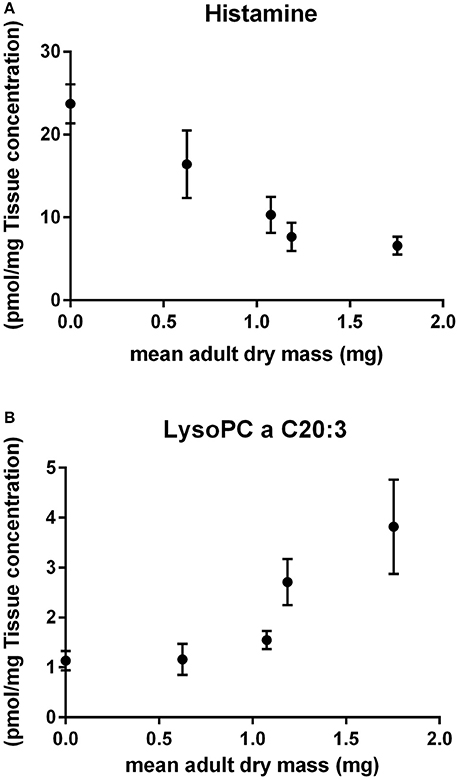
Figure 4. Association of individual metabolites and life history outcomes. (A) Histamine and mean adult dry mass (r = −0.96) and (B) lysoPC a C20:3 and mean adult dry mass (r = 0.87).
Discussion
The changing thermal regimes of freshwater ecosystems require that we better understand species responses to temperature at different time scales (Verberk et al., 2013; Sweeney et al., 2018). Recent efforts to establish thermal reaction norms (Funk et al., in preparation) and develop a growing suite of primers for functional genomic studies (Kim et al., 2017) should accelerate our understanding of thermal stress in this important taxon. In this study, we used two approaches—gene expression and targeted metabolomics to identify processes that change on the descending limb of chronic thermal performance curves.
In our gene expression studies, expression of EGL-9 was unresponsive to chronic thermal stress at both chronically stressful and lethal temperatures. This gene is strongly upregulated in response to hypoxia in this species (Kim et al., 2017), so the lack of response to chronic thermal stress suggests that hypoxia signaling is not an important process at the chronic upper thermal limits of this species. This finding is consistent with previous work showing EGL-9 to be unresponsive to short term thermal challenge at environmentally relevant temperatures (Kim et al., 2017). A slight increase in LDH expression in larvae reared at 28 and 30°C was not statistically significant relative to 22°C. An increase in LDH expression was previously observed in short-term, thermal ramp studies, but only at 34°C, a temperature that exceeds this species chronic thermal limit (Kim et al., 2017). Taken together, these results demonstrate that chronic thermal stress did not induce systemic hypoxia. We found only a modest upregulation in both thermal stress responsive genes HSP-90 and HSP-40 at 28°C, a rearing temperature considered as stressful from the poor survivorship. Therefore, it may be worthwhile to conduct full transcriptome analysis under thermal stress challenges in the future and identify HSPs that respond to thermal stress at a lower threshold. Regardless, HSP-90 expression was significantly upregulated at 30°C when compared to 22°C. The 3.3- and 1.4-fold change of HSP-90 and HSP-40 at 30°C in this study are similar to previous short term thermal challenge (with the same temperature comparison) in the same species (Kim et al., 2017). Our findings show HSP-90 as a good indicator for thermal stress studies in N. triangulifer and 30°C is a threshold for significant HSP-90 upregulation under both acute and chronic thermal challenge settings.
