- Laboratory of Invertebrate Immunological Defense and Reproductive Biology, School of Life Sciences, East China Normal University, Shanghai, China
The Down syndrome cell adhesion molecule (Dscam) gene is an extraordinary example of diversity that can produce thousands of isoforms and has so far been found only in insects and crustaceans. Cumulative evidence indicates that Dscam may contribute to the mechanistic foundations of specific immune responses in insects. However, the mechanism and functions of Dscam in relation to pathogens and immunity remain largely unknown. In this study, we identified the genome organization and alternative Dscam exons from Chinese mitten crab, Eriocheir sinensis. These variants, designated EsDscam, potentially produce 30,600 isoforms due to three alternatively spliced immunoglobulin (Ig) domains and a transmembrane domain. EsDscam was significantly upregulated after bacterial challenge at both mRNA and protein levels. Moreover, bacterial specific EsDscam isoforms were found to bind specifically with the original bacteria to facilitate efficient clearance. Furthermore, bacteria-specific binding of soluble EsDscam via the complete Ig1–Ig4 domain significantly enhanced elimination of the original bacteria via phagocytosis by hemocytes; this function was abolished by partial Ig1–Ig4 domain truncation. Further studies showed that knockdown of membrane-bound EsDscam inhibited the ability of EsDscam with the same extracellular region to promote bacterial phagocytosis. Immunocytochemistry indicated colocalization of the soluble and membrane-bound forms of EsDscam at the hemocyte surface. Far-Western and coimmunoprecipitation assays demonstrated homotypic interactions between EsDscam isoforms. This study provides insights into a mechanism by which soluble Dscam regulates hemocyte phagocytosis via bacteria-specific binding and specific interactions with membrane-bound Dscam as a phagocytic receptor.
Introduction
Although the specializations of the immune system that define adaptive immunity were believed to be unique to gnathostomes (jawed vertebrates) (1, 2), a possible alternative adaptive immune system has recently been identified in lamprey (3) and arthropods (4, 5). It has been reported that highly specific immune protection to certain pathogens can be transferred from mother to offspring in crustacean and insects (6–10), and specific life-long immunological memory can be sustained, providing protection against the pathogen responsible for the original infection (11). Thus, accumulating evidence suggests that the arthropod immune system has greater complexity than previously appreciated.
The vertebrate immune system discriminates between a plethora of microbes through a diverse repertoire of pattern-recognition receptors generated by V(D)J recombination and somatic hyper-mutation of the antibody immunoglobulin (Ig) domains. By contrast, although arthropods do not produce antibodies, approximately 150 Ig domain protein-encoding genes have been identified in some insect genomes (12–14). Most of these genes have been studied with regard to neuronal guidance, although the implications for immunity have been investigated more recently (12–14). The Down syndrome cell adhesion molecule gene, Dscam, which is a member of the arthropod Ig superfamily, can potentially produce 38,016 different forms through alternative splicing of exons in Drosophila melanogaster (14). Moreover, membrane-bound and soluble forms of Dscam (mDscam and sDscam), which are distinguished only by the presence or absence of transmembrane and cytoplasmic tail regions, have also been identified in hemocyte and cell-free hemolymph (13), respectively. Therefore, it is conceivable that a large protein isoform repertoire with the potential for recognizing diverse ligands and epitopes could be generated in crustaceans and insects.
Limited research on the Dscam-mediated immune responses has been published (13, 15–20), although these studies have provided pioneering insights into the specific arthropod immune responses. Studies in mosquito demonstrated that alternative splicing of Dscam can generate pathogen-specific isoforms in response to immune challenge (16). Moreover, Dscam knockdown was shown to impair the capacity of hemocytes to phagocytose bacteria in D. melanogaster, while Dscam in hemocytes of D. melanogaster (13) and Anopholes gambiae (16) bind Escherichia coli and potentially acts as both a phagocytic receptor and subsequently as an opsonin. The crystal structures of the two Dscam isoforms revealed two distinct surface epitopes (epitope I and epitope II) that are composed of hypervariable amino-acid residues and conserved residues, allowing a given Dscam isoform to form a homodimer and retain the ability to potentially recognize, opsonize, and crosslink pathogens (21, 22). Epitope I sequences is the N-terminal segments of both exon 4 and exon 6, which engaged in homophilic binding specificity, whereas epitope II is the C-terminal segments of both exon 4 and exon 6, which was hypothesized to bind to non-Dscam ligands, e.g., pathogens, due to the apparently faster-evolving sequence variability that would be consistent with immune receptor adaptations to dynamic alterations in host–pathogen interactions (21). These findings provide exciting evidence that Dscam may mediate distinct functions in the innate immune system of arthropods via the preferential use of splice variants post-infection and also highlight the potential importance for epitope I and II in Dscam-mediated immune reactions.
Chinese mitten crab, Eriocheir sinensis, is one of the most important economic aquaculture crustacean species in southeast Asia. In recent years, the frequent outbreaks of diseases have given rise to decreased production and enormous economic loss. Most of these diseases in E. sinensis are usually caused by different bacteria, for this reason, the study of the pattern-recognition receptors would help to reveal the pathogenesis and expand the knowledge on arthropods’ Dscam functions. In this study, we raised the hypothesis that pathogen-induced particular soluble Dscam isoforms specific binding with the original bacteria via epitope II, and then interact with membrane-bound Dscam that share the same extracellular regions via epitope I to promote phagocytosis. To test that hypothesis, the genome structure of Eriocheir sinensis Dscam (EsDscam) was revealed and every alternative spliced exon was confirmed. After that, the mRNA and protein expression level post bacteria stimulation were analyzed, the candidate alternatively spliced exons that may contribute to bacteria-specific binding were captured by testing the bacteria-binding activity of epitope II, and confirmed by test recombinant protein mediated bacteria-specific binding activity in vitro and bacteria clearance in vivo. Furthermore, soluble EsDscam regulated hemocyte phagocytosis and the role of specific membrane-bound EsDscam in this process were tested. This study provides novel data testing the role of epitope II in bacteria-specific binding, and epitope I-mediated interaction between membrane-bound and soluble Dscam. To the best of our knowledge, these are important and as-of-yet unanswered questions in Dscam research, and this study will benefit for better understanding the previous unassured alternatively spliced genes mediated sophisticated immune functions in arthropods.
Materials and Methods
Animals
All animal experiments were performed according to the protocol approved by the East China Normal University Animal Care and Use Committee (Protocol license number: AR2012/12017) and in direct accordance with Ministry of Science and Technology of the People’s Republic of China on animal care guidelines. Healthy crabs (100 ± 20 g each, adult, non-antibiotic, or antifungal feed ground) were obtained from a local aquatic farm in Shanghai, China. After transfer to the laboratory, the crabs were maintained in filtered and aerated freshwater with abundance of oxygen, and fed with a commercial formulated diet (KangDa NO.183 diets for the adult crabs, Huai’an KangDa Feed Co., Ltd., Huai’an City, Jiangsu Province, China) daily which contains no antibiotics. Crabs were acclimated for a week at 22°C before use and 5% was randomly detected by PCR using TaKaRa 16S rDNA Bacterial Identification PCR Kit to ensure that the crab were free of Staphylococcus aureus and Vibrio parahemolyticus.
Genomic Sequencing, Assembly, and Annotation
Multiple paired-end and mate-pair libraries were constructed, with a spanning size range of 180 bp to 20 kb. All libraries were sequenced on the Illumina HiSeq 2000 and PacBio platforms. After filtering out the adaptor sequences, low quality reads and duplicate reads, a total of 439.3 Gb of data were retained for assembly. The draft assembly was evaluated using transcriptome data and comparing the distributions of GC content across the whole genomes of primates, as well as by mapping the high-quality reads from paired-end libraries with short insert sizes to the scaffolds using the Burrows-Wheeler Aligner (unpublished). Subsequently, the EsDscam gene sequence was captured from E. sinensis genomic data by alignment and searching the EsDscam cDNA sequence. The preliminary EsDscam gene was improved by PCR to avoid sequencing errors and gaps.
Analysis of Epitope I and Epitope II
The amino-terminal halves of Ig2 and Ig3 were encoded by variable alternative exons represented by DNA stretches of approximately 195 bp for Ig2 (exon 4) and 169 bp for Ig3 (exon 6) in E. sinensis. Based on the structural characteristics in D. melanogaster (21) and the similarities in the secondary structure of Dscam among D. melanogaster, D. magna (23), and E. sinensis, the epitope I and epitope II sequences of EsDscam were identified using PSIPRED1 (24). Both exon 4 and exon 6 in EsDscam contribute to each epitope in such a manner that their N-terminal segments encode epitope I and the C-terminal segments encode epitope II. In exon cluster 4, the 12 amino acids between the conserved 7Q and the 18V were considered to belong to epitope I, and the sequence of approximately 18 amino acids after 43W were considered to belong to epitope II (23). In exon cluster 6, the eight amino acids after 15R were considered to belong to epitope I, and the eight amino acids before the conserved LLC motif were considered to belong to epitope II (23) (Figure S3 in Supplementary Material). For analysis of the conservation of different epitope sequences, the identified epitope I and epitope II sequence logos of exon 4 and exon 6 were generated using WebLogo.2
Hemocyte Culture
Primary culture of E. sinensis hemocyte was performed according to established techniques (25). Briefly, hemolymph was collected from the non-sclerotized membrane of the posterior walking leg using a 10-ml sterile syringe preloaded with 5-ml pre-cooled sterile anticoagulant (0.14 M NaCl; 0.1 M glucose; 30 mM trisodium citrate; 26 mM citric acid; 10 mM EDTA, pH 4.6) at a ratio of 1:1 (25). The collected hemolymph was immediately centrifuged at 300 × g for 10 min at 4°C, and then the serum was removed and washed with PBS. The isolated hemocytes were gently resuspended in Leibovitz’s L-15 medium (Sigma-Aldrich, USA) supplemented with 1% antibiotics [10,000 U/ml penicillin, 10,000 µg/ml streptomycin (Gibco, USA)], 0.2 mM NaCl (676 ± 5.22 mOsm/kg), pH 7.20–7.40, and subsequently were counted using an automated cell counter (Invitrogen Countess) before seeding 4 ml (1 × 105 cells/ml) in 60-mm dishes (Corning Inc., USA).