To understand the effect of chronic thermal stress on energy distribution, we developed new PCR probes for three genes related to energy metabolism (i.e., IR, mTOR, T6PS). IR (~2-fold, p = 0.048) and mTOR (~2.6-fold, p = 0.003) expression were both significantly upregulated at 30°C. Insulin-like proteins binding to IR activates the insulin/ insulin-like growth factor signaling pathway. This pathway interacts with various signaling pathways such as mTOR (an important nutrient sensor), and together are both involved in promoting cell growth and proliferation in insects (Zhang et al., 2000; Brogiolo et al., 2001; Neufeld, 2003; Malik et al., 2013; Vafopoulou, 2014). Prior work has demonstrated that the mTORC1 (mTOR complex 1) activity is repressed under hypoxia (Vadysirisack and Ellisen, 2012). Thus, the stimulation mTOR expression at the higher temperatures provides another line of evidence that systemic hypoxia is not occurring. Trehalose serves as a source of energy in insects as well as a compatible solute to combat environmental stresses (Asano, 2003; Shukla et al., 2014). The gene T6PS encodes the enzyme converting glucose to trehalose in insects. Although the downregulation of T6PS is not statistically significant in both 28 and 30°C, there is a suggestion that perhaps less sugar storage occurs under chronic thermal stress.
In our targeted metabolomics study the biogenic amine histamine was increased in larvae reared at both 28 and 30°C relative to larvae reared at 24°C (Tables 2, 3). Histamine is a neuro transmitter involved in several roles in insects including thermal preference behavior (Hong et al., 2006), circadian pacemaker (Arendt et al., 2017), visual and mechanosensory processing (Buchner et al., 1993; Melzig et al., 1996). Hong et al. (2006) showed impaired histamine signaling in drosophila reduces its tolerance for high temperature. Work from Morimoto et al. (2001) suggested that brain histamine involves in modulating mouse feeding behavior. Yang and colleagues (Yang et al., 2012) showed that exogenous histamine had an effect on tissue histamine accumulation and also reduced body length, body weight and development time in the crustacean Neomysis japonica Nakazawa. Although the effect of histamine on feeding behavior and growth/development in insects has not been studied, it is possible that thermally driven increases in histamine reduces food intake in mayfly larvae, resulting in smaller body size and associated reduction in fecundity (Figure 4). Interestingly, histamine was not identified in the short term thermally challenged N. triangulifer larvae (Chou et al., 2017). This suggests there may be an association between histamine and growth/development. Two other biogenic amines, dopamine and DOPA were also found to be more abundant in larvae reared at 30°C (Table 3). Like histamine, dopamine is also a neurotransmitter and plays several roles in insects such as regulating movement (Akasaka et al., 2010), arousal (Kume et al., 2005), metabolic rate, and temperature sensitivity (Ueno et al., 2012). Research shows dopamine reduces sucrose intake in cockroach Rhyparobia maderae (Allen et al., 2011). Ueno and colleagues found that fruit flies fed with a dopamine biosynthesis inhibitor had preference for a higher temperature (Ueno et al., 2012). Therefore, it is likely that dopamine acts as a cue for heat stress and reduces food intake in larvae reared at 30°C.
Additionally, we found that compared to larvae reared at 24°C, the abundance of several lysophospholipids decreased in both larvae reared at 28 and 30°C (Tables 2, 3). Sphingolipids were only found affected in larvae reared at 30°C. Although it is unclear what the specific roles of these individual lipids play in N. triangulifer, lysophopholipids, and sphingolipids generally involve in membrane composition, signal transduction, growth, and reproduction in organisms. The balance of sphingolipids has a central role in controlling the utilization of nutrients and growth in mammals (Unger, 2003; Holland and Summers, 2008). Lipids are also the main source for metabolic fuel and provides energy during extended non-feeding periods (Arrese and Soulages, 2010), such as those that occur around molting. Therefore, the decrease of lipids found in chronically thermal stressed mayfly larvae can be explained by either less lipid biosynthesis and/or increased lipid utilization as energy source for maintenance and growth. Interestingly, lysoPC a C20:3 was found to be positively associated with predicted fecundity (Figure 4). This lysophospholipid may be a key lipid for energy maintenance and growth and has potential to be explored as a biomarker in future studies. Acylcarnitines are intermediate metabolites. They are formed by carnitine accepting acyl groups from fatty acyl-CoA. Therefore, carnitine acts as a shuttle for transporting fatty acids into the mitochondria for energy production. Compared to larvae reared at 24°C, we found decreased concentration of one acylcarnitine in larvae reared at 28°C and decreased concentrations of five more acylcarnitines in larvae reared at 30°C. The results suggest that high temperature decreases fatty acid transportation efficiency for energy production. The decreased efficiency under stressful temperatures can be due to less available fatty acids or prevention of acyl groups transferring to carnitine.