Bacterial Challenge and Sample Collection
Bacterial strains [V. parahemolyticus (BYK00036), S. aureus (BYK0113), Bacillus subtilis (BYK0123), and Aeromonas hydrophila (BYK00335)], which are main pathogenic bacteria that caused heavy diseases in aquaculture (26), were gifted by the National Pathogen Collection Center for Aquatic Animals (Shanghai Ocean University, Shanghai, China). Bacteria were cultured overnight in LB medium and collected by centrifugation at 5,000 × g for 5 min, then washed three times, and resuspended in sterile PBS. The colony-forming unit (CFU) counts of bacteria were determined by plating the diluted suspension onto agar plates.
For in vitro bacterial stimulation, experiments were performed according to previously reported methods (25). Briefly, overnight cultures of bacteria were heat inactivated at 72°C for 20 min and collected by centrifugation. Heat-inactivated V. parahemolyticus, S. aureus, B. subtilis, and A. hydrophila (1 × 108 microbes per milliliter, 50 µl) were added separately to one 60-mm dish (Corning) with about 4 × 105 of hemocytes. Sterile PBS (50 µl) was used as the control. After 0, 2, 4, 6, 12, 24, 36, and 48 h of bacterial stimulation, hemocytes and hemocyte culture medium were both collected and stored at −80°C. The collected hemocytes samples were used to extract the total RNA for EsDscam mRNA expression analyses, the hemocyte culture medium samples were used for EsDscam protein expression analyses. Three independent repeats were performed with at least three crab for each sample.
For in vivo bacterial infection, S. aureus and V. parahemolyticus were both cultured overnight in LB broth at 37°C and adjusted to 1 × 108 CFU per milliliter, after that, mixed pathogen (2 × 107 CFU, 200 µl) were injected into hemolymph from the non-sclerotized membrane of the posterior walking leg, then different tissues that including intestine, heart, brain, hepatopancreas, gill, testis, stomach, cell-free hemolymph, and hemocytes were collected at 24 h post-challenge and stored at −80°C. The collected samples were used to extract the total protein for EsDscam protein expression analyses, three independent repeats were performed with three crab for each sample.
Recombinant Expression, Purification, and Antiserum Production
To produce antiserum against EsDscam, a fusion protein was expressed in E. coli according to a previously reported method (27). A PCR fragment representing the extracellular domains of EsDscam from FNIII3 to FNIII6 was amplified using specific primers (EsDscam-anti-F and EsDscam-anti-R; Table 1). Subsequently, the PCR products were purified and digested with restriction enzymes (EcoRI and XhoI) for ligation of the final DNA fragment into the pET-28a (+) vector (Novagen, USA). The recombinant plasmid pET-28a (+)-EsDscam was transformed into E. coli Rosetta (DE3) cells (Transgen, China) for expression of the recombinant EsDscam protein. Fusion protein expression was induced by exposure to four different sets of conditions: 1 mM isopropyl-β-d-thiogalactoside (IPTG) for 3 h at 37°C; 0.25 mM IPTG for 3 h at 37°C; 1 mM IPTG for 3 h at 30°C; and 0.25 mM IPTG for 3 h at 30°C. The fusion protein was purified with Ni-NTA resin (Transgen, China) according to the manufacturer’s instructions. Subsequently, rabbit antiserum against EsDscam was prepared according to a standard procedure (28), and the antiserum titer was determined by ELISA. Three recombinant proteins [rEsDscam(4.6,6.9), rEsDscam(4.24,6.19), and rEsDscam(4.12,6.20)] containing Ig1–Ig4 and another three proteins containing Ig1–Ig2, Ig2–Ig3, and Ig3–Ig4 were also expressed in E. coli with the pET-28a (+) vector after induction with IPTG as described above. The specific primers are listed in Table 1. Subsequently, rabbit antisera against rEsDscam(4.24,6.19) and rEsDscam(4.12,6.20) were prepared using the same method for detection of the specific protein isoforms expressed by the bacteria. Furthermore, to confirm the interaction of Es-sDscam and Es-mDscam by far-Western assay, the myc-tag recombinant proteins [rEsDscam(4.24,6.19) and rEsDscam(4.12,6.20)] were expressed in E. coli with the pBAD-myc-His vector after induction with l-arabinose. For use in vivo, these proteins received an additional wash with excess 0.1% Triton X-114 at 4°C before the final elution was performed to remove contaminating endotoxins according to a previously reported method (29). The endotoxin content of the proteins was detected using a lipopolysaccharide (LPS) detection kit (GenScript, China) according to the manufacturer’s instructions.
Expression Profiles of EsDscam
The total RNA from bacteria-stimulated hemocytes was extracted using TRIzol Reagent (Invitrogen) according to the manufacturer’s instructions. Total RNA (1 µg) was then reverse transcribed using the PrimeScript™ RT reagent kit with gDNA Eraser (Perfect Real-Time) (TaKaRa, Japan) and the synthesized first-strand cDNA was used as a template for RT-qPCR. RT-qPCR was performed using SYBR Premix Ex Taq (Tli RNaseH Plus) (TaKaRa) and the CFX96™ Real-Time System (BioRad, USA). The EsDscam-RT-F and EsDscam-RT-R primers (Table 1) were used to analyze the expression patterns of the EsDscam gene in hemocytes after 0, 2, 4, 6, 12, 24, 36, and 48 h of bacterial stimulation. β-actin gene was used as the internal reference. All RT-qPCRs were completed in triplicate and normalized to the control gene. The PCR conditions were 95°C for 30 s, followed by 40 cycles of 95°C for 5 s and 66°C for 30 s. The melting curves from 60 to 95°C were then determined. EsDscam expression levels were calculated using the 2−ΔΔCT (ΔΔCT = ΔCTEsDscam-ΔCTβ-actin) method (30). Three independent experiments were performed, and the results present the mean ± SD.
The EsDscam protein expression profiles after bacterial challenge in vivo and in vitro were both determined by Western blotting. Briefly, for detecting total EsDscam protein expression profiles, the protein samples from different tissues were extracted using the Minute™ series of protein extraction kits (Invent, USA) and separated by 6% SDS-PAGE, the non-stimulation crabs’ protein samples were also extracted and analyzed by Western blotting. Proteins (50 µg) were then transferred to a PVDF membrane, and blocked with 5% non-fat milk in PBST for 1 h at room temperature. After blocking, the membrane was incubated with primary antiserum against EsDscam (1:1,000) overnight at 4°C, followed by incubation with goat anti-rat IgG H&L (Alexa Fluor® 790) (1:2000; Abcam, UK). After several washing steps with PBST, the target band was visualized using the eECL Western Blot kit (Cwbiotech, China) and imaged with ChemiDoc XRS (BioRad, USA). The expression of β-actin (Transgen, China) was detected as the reference. To detect the soluble EsDscam expression profiles, the hemocyte culture medium (10 µl) was separated by 6% SDS-PAGE, and analyzed by Western blotting as described above.
Exon 4 and 6 Variants in EsDscam
To detect the alternatively spliced exon 4 and exon 6 isoforms of EsDscam after bacterial stimulation, total RNA was extracted from the hemocytes at a specific time point post-stimulation with S. aureus (6 h), B. subtilis (48 h), A. hydrophila (12 h), and V. parahemolyticus (6 h); a non-bacteria-stimulated (0 h) group served as the control. According to previously reported methods (18, 27, 31), the hemocyte from three independent culture dishes were used to prepare each sample, and the exon 4 and exon 6 isoforms of EsDscam were amplified by RT-PCR using the EsDscam exon4-F/R and EsDscam exon6-F/R primers for TA cloning. Individual colonies (n = 80) containing the exon 4 and exon 6 fragments, respectively, were selected randomly from each agar plate and sequenced using the universal primer. The sequencing results for the clones from the five different groups were aligned and analyzed using ClustalX 2.0. The observed number of exon 4 and exon 6 isoforms in each group was calculated by Excel. Two independent experiments were performed, and the results represent the mean colonies.
Peptide Synthesis
Based on the results of exon variants of EsDscam detected after bacterial stimulation as well as the conservation sites of epitope II sequences, 11 peptides (10 representing epitope II of exon 4 and exon 6 and 1 from the non-alternative splicing region of EsDscam gene representing a conserved motif as a control) were synthesized by GL Biochem (Shanghai, China) and dissolved in ddH2O at a final concentration of 50 µg/ml.
Bacteria-Binding Assays
The assay of bacteria binding with peptide was detected according to a previously reported method (27). Briefly, 10 ml of overnight cultures of S. aureus, B. subtilis, A. hydrophila, and V. parahemolyticus were collected and adjusted to 1 × 109 CFU/ml. The bacteria were centrifuged at 5,000 × g for 5 min, washed three times, resuspended in sterile PBS, and then heat inactivated at 72°C for 20 min. For the binding assay, each peptide (5 µg, 100 µl) or control was mixed with 100 µl bacterial suspension (1.0 × 108 microbes). The mixture was then incubated at 28°C for 1 h under gentle agitation. After centrifugation 5,000 × g for 5 min and washing with PBS, the samples were centrifuged again under the same conditions, and the supernatant was removed. The bacteria pellet was resuspended in 100 µl, 0.1 M carbonate/bicarbonate buffer (pH 9.6), and used to coat a 96-well plate by incubation for 2 h at 37°C. Subsequently, the wells were blocked with blocking buffer (2% BSA in PBST) for 2 h at room temperature and then incubated with goat anti-His mouse monoclonal antibody (1:1,000) (Transgen, China) overnight at 4°C. Plates were washed with PBST before incubation with goat anti-mouse HRP-conjugated secondary antibody (1:5,000) (Transgen, China) for 1 h. To detect the binding signal, freshly prepared TMB substrate (Sigma) was added to the plates for 10 min, and the reaction was terminated by the addition of stop solution (2 M HCl). Subsequently, the absorbance of each well was measured within 30 min at a wavelength of 450 nm using a microplate reader (Thermo Scientific, USA).
The assay of bacteria binding with recombinant protein was detected according to previously reported method (16). Briefly, the purified recombinant proteins [rEsDscam(4.6,6.9), rEsDscam(4.24,6.19), and rEsDscam(4.12,6.20)] (5 µg) were mixed with 1 × 108 microbes of three strains of bacteria (S. aureus, V. parahemolyticus, and A. hydrophila). The mixture was then incubated by gentle agitation at 28°C for 1 h. The bacteria pellets were pre-washed three times with 1 ml PBS buffer (pH 8.0) and isolated by centrifugation at 5,000 × g for 5 min, then resuspended in 100 µl PBS. Finally, the samples were subjected to Western blotting using the anti-His mouse monoclonal antibody (1:1,000; Transgen, China). Bacteria incubated in the absence of the recombinant proteins were used as controls. ELISAs were also used to quantify the microorganism binding activities of rEsDscams as described above. The dissociation constants (Kd) and the maximum binding (Bmax) parameters were calculated using the GraphPad Prism version 5.0 software. Three independent ELISA experiments were performed, and the results represent the mean ± SD.
Bacteria Clearance Assays
Bacteria clearance assays were performed according to a previously reported method (32). Bacterial suspensions (S. aureus and V. parahemolyticus) were cultured overnight in LB broth at 37°C and adjusted to OD600 = 0.2. The recombinant proteins (rEsDscam4.6,6.9, rEsDscam4.24,6.19, and rEsDscam4.12,6.20) (5 µg) were then mixed with 100 µl of the suspensions (1 × 108 CFU) and incubated under gentle agitation at 28°C for 1 h. After thorough washing with PBS, the bacteria were collected by centrifugation at 5,000 × g for 5 min and resuspended in 200 µl of PBS, then injected into each crab as described earlier, and the hemolymph was collected at 30 min post-injection. After serial dilution, the number of residual bacteria was determined by plating the samples onto LB agar plates. Three to five crabs were used in each group, and each experiment was repeated three times. The results are presented as the mean ± SD. Bacteria incubated in the absence of recombinant proteins were used as controls.
Fluorescent Labeling of Bacteria and Phagocytosis Assay
Phagocytosis assays (in vitro and in vivo) were performed according to previously reported methods (16, 32–34). Overnight-cultured S. aureus and V. parahemolyticus were heat killed and fluorescein isothiocyanate (Sigma, USA) conjugated. The bacterial suspension (1 × 108 microbes per milliliter, 1 ml) in PBS was mixed and incubated with 500 µg of recombinant proteins by gentle rotation for 1 h at 28°C to ensure full coating. The bacteria were pelleted and washed three times with PBS by centrifugation.
For the in vitro analysis, cultured hemocytes (4 × 105 cells) were stimulated firstly with heat-killed bacteria (1 × 106 microbes) for 12 h and washed with PBS twice before the addition of approximately 1 × 106 (40 µl) FITC-conjugated microbes coated with recombinant proteins. After incubation for 1 h at room temperature, the cells were washed twice with PBS and stained with trypan blue (2 mg/ml) for 20 min to quench the non-phagocytosed bacteria. The cells were then washed three times with PBS, and stained with DAPI for 1 min. Subsequently, phagocytosis was observed using a fluorescence microscope (Olympus BX71, Japan). Five hundred hemocytes were counted for each sample, which was from three crab. The hemocytes (500 cells) were counted and the phagocytic rate was determined as the ratio of the number of phagocytic cells containing fluorescent bacteria to the total number of cells.
For the in vivo analysis, the bacterial suspension (100 µl) was injected into the hemolymph of crab from the one of the posterior walking legs. After 1 h, the hemocytes (6 × 105 cells) were isolated from the other one of the posterior walking legs which was the different wound-sites and centrifuged at 300 × g for 10 min at 4°C, followed by washed with PBS three times. Subsequently, the phagocytosis rate in 1 ml of each sample was determined by flow cytometry using a CytoFLEX flow cytometry (Beckman, USA), and the data were analyzed using CytExpert software. Ten thousand hemocytes were counted for each sample, which was from three crab. The experiments were repeated three times.
RNA Interference
For knockdown of EsDscam expression, a partial EsDscam non-alternatively spliced cDNA fragment was amplified by PCR with primers linked to the T7 promoter (Table 1) and used as the template to produce dsRNA with an in vitro T7 Transcription Kit (Fermentas, Burlington, ON, Canada). The control GFP dsRNA was synthesized in the same way with primers listed in Table 1. To test whether the expression of EsDscam could be suppressed, the dsRNA was then dissolved in RNase-free water and transfected into E. sinensis primary cultured hemocytes using Lipofectamine 3000 (Thermo, USA) at a final concentration of 5 nM. At least three crab were used for testing of RNAi efficiency at 24 h post dsRNA transfection. To detect the effect of EsDscam knockdown on phagocytosis, dsRNA pre-treated hemocytes were then subjected to bacteria stimulation for a further 12 h.
For knockdown of exon 4.24- and exon 6.20-containing EsDscam isoforms expression, siRNA methods were used to silence the expression of exon 4.24- and exon 6.20-containing EsDscam isoforms due to the short epitope II sequences. Briefly, siRNA against exon 4.24- and exon 6.20-containing EsDscam isoforms and GFP (as control) were synthesized by GenePharma (Shanghai, China); the primer sequences used are listed in Table 1. These siRNAs were used to transfect E. sinensis primary hemocytes using the siRNA-Mate reagent (GenePharma, China) according to the manufacturer’s instructions. At least three crab were used for testing of RNAi efficiency at 24 h post siRNA transfection.
After validating that targeted gene expression could be silenced by the dsRNA and siRNA, crab hemocyte were used for RNAi, which divided into four parts. The first part was hemocyte transfected each with 5 nM dsRNA, the second part was hemocyte transfected each with 5 nM siRNA, and the last two parts were hemocyte each for GFP dsRNA and GFP siRNA transfection as control. After bacteria pre-stimulation, hemocyte were stimulated by bacteria coated with recombinant proteins for another 1 h and phagocytosis were analyzed as described earlier. Results were analyzed by Student’s t-test and expressed as the mean ± SD from three independent experiments.
Immunocytochemistry
Hemocyte immunocytochemistry was performed according to a previously reported method (34). Briefly, the cultured primary hemocytes (approximately 4 × 105 cells) were seeded onto a 24 mm × 24 mm glass cover slips in 35-mm dishes. After RNAi-mediated knockdown of EsDscam expression or stimulation with bacteria, the cells were washed twice with PBS and fixed immediately with 4% paraformaldehyde in sterile PBS for 15 min. The hemocytes were washed again with PBS and exposed to 0.5% Triton X-100 for 10 min. After washing three times with PBS, the hemocytes were blocked with 3% BSA in PBS for 2 h and incubated with polyclonal rabbit antiserum against EsDscam (1:200) and anti-His mouse monoclonal antibody (1:1,000; Transgen, China) overnight at 4°C. After washing with PBST for removal of unbound antibody, cells were stained with goat anti-rat IgG H&L (FITC Conjugated) (1:100; CWBIO, China) and goat anti-mouse IgG H (Rhodamine Red-X Conjugated) (1:100; CWBIO, China) for 2 h at 37°C. After washing with PBST, cells were stained with DAPI for 1 min, and the cover slips were removed for observation under a fluorescence microscope (Olympus BX71, Japan).
Far-Western Blotting and Co-immunoprecipitation Assays
The interactions between the same and different isoforms of EsDscam in vitro were evaluated using the far-Western blotting assays performed according to previously described methods with slight modifications (35, 36). Briefly, rEsDscam4.24,6.19-His (50 µg) was separated by 12% SDS-PAGE and transferred to a nitrocellulose membrane. The membrane containing the bait protein was then washed with denaturation buffer [6 M guanidine–HCl in basic buffer (10% glycerol, 100 mM NaCl, 1 mM EDTA, 1 mM DTT, 1% Tween-20, 20 mM Tris, pH 7.5)] at room temperature for 30 min with gentle agitation. The membrane was then washed sequentially in 3 M guanidine–HCl for 30 min at room temperature, in 1 M guanidine–HCl for 30 min at 4°C, and in 0.1 M guanidine–HCl for 30 min at 4°C. Finally, the membrane was washed in basic buffer for 30 min and blocked with the blocking buffer (2% non-fat milk in basic buffer) for 2 h. Subsequently, the crab plasma protein (1 mg), His peptide (1 mg), rEsDscam4.24,6.19-myc (1 mg), or rEsDscam4.12,6.20-myc (1 mg) was added to the interaction buffer (2% milk in basic buffer) at 4°C overnight. The non-interacting proteins were then removed by washing in PBST, and the bound proteins were detected with anti-EsDscam (1:500), anti-His (1:1,000; Transgen, China), and anti-Myc (1:1,000; Transgen, China) antibodies by Western blotting.
No crab or crustacean cell lines are currently available; however, the Drosophila S2 cell line has been widely used to study the function of crustacean genes (37–39). To confirm the interaction between the same and different isoforms of Dscam in vivo, S2 cells were cultured for coimmunoprecipitation assays according to previously reported methods (39). Briefly, Drosophila S2 cells were seeded in 24-well plates and cultured at 28°C overnight in Schneider’s insect medium (Sigma) supplemented with 10% FBS (Life Technologies, USA). After 24 h, cells were cotransfected with pAc5.1-V5-His A, pAc5.1-rEsDscam4.12,6.20-V5 or pAc5.1-rEsDscam4.24,6.19-His, and pAc5.1-rEsDscam4.24,6.19-V5 using Lipofectamine® 3000 transfection reagent (Thermo, USA) according to the manufacturer’s instructions. pAc5.1-V5-His A and pAc5.1-rEsDscam4.12,6.20-V5 were used as controls. At 36 h post-transfection, S2 cells were washed twice with PBS before proteins were extracted using RIPA buffer followed by centrifugation at 14,000 × g for 10 min. The supernatant containing a pool of rEsDscam4.24,6.19-His and rEsDscam4.24,6.19-V5 proteins was pre-cleared with 30 µl of Protein A beads at 4°C for 40 min with agitation. Subsequently, the mixture was centrifuged at 12,000 × g for 10 min to remove the beads. The supernatant was then incubated with 10 µg His and V5 antibodies overnight at 4°C under gentle rotation. Protein A beads were then added to the mixture and incubated for 1 h at 4°C to capture the antibodies. The beads were collected by centrifugation and then washed with PBS and resuspended in the SDS-PAGE loading buffer for separation by SDS-PAGE and Western blot analysis using the His or V5 antibody.