Glutaminolysis is the process of glutamine conversion to glutamate. Glutamate then enters the TCA cycle as α-ketoglutarate to gain energy. Research in human cells found that glutaminolysis enhances mTORC1 signaling and is also an indication of carcinogenesis (Durán et al., 2012). Interestingly, in our results the custom metabolite indicator from the AbsoluteIDQ™ p180 kit showed an increase in glutaminolysis as well as increased ratio of glutamate to glutamine in larvae reared at 30°C (Table 3). These data indicate that larvae were using amino acids as alternative energy sources to gain energy. The indication of glutaminolysis also supports the upregulation of mTOR gene expression in Figure 2.
Taken altogether, we found a general reduction of lipids and acylcarnitines in the mayfly N. triangulifer exposed to chronic thermal stress, suggesting that larvae were energetically challenged. Thermal stress increased endogenous histamine (larvae reared at both 28 and 30°C) and dopamine (larvae reared at 30°C), which may have reduced food intake in mayfly larvae. While both thermal stress and hypoxia can cause an organism to utilize alternative energy source, gene expression and metabolomics data show no evidence of hypoxia in mayfly larvae. Temperature appears to impact (decrease) the developmental period more strongly than it increases growth rates—thus contributing to the temperature size rule. Here we show that chronic temperature challenge also forces larvae to use lipids and amino acids as alternative energy sources to support growth and maintenance costs. Further, we identified increased histamine and dopamine concentrations in larvae in response to thermal stress. It is possible that increases in histamine and dopamine also contribute to smaller body size and reduced fitness via reduced food intake. More research is needed to elucidate this potential pathway that results in restricting N. triangulifer body size and fecundity at warmer temperature.
Author Contributions
WP, JD, SS, and DJ conducted the metabolomics studies and data analyses. DF, JJ, and BS conducted the life history studies. HC conducted the gene expression studies and analyses. HC and DB conceived of the research and wrote the paper.
Funding
This work was supported by the National Science Foundation (1456191 to DB). The metabolomics analysis was funded by the National Institutes of Health Common Fund Metabolomics Program (1U24DK097193, SS, PI), and National Institute of Environmental Health Sciences (P30ES025128) to the Center for Human Health and the Environment.
Conflict of Interest Statement
The authors declare that the research was conducted in the absence of any commercial or financial relationships that could be construed as a potential conflict of interest.
Acknowledgments
Zach Acuff (RTI International, NC) is acknowledged for his work on statistical analysis using SAS.
Supplementary Material
The Supplementary Material for this article can be found online at: https://www.frontiersin.org/articles/10.3389/fevo.2018.00027/full#supplementary-material
References
Akasaka, S., Sasaki, K., Harano, K., and Nagao, T. (2010). Dopamine enhances locomotor activity for mating in male honeybees (Apis mellifera L.). J. Insect Physiol. 56, 1160–1166. doi: 10.1016/j.jinsphys.2010.03.013
Allen, J. M., Van Kummer, B. H., and Cohen, R. W. (2011). Dopamine as an anorectic neuromodulator in the cockroach Rhyparobia maderae. J. Exp. Biol. 214, 3843–3849. doi: 10.1242/jeb.062430
Angilletta, M. J. Jr. (2009). Thermal Adaptation: A Theoretical and Empirical Synthesis. Oxford: Oxford University Press, 1–304.
Angilletta, M. J., and Dunham, A. E. (2003). The temperature-size rule in ectotherms : simple evolutionary explanations may not be general. Am. Nat. 162, 334–342. doi: 10.1086/377187
Arendt, A., Baz, E., and Stengl, M. (2017). Functions of corazonin and histamine in light entrainment of the circadian pacemaker in the madeira cockroach, Rhyparobia maderae. J. Comp. Neurol. 1272, 1250–1272. doi: 10.1093/acprof:oso/9780198570875.001.1
Arrese, E. L., and Soulages, J. L. (2010). Insect fat body: energy, metabolism and regulation. Annu. Rev. Entomol. 473, 376–379. doi: 10.1146/annurev-ento-112408-085356
Asano, N. (2003). Glycosidase inhibitors: update and perspectives on practical use. Glycobiology 10, 93–104. doi: 10.1093/glycob/cwg09
Atkinson, D. (1994). Temperature and organism size: a biological law for ectortherms? Adv. Ecol. Res. 25, 1–58. doi: 10.1016/S0065-2504(08)60212-3
Atkinson, D., and Sibly, R. M. (1997). Why are organisms usually bigger in colder environments? Making sense of a life history puzzle. Trends Ecol. Evol. 12, 234–239. doi: 10.1016/S0169-5347(97)01058-6
Bonada, N., Prat, N., Resh, V. H., and Statzner, B. (2006). Developments in aquatic insect biomonitoring: a comparative analysis of recent approaches. Annu. Rev. Entomol. 51, 495–523. doi: 10.1146/annurev.ento.51.110104.151124
Brogiolo, W., Stocker, H., Ikeya, T., Rintelen, F., Fernandez, R., and Hafen, E. (2001). An evolutionarily conserved function of the Drosophila insulin receptor and insulin-like peptides in growth control. Curr. Biol. 11, 213–221. doi: 10.1016/S0960-9822(01)00068-9
Buchner, E., Buchner, S., Burg, M. G., Hobauer, A., Pak, W. L., and Pollack, I. (1993). Histamine is a major mechanosensory neurotransmitter candidate in Drosophila melanogaster. Cell Tissue Res. 273, 119–125.
Cairns, J. Jr., and Pratt, J. R. (1993). “A history of biological monitoring using benthic macroinvertebrates,” in Freshwater Biomonitoring and Benthic Macroinvertebrates, eds D. M. Rosenberg and V. H. Resh (New York, NY: Chapman and Hall), 10–27.
Chou, H., Pathmasiri, W., Deese-spruill, J., Sumner, S., and Buchwalter, D. B. (2017). Metabolomics reveal physiological changes in mayfly larvae (Neocloeon triangulifer) at ecological upper thermal limits. J. Insect Physiol. 101, 107–112. doi: 10.1016/j.jinsphys.2017.07.008
Conley, J. M., Funk, D. H., and Buchwalter, D. B. (2009). Selenium bioaccumulation and maternal transfer in the mayfly Centroptilum triangulifer in a life-cycle, periphyton-biofilm trophic assay. Environ. Sci. Technol. 43, 7952–7957. doi: 10.1021/es9016377
Conley, J. M., Funk, D. H., Cariello, N. J., and Buchwalter, D. B. (2011). Food rationing affects dietary selenium bioaccumulation and life cycle performance in the mayfly Centroptilum triangulifer. Ecotoxicology 20, 1840–1851. doi: 10.1007/s10646-011-0722-1
Conley, J. M., Funk, D. H., Hesterberg, D. H., Hsu, L. C., Kan, J., Liu, Y. T., et al. (2013). Bioconcentration and biotransformation of selenite versus selenate exposed periphyton and subsequent toxicity to the mayfly Centroptilum triangulifer. Environ. Sci. Technol. 47, 7965–7973. doi: 10.1021/es400643x
Conley, J. M., Watson, A. T., Xie, L., and Buchwalter, D. B. (2014). Dynamic selenium assimilation, distribution, efflux, and maternal transfer in Japanese medaka fed a diet of se-enriched mayflies. Environ. Sci. Technol. 48, 2971–2978. doi: 10.1021/es404933t
Dillon, M. E., and Frazier, M. R. (2013). Thermodynamics constrains allometric scaling of optimal development time in insects. PLoS ONE 8:e84308. doi: 10.1371/journal.pone.0084308
Durán, R. V., Oppliger, W., Robitaille, A. M., Heiserich, L., Skendaj, R., Gottlieb, E., and Hall, M. N. (2012). Article glutaminolysis activates Rag-mTORC1 signaling. Mol. Cell. 47, 349–358. doi: 10.1016/j.molcel.2012.05.043
Funk, D. H., Jackson, J. K., and Sweeney, B. W. (2006). Taxonomy and genetics of the parthenogenetic mayfly Centroptilum triangulifer and its sexual sister Centroptilum alamance (Ephemeroptera:Baetidae). J. North Am. Benthol. Soc. 25, 417–429. doi: 10.1899/0887-3593(2006)25[417:TAGOTP]2.0.CO;2