Results
Genome Organization and Alternatively Spliced Exons
The organization of the Dscam gene in arthropods usually comprises clusters of variable exons flanked by constant exons (14, 40). The EsDscam gene (GenBank accession number: KT175608) (Figure 1A) contains 114 exons, of which 40 account for the mature mRNA (Figure 1B, middle panel) encoding the protein structure that shares high similarity with D. melanogaster Dscam (Figures S1A,B in Supplementary Material). The alternative splicing regions are located in sequences encoding the extracellular and transmembrane regions (Figure 1B, upper panel). Similar to other arthropod species (14, 23, 41, 42), the extracellular region of EsDscam has three hypervariable sites: the exon 4 cluster that encodes part of the Ig2 domain and has 25 variants, the exon 6 cluster that encodes part of the Ig3 domain and has 34 variants, and the exon 14 cluster that encodes the entire Ig7 domain and has 18 variants (Figure 1B, lower panel). In addition, there are two variants of the transmembrane domain (TM) region (Figure 1B, lower panel). The variable number of spliced exons in arthropod species confers the potential for expression of different isoforms, and even the lowest number maintained a high degree of divergence of 104 (Figure 1C). Taken together, these findings demonstrated that EsDscam forms the domain organization of the 9(Ig)-4(FNIII)-Ig-2(FNIII)-TM-cytoplasmic tail, with the possible generation of 30,600 isoforms via alternative splicing of three Ig domain regions (Ig2, Ig3, and Ig7) and a TM.
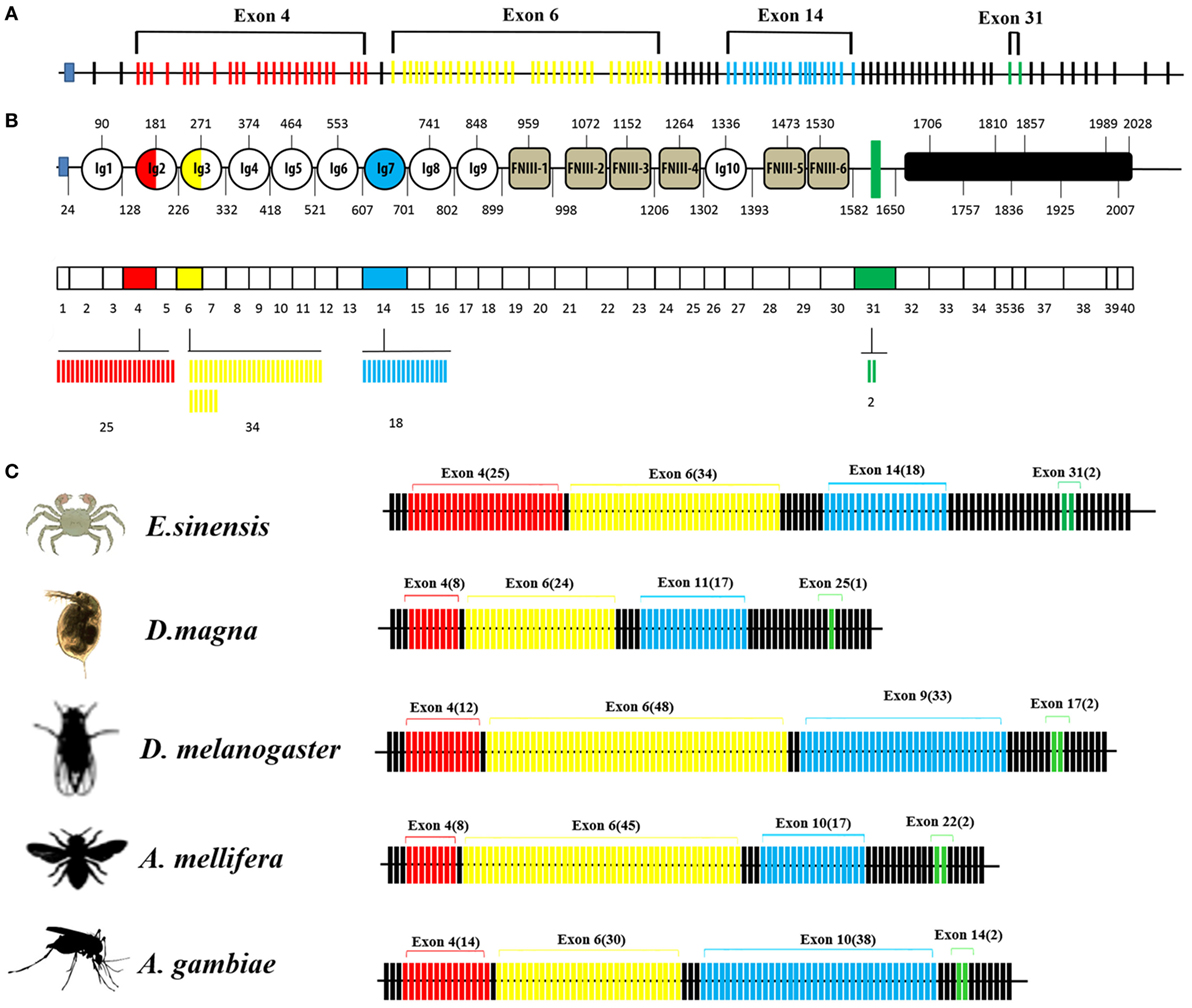
Figure 1. Genome structure of Dscam gene. (A) Eriocheir sinensis Dscam genomic DNA structure. EsDscam mRNA comprises 40 exons (short lines); 36 constant exons are indicated by black lines and 4 hypervariable exons are indicated by colored lines. Exon 4 contains 25 variants (red), exon 6 contains 34 variants (yellow), exon 14 contains 18 variants (blue), and exon 31 contains 2 variants (green). (B) EsDscam protein domain and mRNA structure. (Upper panel) The alternatively spliced exons encode the N-terminal half of Ig2 (red semicircle) and Ig3 (yellow semicircle), all of Ig7 (blue circle), and the transmembrane domain (green rectangle); (middle panel) constant exons are shown as white boxes and the mutually exclusive alternative splicing exons are shown in the same color scheme as that used for the protein structure. EsDscam mRNAs contain only one of each of the alternative splicing exons; (lower panel) the variant numbers of mutually exclusive alternative splicing exons are indicated by colored lines using the same color scheme as that used for the protein structure. (C) Comparison of Dscam gene within arthropod species. The number of exons is shown. Black lines represent constant exons. The hypervariable exons in the exon 4, 6, 9, and 17 clusters of Drosophila melanogaster are shown in red, yellow, blue, and green, respectively. The number of variable exons within each array in each species is displayed above each array. The exon 10 arrays of Anopholes gambiae and A. mellifera, the exon 11 array of D. magna and the exon 14 array of Eriocheir sinensis correspond to the exon 9 array of Drosophila. Similarly, the exon 14 and 22 arrays of A. gambiae and A. mellifera, the exon 25 array of D. magna and the exon 31 array of E. sinensis correspond to the exon 17 array of Drosophila.
EsDscam Is Upregulated in Hemocytes Post-Bacterial Stimulation
EsDscam protein expression was analyzed by Western blotting using anti-EsDscam serum (Figure S2 in Supplementary Material) generated using the purified extracellular region of FNIII3 to FNIII6 (Figure 2A), and with preimmune serum as negative controls. The results showed that non-specific signals from the antibodies were not detected, so it is acceptable for use in subsequent studies. EsDscam expression was detected in hemocytes, intestine, heart, brain, stomach, and cell-free hemolymph after pathogen stimulation (Figures 2B,C). The expression of Dscam in hemocytes has been widely confirmed in arthropod species (13, 18, 20, 27, 31, 43, 44), its expression in E. sinensis hemocytes post-pathogen stimulation remains to be established, although it has been tested in E. sinensis in relation to PAMPs (Glu, LPS, and PG) stimulation (43). In this study, qRT-PCR analysis of the temporal expression patterns of EsDscam in hemocytes after bacterial stimulation showed that EsDscam was significantly upregulated and peaked at 6 h (approximately fourfold upregulation) post-stimulation with S. aureus (Figure 2D), at 48 h (approximately eightfold upregulation) post-stimulation with B. subtilis (Figure 2E), at 12 h (approximately eightfold upregulation) post-stimulation with A. hydrophila (Figure 2F), and at 6 h (approximately sevenfold upregulation) post-stimulation with V. parahemolyticus (Figure 2G). However, no suitable primers were available to distinguish soluble Dscam from membrane-bound Dscam since the two forms express the same extracellular region (13), therefore we were unable to analyze the expression profile of soluble EsDscam using this strategy. To address this issue, the expression profile of soluble EsDscam protein in the cell-free hemolymph post-bacterial stimulation was analyzed by Western blotting. The results revealed marked upregulation of EsDscam post-stimulation with S. aureus, B. subtilis, A. hydrophila, and V. parahemolyticus (Figure 2H), indicating that soluble EsDscam may play a role in antimicrobial responses.
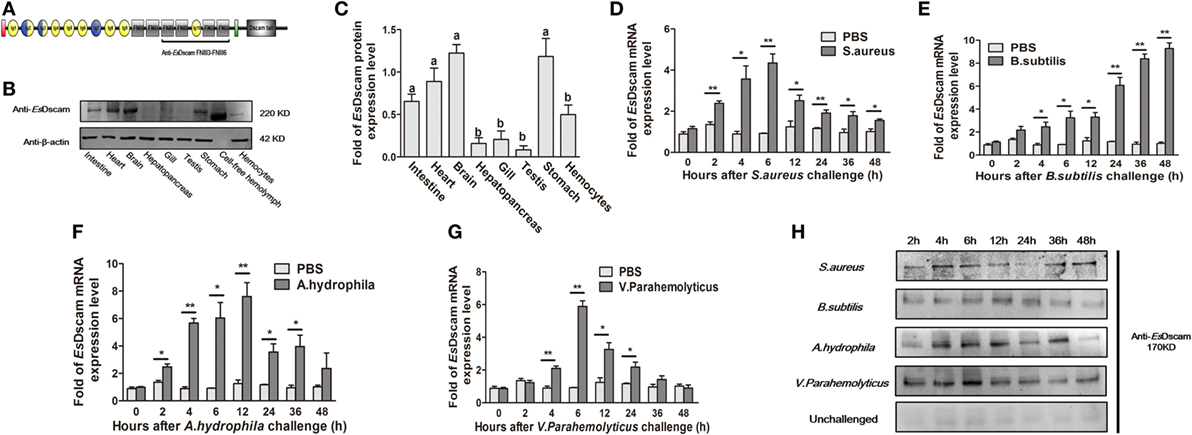
Figure 2. Identification of EsDscam as a bacteria-sensitive gene in crab hemocyte. (A) Schematic diagram of the conserved EsDscam FNIII3–FNIII6 region recognized by the EsDscam Ab. (B,C) Tissue distribution of EsDscam. (Left panel) The protein expression was analyzed by Western blot with β-actin detected as the reference. Each sample was from four crab. (Right panel) ImageJ was used to scan the Western blot bands from three independent repeats. Relative expression level of EsDscam/β-actin are shown, and the value are expressed as relative arbitrary units. Results were analyzed by one-way ANOVA and the letters (a, b) presented significant differences (p < 0.05). (D–G) Expression profile of EsDscam mRNA after bacterial stimulation in the hemocytes. RNA was extracted at each time point from experimental and control crab. qRT-PCR was performed to examine the expression of EsDscam in each sample with β-actin as the reference gene. The expression was normalized to that in PBS stimulated crab. Three independent repeats were performed with at least three crab for each sample, and results are expressed as the mean ± SD. Data were analyzed by Student’s t-test. *p < 0.05, **p < 0.01. (H) Expression profile of soluble EsDscam protein in hemocyte culture medium after bacterial stimulation. The protein expression was analyzed by Western blot with unchallenged hemocyte as the control. Data are representative of two independent repeats.
Dscam Alternative Splicing in Response to Infection
A global assessment of Dscam alternative splicing in D. melanogaster using custom-made oligo-arrays demonstrated that almost all alternative exon 4 and exon 6 sequences were expressed in fat bodies and hemocytes, while only a limited subset of exon 9 sequences were expressed in these cell types (13). Furthermore, challenge of the hemocyte-like immune-competent cell line, Sua5B, with bacteria, fungi, and pathogen-associated surface molecules resulted in rapid and specific changes in exon usage in an acute phase-responsive manner (16). To investigate the alternative splicing pattern of EsDscam in hemocytes post-bacterial stimulation, two gene specific primer pairs (Table 1) were designed to amplify alternatively spliced Ig2 and Ig3 regions. Bacteria were found to induce obvious variation in EsDscam splice forms, while stimulation with PBS resulted in the predominant production of splice forms containing exon 4.15 (Figure 3A) and exon 6.12 (Figure 3B). Stimulation with S. aureus resulted in the predominant production of splice forms containing exon 4.24 (Figure 3A) and exon 6.19 (Figure 3B). Stimulation with B. subtilis resulted in the predominant production of splice forms containing exon 4.7/4.8/4.9/4.10 (Figure 3A) and exon 6.5 (Figure 3B). Stimulation with A. hydrophila resulted in the predominant production of splice forms containing exon 4.8 (Figure 3A) and exon 6.10 (Figure 3B). Stimulation with V. parahemolyticus resulted in the predominant production of splice forms containing exon 4.12/4.15 (Figure 3A) and exon 6.20 (Figure 3B). These result suggested that the expression profile of Dscam isoforms may varied upon different bacteria stimulation, and the predominant exons may provide us some clues for constructing recombinant proteins that specific binding with the original bacteria. The partition of exon 4 and exon 6 epitopes was assigned based on the crystal structure of D. melanogaster Dscam (21) and the secondary structure of Dscam (Figure S1C in Supplementary Material). For comparisons of the similarities and phylogenetics among the epitopes, we analyzed the sequence conservation of epitopes I and II of exons 4 and 6 in E. sinensis (Figure 3C), which showed low conservation for both epitopes I and II. These results were similar with the previous report (21) that within a species that epitope I is low conserved, while across species that epitope I is comparative highly conserved. However, the potential role of epitope II mediated non-Dscam ligands binding (21) has not been empirically tested so far.
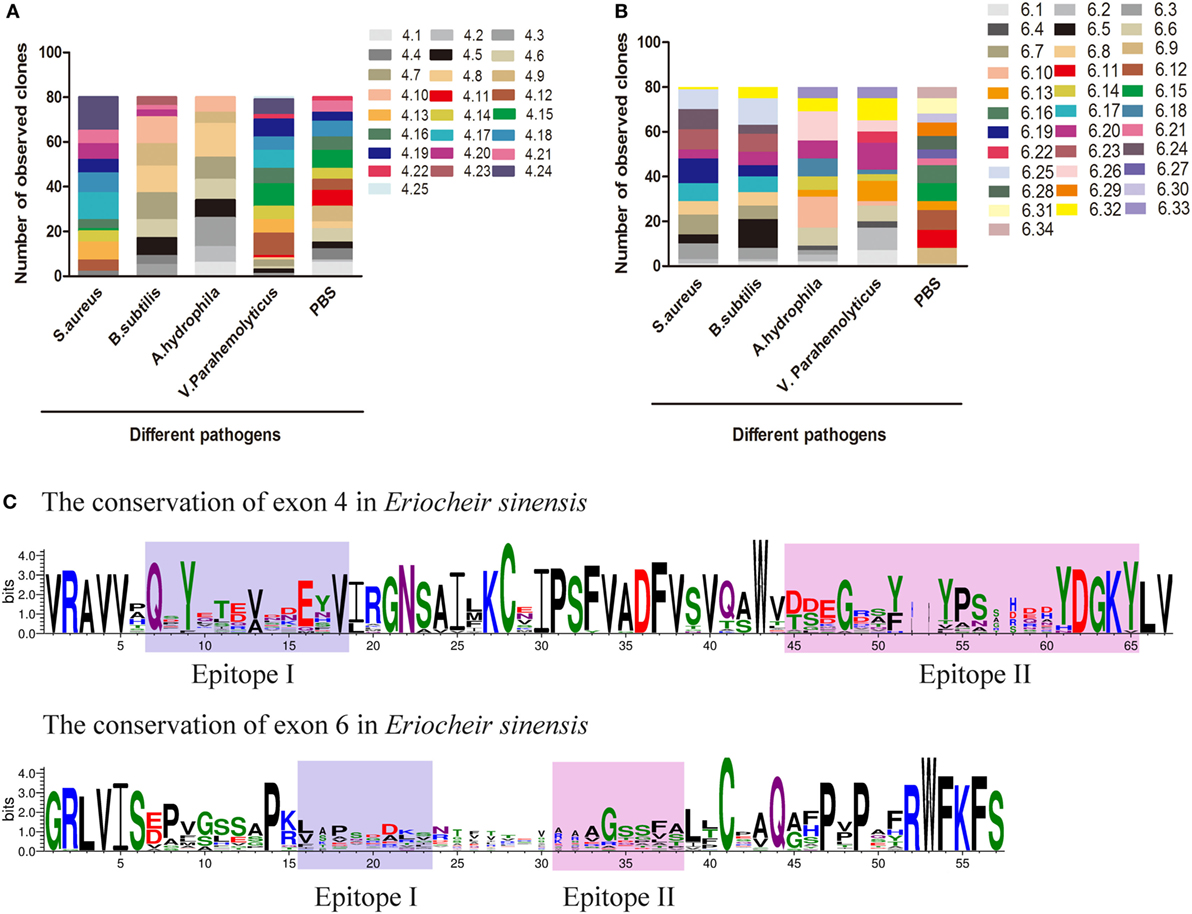
Figure 3. Preferential variants of exon 4 and exon 6 after bacterial stimulation in hemocyte. (A) Number of observed clones of exon 4 in hemocytes post-challenged with various bacteria and PBS (control). The variants of exon 4 were analyzed by cloning and sequencing 80 clones from each group. Individual colonies were randomly selected from each sample. All assays were repeated on two independent occasions, and values represent the mean number of observed clones from two independent experiments. The serial number of exon 4/6 was assigned and the amino-acid sequences of exon 4/6 are shown in Figure S3 in Supplementary Material. (B) Number of observed clones of exon 6 post-challenge with various bacteria and PBS. (C) Differential sequence conservation of epitopes I and II of EsDscam following alternative splicing of exon 4 and exon 6. (Upper panel) Sequence logo representation of the conservation of exon 4 variants in Eriocheir sinensis; (lower panel) sequence logo representation of the conservation of exon 6 variants in E. sinensis. Bits (y-axis) indicate units of evolutionary conservation.