Holland, W. L., and Summers, S. A. (2008). Sphingolipids, insulin resistance, and metabolic disease: new insights from in vivo manipulation of sphingolipid metabolism. Endocr. Rev. 29, 381–402. doi: 10.1210/er.2007-0025
Hong, S. T., Bang, S., Paik, D., Kang, J., Hwang, S., Jeon, K., et al. (2006). Histamine and its receptors modulate temperature-preference behaviors in Drosophila. J. Neurosci. 26, 7245–7256. doi: 10.1523/JNEUROSCI.5426-05.2006
Hynes, H. B. N. (1970). The ecology of stream insects. Ann. Rev. Entomol. 15, 25–42. doi: 10.1146/annurev.en.15.010170.000325
Johnson, B. R., Weaver, P. C., Nietch, C. T., Lazorchak, J. M., Struewing, K. A., and Funk, D. H. (2015). Elevated major ion concentrations inhibit larval mayfly growth and development. Environ. Toxicol. Chem. 34, 167–172. doi: 10.1002/etc.2777
Johnson, R. K., Wiederholm, T., and Rosenberg, D. M. (1993). “Freshwatr biomonitoring using individual organisms, populations, and species assemblages of benthic macroinvertebrates,” in Freshwater Biomonitoring and Benthic Macroinvertebrates, eds D. M. Rosenberg and V. H., Resh (New York, NY: Chapman & Hall), 40–158.
Kim, K. S., Funk, D. H., and Buchwalter, D. B. (2012). Dietary (periphyton) and aqueous Zn bioaccumulation dynamics in the mayfly Centroptilum triangulifer. Ecotoxicology 21, 2288–2296. doi: 10.1007/s10646-012-0985-1
Kim, K. S., Chou, H., Funk, D. H., Jackson, J. K., Sweeney, B. W., and Buchwalter, D. B. (2017). Physiological responses to short term thermal challenge in mayfly larvae (Neocloeon triangulifer) in relation to upper thermal limits. J. Exp. Biol. 220, 2598–2605 doi: 10.1242/jeb.156919
Kingsolver, J. G., and Huey, R. B. (2008). Size, temperature, and fitness: three rules. Evol. Ecol. Res. 10, 251–268.
Klok, C. J., and Harrison, J. F. (2013). The temperature size rule in arthropods: independent of macro-environmental variables but size dependent. Integr. Comp. Biol. 53, 557–570. doi: 10.1093/icb/ict075
Klok, C. J., Sinclair, B. J., and Chown, S. L. (2004). Upper thermal tolerance and oxygen limitation in terrestrial arthropods. J. Exp. Biol. 207, 2361–2370. doi: 10.1242/jeb.01023
Kume, K., Kume, S., Park, S. K., Hirsh, J., and Jackson, F. R. (2005). Dopamine is a regulator of arousal in the fruit fly. J. Neurosci. 25, 7377–7384 doi: 10.1523/JNEUROSCI.2048-05.2005
Kunz, J. L., Conley, J. M., Buchwalter, D. B., Norberg-King, T. J., Kemble, N. E., Wang, N., et al. (2013). Use of reconstituted waters to evaluate effects of elevated major ions associated with mountaintop coal mining on freshwater invertebrates. Environ. Toxicol. Chem. 32, 2826–2835. doi: 10.1002/etc.2391
Lopez, A. R., Hesterberg, D. R., Funk, D. H., and Buchwalter, D. B. (2016). Bioaccumulation dynamics of arsenate at the base of aquatic food webs. Environ. Sci. Technol. 50, 6556–6564. doi: 10.1021/acs.est.6b01453
Malik, A. R., Urbanska, M., Macias, M., Skalecka, A., and Jaworski, J. (2013). Beyond control of protein translation : what we have learned about the non-canonical regulation and function of mammalian target of rapamycin (mTOR). Biochim. Biophys. Acta 1834, 1434–1448. doi: 10.1016/j.bbapap.2012.12.010
Mccauley, S. J., Hammond, J. I., Frances, D. N., and Mabry, K. E. (2015). Effects of experimental warming on survival, phenology, and morphology of an aquatic insect (Odonata). Ecol. Entomol. 40, 211–220. doi: 10.1111/een.12175
McCue, M. D., and De Los Santos, R. (2013). Upper thermal limits of insects are not the result of insufficient oxygen delivery. Physiol. Biochem. Zool. 86, 257–265. doi: 10.1086/669932
Melzig, J., Buchner, S., Wiebel, F., Wolf, R., Burg, M., Pak, W. L., et al. (1996). Genetic depletion of histamine from the nervous system of Drosophila eliminates specific visual and mechanosensory behavior. J. Comp. Physiol. A 179, 763–773.
Morimoto, T., Yamamoto, Y., and Yamatodani, A. (2001). Brain histamine and feeding behavior. Behav. Brain Res. 124, 145–150. doi: 10.1016/S0166-4328(01)00225-X
Neckameyer, W. S., and Quinn, W. G. (1989). Isolation and characterization of the gene for drosophila tyrosine hydroxylase. Neuron 2, 1167–1175. doi: 10.1016/0896-6273(89)90183-9
Neufeld, T. P. (2003). Body building : regulation of shape and size by PI3K / TOR signaling during development. Mech. Dev. 120, 1283–1296 doi: 10.1016/j.mod.2003.07.003
Newbold, J. D., Sweeney, B. W., and Vannote, R. L. (1994). A model for seasonal synchrony in stream mayflies. J. North Am. Benthol. Soc. 13, 3–18. doi: 10.2307/1467261
Pfaffl, M. W. (2001). A new mathematical model for relative quantification in real-time RT-PCR. Nucleic Acids Res. 29:e45. doi: 10.1093/nar/29.9.e45
Pörtner, H. O. (2001). Climate change and temperature-dependent biogeography: oxygen limitation and thermal tolerance in animals. Naturwissenschaften 88, 137–146. doi: 10.1007/s001140100216
Pörtner, H. O. (2002). Climate variations and the physiological basis of temperature dependent biogeography: systemic to molecular hierarchy of thermal tolerance in animals. Comp. Biochem. Physiol. 132A, 739–761. doi: 10.1016/S1095-6433(02)00045-4
Pörtner, H. O. (2010). Oxygen- and capacity-limitation of thermal tolerance: a matrix for integrating climate-related stressor effects in marine ecosystems. J. Exp. Biol. 213, 881–893. doi: 10.1242/jeb.037523
Pörtner, H. O., Bennett, A. F., Bozinovic, F., Clarke, A., Lardies, M. A., Lucassen, M., et al. (2006). Trade-offs in thermal adaptation: the need for a molecular to ecological integration. Physiol. Biochem. Zool. 79, 295–313. doi: 10.1086/499986
Rosenberg, D. M., and Resh, V. H. (1993). Freshwater Biomonitoring and Benthic Macroinvertebrates. New York, NY: Chapman and Hall.
Rosenberg, D. M., Resh, V. H., and King, R. S. (2008). “Use of aquatic insects in biomonitoring,” in An Introduction to the Aquatic Insects of North America, eds R. W. Merritt, K. W. Cummins, and M. B. Berg (Dubuque: Kendall/Hunt Publishing Company), 123–138.