EsDscam-Specific Binding With Bacteria
Alternative splicing allows Dscam to produce a broad repertoire of receptors, thereby increasing the probability of recognizing and defending against a broad spectrum of pathogens (45). To confirm the existence of bacteria-specific EsDscam isoforms, we first analyzed the epitope II sequences in every alternatively spliced exon of the Ig2 and Ig3 regions, and classified into five or six groups based on the similarity of these sequences (Figure S1E in Supplementary Material). Subsequently, we synthesized 11 peptides based on the epitope II sequence divergence and the predominant production of splice forms detected following bacterial stimulation. The synthesized 11 peptides contains 5 peptides from epitope II from the exon 4, 5 peptides from epitope II from the exon 6, and control peptide from the non-alternative splicing region of EsDscam gene (Figure S1D in Supplementary Material). Exons 4.24 and 6.19 were implicated in strong binding activity with S. aureus (Figure 4A), exon 4.8, with B. subtilis (Figure 4B), exons 4.8 and 6.10, with A. hydrophila (Figure 4C), and exons 4.12 and 6.20, with V. parahemolyticus (Figure 4D). These results indicated that exon 4.24 and 6.19 involved EsDscam isoform may specific binding with S. aureus, exon 4.12 and 6.20 involved EsDscam isoform may specific binding with V. parahemolyticus. However, since it is difficult to synthesis all epitope II sequences in every alternative spliced exon, we cannot draw the whole picture of bacteria-specific binding exons at this time, and we think epitope II from some untested exons might also specific binding with particular bacteria.
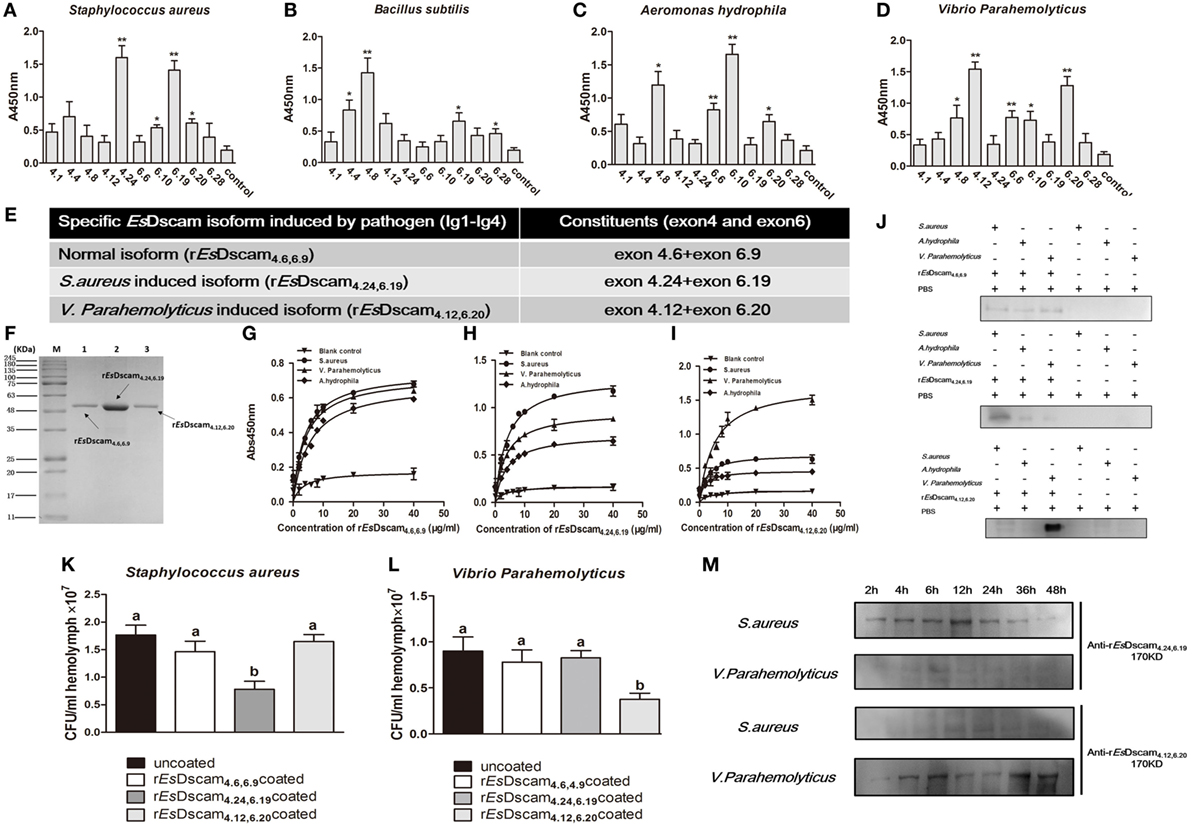
Figure 4. Soluble EsDscam-specific binding with bacteria and promotes its clearance. (A–D) Bacterial binding specificity of epitope II in exon 4/exon 6. The binding activity between selected peptides and different bacteria were analyzed by ELISA with peptides that containing the conserved motif as control. Three independent repeats were performed, and results are expressed as the mean ± SD. Data were analyzed by Student’s t-test. *p < 0.05, **p < 0.01. (E) Specific recombinant EsDscam isoforms induced by pathogens. Normal isoform composed of alternative spliced exon 4.6, alternative spliced exon 6.9, and constant exon designated rEsDscam4.6,6.9; Staphylococcus aureus-induced isoform composed of alternative spliced exon 4.24, alternative spliced exon 6.19, and constant exons designated rEsDscam4.24,6.19; Vibrio parahemolyticus-induced isoform composed of alternative spliced exon 4.12, alternative spliced exon 6.20, and constant exons designated rEsDscam4.12,6.20. (F) Purified recombinant specific EsDscam isoform. Three recombinant rEsDscam protein were expressed from the pET-28a (+) vector in Escherichia coli Rosetta (DE) cells and purified by affinity chromatography, followed by SDS-PAGE detection. (G–I) Quantitative binding of EsDscam with bacteria. Microtiter plates were coated with heat-inactivated bacteria which were pre-coated with serial concentrations of proteins and air dried at 37°C for 2 h. After the wells were blocked with 2% BSA, the bound proteins were then detected by ELISA using an anti-His-tag Ab. Data represent the mean ± SD of three independent experiments. (J) Microorganism binding assays of rEsDscam proteins. The coated samples of bacteria with or without rEsDscam protein and PBS were boiled for 10 min and separated by 12% SDS-PAGE for Western blotting using an anti-His-tag antibody, with PBS incubated with microorganisms as control. Data are representative of two independent repeats. (K,L) EsDscam promotes bacteria clearance in crab. Each rEsDscam (5 µg) was incubated with 100 µl of the S. aureus (left panel) or V. parahemolyticus (right panel) suspension (OD600 = 0.2) for 1 h. The bacteria were washed and suspended in 200 µl of PBS. Then, 200 µl of the suspension was injected into the crab, and hemolymph was collected 30 min later. The number of residual bacteria in the hemolymph was determined by plating onto LB agar plates. Uncoated bacteria were used as the control. Three to five crabs were used for each group. Three independent repeats were performed, and results are expressed as the mean ± SD. Data were analyzed by one-way ANOVA and the letters (a, b) presented significant differences (p < 0.05). (M) Specific EsDscam was highly induced after bacteria stimulation in cell-free hemolymph. Protein from cell-free hemolymph was extracted at each time point after bacterial stimulation and analyzed by Western blot using anti-rEsDscam4.24,6.19 and anti-rEsDscam4.12,6.20 antibodies. Each sample was from four crab. Data are representative of two independent repeats.
To test the ability of EsDscam(4.24, 6.19) and EsDscam(4.12, 6.20) binding specifically with its inducing bacteria, we used a prokaryotic expression system to generate recombinant proteins, with rEsDscam(4.6, 6.9) protein as control (Figures 4E,F; Figure S4 in Supplementary Material). ELISA and Western blot analyses showed that rEsDscam(4.6, 6.9) bound all the bacterial strains tested with low affinity (Figures 4G,J, upper panel), while rEsDscam(4.24, 6.19) bound S. aureus with higher affinity than either A. hydrophila or V. parahemolyticus (Figures 4H,J, middle panel), and rEsDscam(4.12, 6.20) bound only V. parahemolyticus with high affinity (Figures 4I,J, lower panel). Furthermore, in vivo bacteria clearance assays in E. sinensis showed that S. aureus was only cleared efficiently when pre-coated with rEsDscam(4.24, 6.19) (Figure 4K), while V. parahemolyticus was only cleared efficiently when pre-coated with rEsDscam(4.12, 6.20) (Figure 4L). These results revealed the specific binding activity of bacteria-induced EsDscam splice forms only with the original inducing bacteria; however, significant induction of these specific EsDscam isoforms in cell-free hemolymph post-bacterial infection remained to be confirmed. To address this issue, we detected the levels of EsDscam(4.24, 6.19) and EsDscam(4.12, 6.20) proteins in hemolymph at different time-points post-infection by Western blot analysis. EsDscam(4.24, 6.19) expression was found to be induced significantly by S. aureus rather than V. parahemolyticus, while soluble EsDscam(4.12, 6.20) was induced significantly by V. parahemolyticus but not by S. aureus (Figure 4M), which implies the role of soluble EsDscam in immune defense.
Bacteria-Specific Binding by Es-sDscam Promotes Phagocytosis
Dscam-regulated phagocytosis has been confirmed in some other arthropod species (13, 16, 46); therefore, we investigated the ability of Es-sDscam to reduce the number of bacteria via phagocytosis regulation and the function of bacteria-specific binding in this process. For this purpose, the hemocytes were pre-stimulated with the bacteria for 12 h, and we pre-coated different strains of FITC-labeled bacteria with rEsDscam(4.24, 6.19) and rEsDscam(4.12, 6.20), then performed the phagocytosis assay in vitro. In vitro phagocytosis assays using different strains of FITC-labeled bacteria showed that rEsDscam(4.24, 6.19) enhanced the rate of S. aureus phagocytosis by approximately 100% (Figures 5A,B) and rEsDscam(4.12, 6.20) enhanced the rate of V. parahemolyticus phagocytosis by approximately 70% (Figures 5C,D). By contrast, rEsDscam(4.24, 6.19) and rEsDscam(4.12, 6.20) had no significant effect on the rate of V. parahemolyticus and S. aureus phagocytosis (Figures 5A–D). These results were confirmed in vivo by flow cytometry to differentiate hemocytes from bacteria and cell debris (Figure 5E). The results also showed that bacteria-specific binding by Es-sDscam promoted a marked increase in phagocytosis of the original inducing bacteria, while only a marginal effect on the non-specific binding bacteria was observed (Figure 5E). These results demonstrated an essential role of bacteria-specific binding in Es-sDscam-promoted phagocytosis.