Schulte, P. M., Healy, T. M., and Fangue, N. A. (2011). Thermal performance curves, phenotypic plasticity, and the time scales of temperature exposure. Integr. Comp. Biol. 51, 691–702. doi: 10.1093/icb/icr097
Shukla, E., Thorat, L. J., Nath, B. B., and Gaikwad, S. M. (2014). Insect trehalase: physiological significance and potential applications. Glycobiology 25, 357–367. doi: 10.1093/glycob/cwu125
Snart, C. J., Hardy, I. C., and Barrett, D. A. (2015). Entometabolomics : applications of modern analytical techniques to insect studies. Entomol. Exp. Appl. 155, 1–17. doi: 10.1111/eea.12281
Soucek, D. J., and Dickinson, A. (2015). Full-life chronic toxicity of sodium salts to the mayfly Neocloeon triangulifer in tests with laboratory cultured food. Environ. Toxicol. Chem. 34, 2126–2137. doi: 10.1002/etc.3038
Stevens, M. M., Jackson, S., Bester, S. A., Terblanche, J. S., and Chown, S. L. (2010). Oxygen limitation and thermal tolerance in two terrestrial arthropod species. J. Exp. Biol. 213, 2209–2218. doi: 10.1242/jeb.040170
Struewing, K. A., Lazorchak, J. M., Weaver, P. C., Johnson, B. R., Funk, D. H., and Buchwalter, D. B. (2015). Chemosphere Part 2 : sensitivity comparisons of the mayfly Centroptilum triangulifer to Ceriodaphnia dubia and Daphnia magna using standard reference. Chemosphere 139, 597–603. doi: 10.1016/j.chemosphere.2014.04.096
Sweeney, B. W., Funk, D. H., Jackson, J. K., Camp, A. A., and Buchwalter, D. B. (2018). Why adult mayflies of Cloeon dipterum (Ephemeroptera: Baetidae) become smaller as temperature warms. Freshw. Sci. 37, 64–81. doi: 10.1086/696611
Sweeney, B. W., Jackson, J. K., Newbold, J. D., and Funk, D. H. (1990). “Climate change and the life histories and biogeography of aquatic insects in Eastern North America,” in Global Cilmate Change and Freshwater Ecosystems, eds P. Firth and S. Fisher (New York, NY: Springer-Verlag), 143–176.
Sweeney, B. W., and Vannote, R. L. (1978). Size variation and the distribution of hemimetabolous aquatic insects: two thermal equilibrium hypotheses. Science 200, 444–446. doi: 10.1126/science.200.4340.444
Sweeney, B. W., and Vannote, R. L. (1984). Influence of food quality and temperature on life- history characteristics of the parthenogenetic mayfly, Cloeon triangulifer. Freshw. Biol. 14, 621–630. doi: 10.1111/j.1365-2427.1984.tb00181.x
Ueno, T., Tomita, J., Kume, S., and Kume, K. (2012). Dopamine modulates metabolic rate and temperature sensitivity in Drosophila melanogaster. PLoS ONE 7:e31513. doi: 10.1371/journal.pone.0031513
Unger, R. H. (2003). Minireview: weapons of lean body mass destruction: the role of ectopic lipids in the metabolic syndrome. Endocrinology 144, 5159–5165 doi: 10.1210/en.2003-0870
Vadysirisack, D. D., and Ellisen, L. W. (2012). mTOR activity under hypoxia. Methods Mol. Biol. 821, 45–58. doi: 10.1007/978-1-61779-430-8_4
Vafopoulou, X. (2014). The coming of age of insulin-signaling in insects. Front. Physiol. 5:216. doi: 10.3389/fphys.2014.00216
Vannote, R. L., and Sweeney, B. W. (1980). Geographical analysis of thermal equilibeia: a conceptual model for evaluating the effect of natural and modified thermal regimes on aquatic insect communities. Am. Nat. 115, 667–695. doi: 10.1086/283591
Verberk, W. C., and Bilton, D. T. (2011). Can oxygen set thermal limits in an insect and drive gigantism? PLoS ONE 6:e22610. doi: 10.1371/journal.pone.0022610
Verberk, W. C., and Bilton, D. T. (2013). Respiratory control in aquatic insects dictates their vulnerability to global warming. Biol. Lett. 9:20130473. doi: 10.1098/rsbl.2013.0473
Verberk, W. C., and Bilton, D. T. (2015). Oxygen-limited thermal tolerance is seen in a plastron-breathing insect and can be induced in a bimodal gas exchanger. J. Exp. Biol. 218, 2083–2088. doi: 10.1242/jeb.119560
Verberk, W. C. E. P., Sommer, U., Davidson, R. L., and Viant, M. R. (2013). Integrative and comparative biology anaerobic metabolism at thermal extremes : a metabolomic test of the oxygen limitation hypothesis in an aquatic insect. Integr. Comp. Biol. 53, 609–619. doi: 10.1093/icb/ict015
Verberk, W. C., Overgaard, J., Ern, R., Bayley, M., Wang, T., Boardman, L., et al. (2016). Does oxygen limit thermal tolerance in arthropods? A critical review of current evidence. Comp. Biochem. Physiol. A Mol. Integr. Physiol. 192, 64–78. doi: 10.1016/j.cbpa.2015.10.020
Vié, A., Cigna, M., Toci, R., and Birman, S. (1999). Differential regulation of drosophila tyrosine hydroxylase isoforms by dopamine binding and cAMP-dependent phosphorylation. J. Biol. Chem. 274, 16788–16795. doi: 10.1074/jbc.274.24.16788
Ward, J. V., and Stanford, J. A. (1982). Thermal responses in the evolutionary ecology of aquatic insects. Ann. Rev. Entomol. 27, 97–117. doi: 10.1146/annurev.en.27.010182.000525
Weaver, P. C., Lazorchak, J. M., Struewing, K. A., Decelles, S. J., Funk, D. H., Buchwalter, D. B., et al. (2015). Chemosphere Part 1 : laboratory culture of Centroptilum triangulifer (Ephemeroptera : Baetidae) using a defined diet of three diatoms. Chemosphere 139, 589–596. doi: 10.1016/j.chemosphere.2014.04.092
Wesner, J. S., Kraus, J. M., Schmidt, T. S., Walters, D. M., and Clements, W. H. (2014). Metamorphosis enhances the effects of metal exposure on the mayfly, Centroptilum triangulifer. Environ. Sci. Technol. 48, 10415–10422. doi: 10.1021/es501914y
Yang, X., Wang, J., Zhao, L., Fan, P., Wu, X., Cheng, Y., et al. (2012). Effects of elevated ambient histamine level on survival, growth, sexual maturity and tissue histamine accumulation of the mysis Neomysis awatschensis and Neomysis japonica Nakazawa. Aquacult. Int. 20, 347–356. doi: 10.1007/s10499-011-9463-7
Keywords: aquatic insects, mayfly, thermal limits, metabolomics, temperature
Citation: Chou H, Pathmasiri W, Deese-spruill J, Sumner SJ, Jima DD, Funk DH, Jackson JK, Sweeney BW and Buchwalter DB (2018) The Good, the Bad, and the Lethal: Gene Expression and Metabolomics Reveal Physiological Mechanisms Underlying Chronic Thermal Effects in Mayfly Larvae (Neocloeon triangulifer). Front. Ecol. Evol. 6:27. doi: 10.3389/fevo.2018.00027
Received: 24 January 2018; Accepted: 06 March 2018;
Published: 23 March 2018.
Edited by:
Adalbert Balog, Sapientia Hungarian University of Transylvania, RomaniaReviewed by:
Domokos Erzsébet, University of Medicine and Pharmacy of Târgu Mureş, RomaniaCaroline Habold, UMR7178 Institut Pluridisciplinaire Hubert Curien (IPHC), France
Copyright © 2018 Chou, Pathmasiri, Deese-spruill, Sumner, Jima, Funk, Jackson, Sweeney and Buchwalter. This is an open-access article distributed under the terms of the Creative Commons Attribution License (CC BY). The use, distribution or reproduction in other forums is permitted, provided the original author(s) and the copyright owner are credited and that the original publication in this journal is cited, in accordance with accepted academic practice. No use, distribution or reproduction is permitted which does not comply with these terms.
*Correspondence: Hsuan Chou, hchou2@ncsu.edu
Susan J. Sumner, susan_sumner@unc.edu