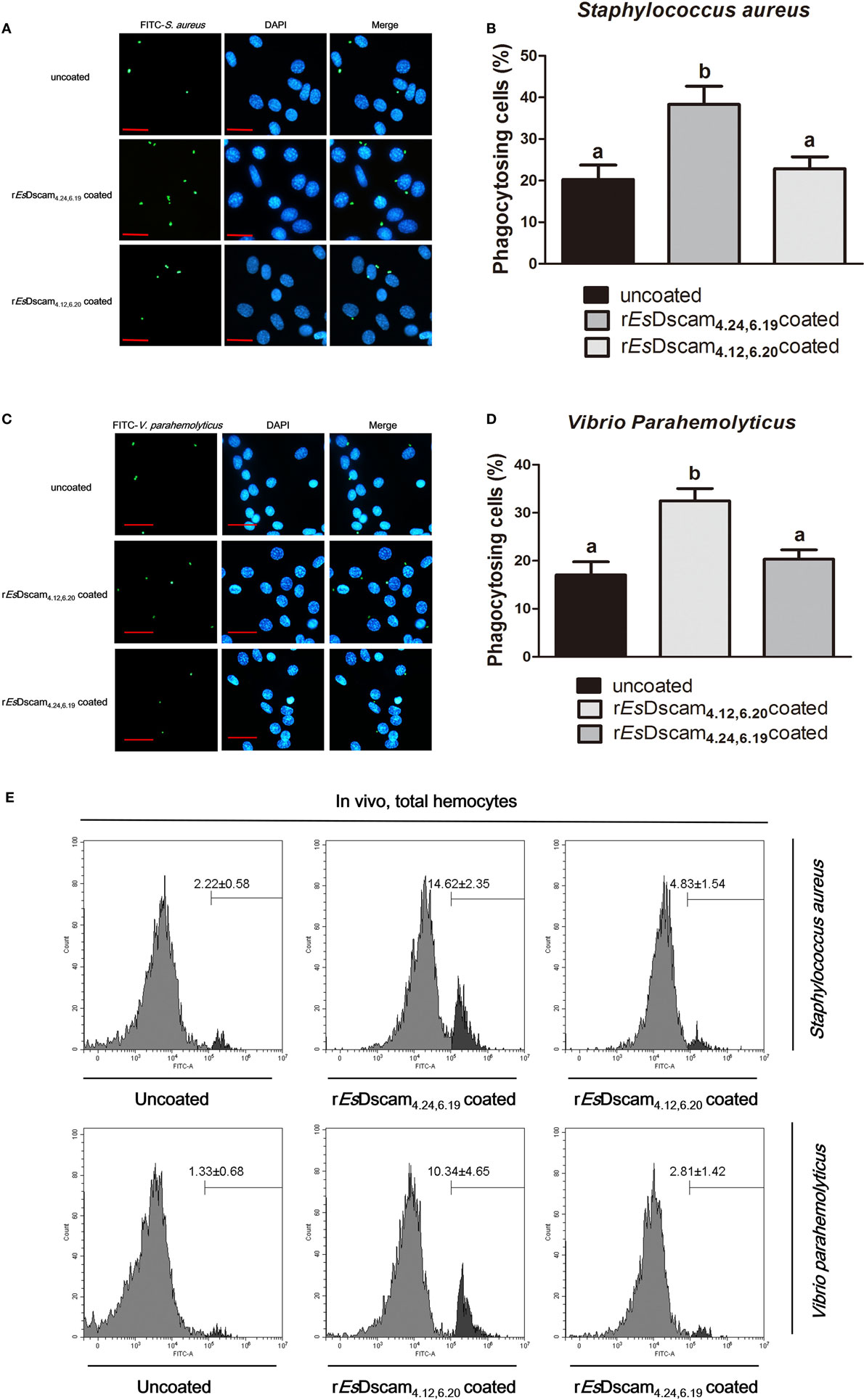
Figure 5. Soluble EsDscam enhanced the phagocytosis of bacteria in hemocytes. (A,B) rEsDscam4.24,6.19 promote phagocytosis of Staphylococcus aureus in vitro. S. aureus (1 × 106 microbes) labeled with FITC and then coated with rEsDscam4.24,6.19 or rEsDscam4.12,6.20 protein was used to stimulate cultured hemocyte, with uncoated bacteria as control. Hemocytes was then collected 1 h later, washed with PBS. After staining with DAPI and quenching with trypan blue, hemocytes were observed under a fluorescence microscope. Scale bar = 15 μm. The phagocytosis (left panel) were detected by fluorescence microscopy, data representative three independent repeats. The phagocytosis rate (right panel) were calculated according to the formula presented in Section “Materials and Methods” based on counting 500 hemocytes in each experiment. Three independent repeats were performed, and results are expressed as the mean ± SD. Data were analyzed by one-way ANOVA and the letters (a, b) presented significant differences (p < 0.05). (C,D) rEsDscam4.12,6.20 promote phagocytosis of Vibrio parahemolyticus in vitro. The same bacteria concentration, as well as the protocol and statistical method were used as described earlier. (E) EsDscam promote phagocytosis of bacteria in vivo. S. aureus and V. parahemolyticus were heat-inactivated and labeled with FITC before coating with rEsDscam4.24,6.19 and rEsDscam4.12,6.20, respectively. The bacteria were then injected into the hemolymph of crab and hemocytes were collected 1 h later for flow cytometric analysis. A total 10,000 hemocytes were counted for each sample. Three independent repeats were performed, and results are expressed as the mean ± SD.
Truncated Es-sDscam Abolished the Promotion of Phagocytosis
X-ray crystallography of the structure of the amino-terminal four Ig domains (Ig1–Ig4) of two distinct Dscam isoforms in D. melanogaster revealed a horseshoe configuration (21). Since Ig1 and Ig4 are constant domains, this configuration should be a general feature of the structure of all Dscam isoforms (21). The 3D-structure of the amino-terminal four Ig domains (Ig1-Ig4) of rEsDscam isoform also revealed a horseshoe configuration (Figure S1C in Supplementary Material), which was very similar to one Dscam isoform in D. melanogaster. Therefore, we hypothesized that the complete Ig1–Ig4 structure may play a critical role in bacteria-specific binding and the subsequent regulation of phagocytosis. To test that hypothesis, we used a prokaryotic expression system to generate three truncated recombinant Es-sDscam proteins (Ig1–Ig2, Ig2–Ig3, and Ig3–Ig4) based on rEsDscam(4.24, 6.19) (Figures 6A,B; Figure S4 in Supplementary Material). Evaluation of bacterial binding by ELISA and Western blotting demonstrated that these truncated rEsDscam proteins retained the ability to bind bacteria, albeit with impaired specificity (Figures 6C,D). Briefly, the truncated recombinant proteins had almost no effect on phagocytosis compared with negative control (Figure 6F). To confirm these results in vivo, flow cytometry was conducted to analyze the phagocytosing cells among the total hemocytes. The results obtained were in accordance with the in vitro study data showing that truncated Es-sDscam has only a slight effect on the promotion of phagocytosis compared with that induced by rEsDscam(4.24, 6.19) (Figure 6G). Collective results demonstrated the role of the complete EsDscam Ig1-Ig4 structure in the promotion of phagocytosis, while the truncated recombinant proteins had almost no effect on phagocytosis, and prompted us to explore the possible mechanism underlying this phenomenon.
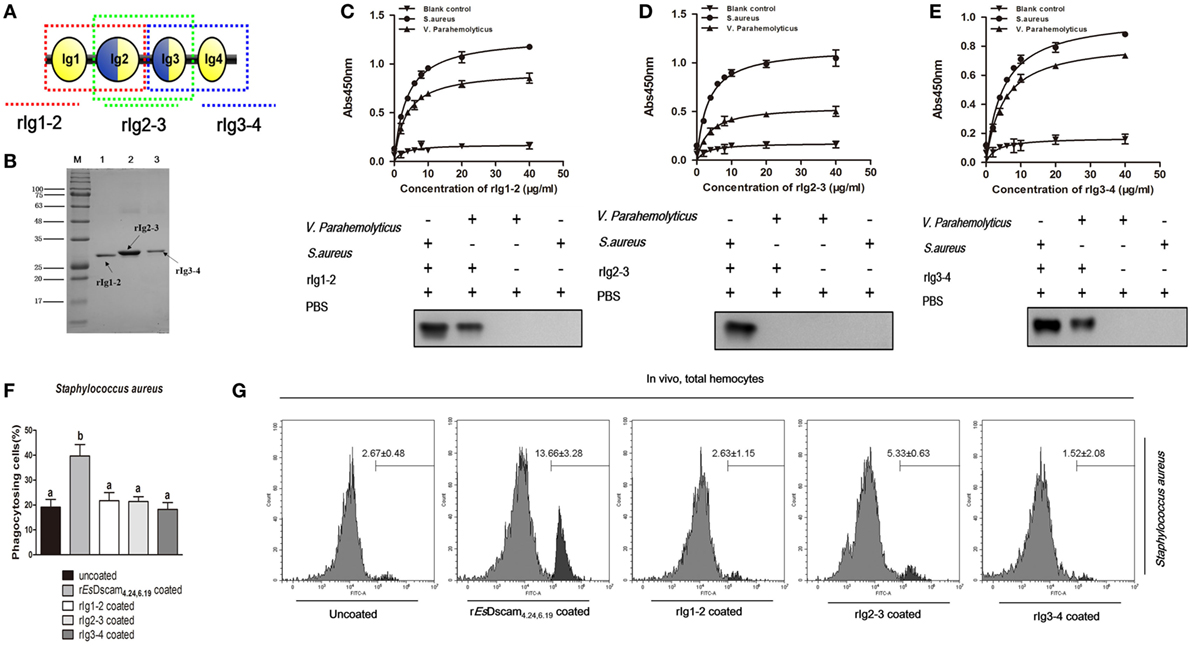
Figure 6. Truncated soluble EsDscam exhibit variation in bacteria-binding ability and loss of the capacity to promote phagocytosis. (A) Schematic diagram of truncated rEsDscam4.24,6.19. The red, green, and blue dotted lines represents the rIg1–2, rIg2–3, and rIg3–4 proteins, respectively. The three domains were expressed in vitro using a prokaryotic expression system (results of the construction, expression, and purification of the recombinant isoforms are shown in Figure S4 in Supplementary Material). (B) Recombinant truncated soluble EsDscam proteins were analyzed by SDS-PAGE. Three proteins were expressed from the pET-28a (+) vector in Escherichia coli Rosetta (DE) cells and purified by affinity chromatography. (C–E) Quantitative binding of truncated rEsDscam proteins with bacteria. The binding activity between truncated rEsDscam proteins and bacteria were detected by ELISA using anti-His-tag antibodies. PBS incubated with microorganisms was used as the control. Absorbance data (upper panel) represent the mean ± SD of three independent experiments. Western data (lower panel) are representative of two independent repeats. (F) Truncated EsDscam has slight effect on phagocytosis of bacteria in vitro. Each recombinant protein was incubated with FITC-labeled Staphylococcus aureus and then used to stimulate hemocyte, with rEsDscam4.24,6.19 as positive control. Three independent repeats were performed, and results are expressed as the mean ± SD. Data were analyzed by one-way ANOVA and the letters (a, b) presented significant differences (p < 0.05). (G) Truncated EsDscam has slight effect on phagocytosis of bacteria in vivo. S. aureus was heat-inactivated and labeled with FITC before coating with each rEsDscam protein. The bacteria were then injected into the hemolymph of crab and hemocytes were collected 1 h later for flow cytometry analysis, with uncoated bacteria as control. A total 10,000 hemocytes were counted for each sample. Three independent repeats were performed, and results are expressed as the mean ± SD.
Membrane-Bound EsDscam Regulated the Promotion of Phagocytosis by Soluble EsDscam
Phagocytosis, which is an essential process performed by unicellular organisms and many cell types found in metazoans, begins with the engagement of phagocytic receptors that activate numerous signaling pathways (46). In mammals, antibody-bound (opsonized) pathogens are recognized by Fc receptors (46); however, in arthropods, it is unclear whether soluble Dscam-opsonized bacteria are recognized by a dedicated receptor or through homotypic interactions with membrane-bound Dscam. To test the function of membrane-bound EsDscam as a phagocytic receptor in soluble EsDscam-regulated phagocytosis, we used dsRNA targeting the transmembrane region of Es-mDscam (Figure 7A) to knockdown expression to approximately 10% of the level detected in the control (Figures 7B,C). The efficiency of the RNAi-mediated reduction in expression was also confirmed by immunohistochemical analysis of Es-mDscam protein expression (Figure 7D). Using this approach, we showed that Es-mDscam knockdown caused a significant reduction in the rate of phagocytosis in S. aureus- and V. parahemolyticus-stimulated hemocytes (Figures 7E,F). More importantly, Es-mDscam knockdown significantly inhibited EsDscam(4.24, 6.19)-induced S. aureus phagocytosis (Figure 7E) and EsDscam(4.12, 6.20)-induced V. parahemolyticus phagocytosis (Figure 7F). These observations demonstrated the role of membrane-bound EsDscam in soluble EsDscam-regulated phagocytosis. In addition, hemocyte phagocytosis was reduced by knockdown of Es-mDscam or culture with rEsDscam(4.24, 6.19). Although these results suggest the participation of Es-mDscam in Es-sDscam-regulated phagocytosis, whether soluble Dscam-opsonized bacteria are recognized through membrane-bound Dscam remains to be clarified.
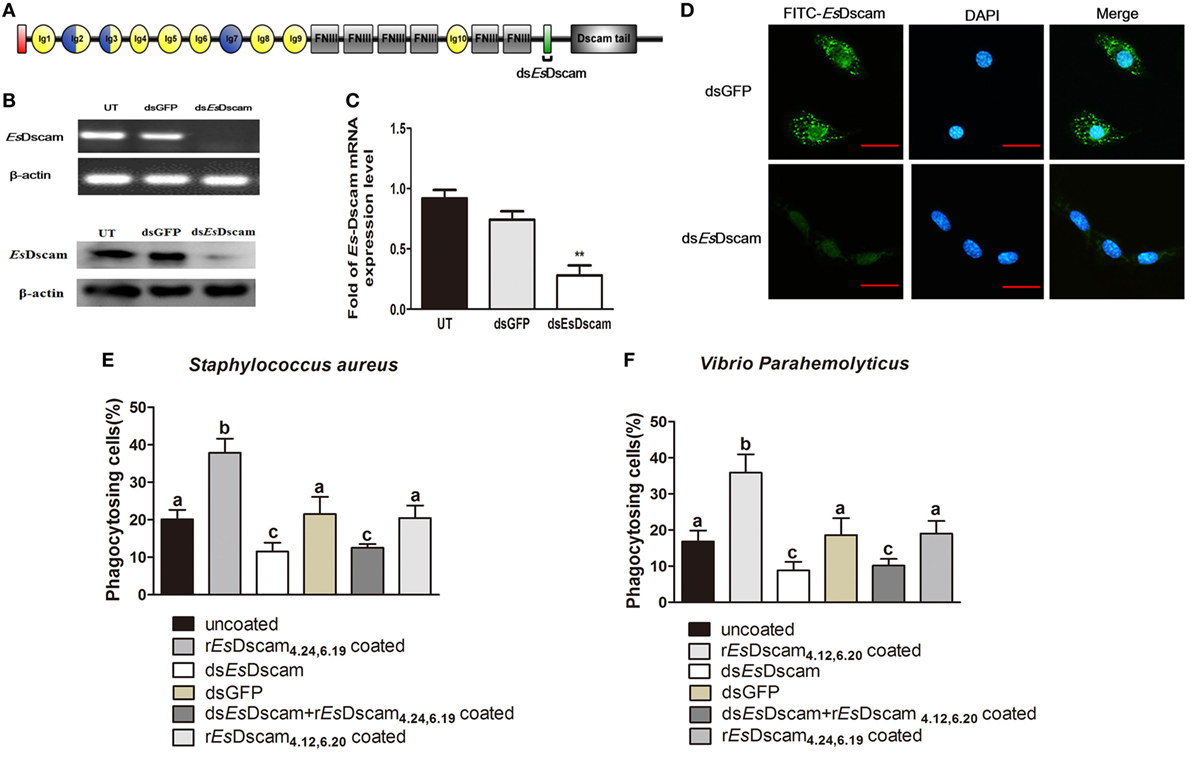
Figure 7. Soluble EsDscam promotes phagocytosis via membrane-bound EsDscam in hemocytes. (A) Schematic diagram showing the RNAi region recognized by primers in the conserved transmembrane region of dsEsDscam. (B–D) Knockdown of EsDscam expression. Crab hemnocyte were transfected with EsDscam dsRNA (5 nM) and equal amounts of GFP dsRNA served as a control. RNA samples were collected at 24 h post-transfection to check the silencing efficiency by RT-PCR (left panel) and qRT-PCR (right panel) with β-actin as a reference. And the protein samples also were collected and detected by Western blotting with β-actin as a reference. RT-PCR data are representative of two independent repeats. At least three crab were used for each sample, and qRT-PCR were performed three times. The results are expressed as the mean ± SD, data were analyzed by Student’s t-test. **p < 0.01. (Right panel) Immunocytochemical analysis of EsDscam dsRNA transfected hemocyte showing decreased EsDscam protein expression compared with dsGFP-treated control cells. Scale bar = 15 µm. Data are representative of three independent repeats. (E,F) Membrane-bound EsDscam regulate soluble EsDscam promoted phagocytosis in hemocyte. Rate of hemocyte phagocytosis of (E) Staphylococcus aureus and (F) Vibrio parahemolyticus following EsDscam knockdown. Phagocytic rates of hemocytes were calculated according to the formula presented in the Materials and Methods section. Three independent repeats were performed, and results are expressed as the mean ± SD. Data were analyzed by one-way ANOVA and the letters (a, b, c) presented significant differences (p < 0.05).
Membrane-Bound EsDscam Acts as a Phagocytic Receptor for Soluble EsDscam
To evaluate the possible interaction between soluble and membrane-bound forms of EsDscam, colocalization of Es-sDscam and Es-mDscam in hemocytes was investigated by immunohistochemistry. Es-sDscam was found to colocalize with Es-mDscam following coculture with its specific binding bacteria (Figure 8A). Since homotypic interactions may be the basis for the binding of mDscam with sDscam, we speculated that only Es-mDscam with the same alternative spliced exons was able to enhance Es-sDscam-regulated phagocytosis. To test this hypothesis, siRNA targeting exons 4.24 and 6.20 (Figure 8B) were transfected into hemocytes leading to significant silencing of exon 4.24 involved membrane-bound EsDscam (exon 4.24-EsDscam) that corresponding to soluble rEsDscam(4.24, 6.19) (Figure 8C) and exon significant silencing of exon 6.20 involved membrane-bound EsDscam (exon 6.20-EsDscam) that corresponding to soluble rEsDscam(4.12, 6.20) (Figure 8D). Knockdown of the both exons led to significantly reduced phagocytosis (Figures 8E,F). Interestingly, knockdown of exon 4.24-EsDscam significantly reduced rEsDscam(4.24, 6.19)-enhanced phagocytosis, while knockdown of exon 6.20-EsDscam did not (Figure 8E). Furthermore, knockdown of exon 6.20-EsDscam significantly reduced rEsDscam(4.12, 6.20)-enhanced phagocytosis, while knockdown of exon 4.24-EsDscam did not (Figure 8F). The interaction of Es-sDscam and Es-mDscam was confirmed in vitro and in vivo by analysis of myc-tagged forms of the rEsDscam(4.24,6.19) and rEsDscam(4.12,6.20) proteins using far-Western blot (Figure 8G) and coimmunoprecipitation (Figure 8H), which revealed that Es-sDscam and Es-mDscam interactions occur only between the same isoforms. These results suggest that Es-mDscam functions as a phagocytic receptor for Es-sDscam via homotypic interactions.
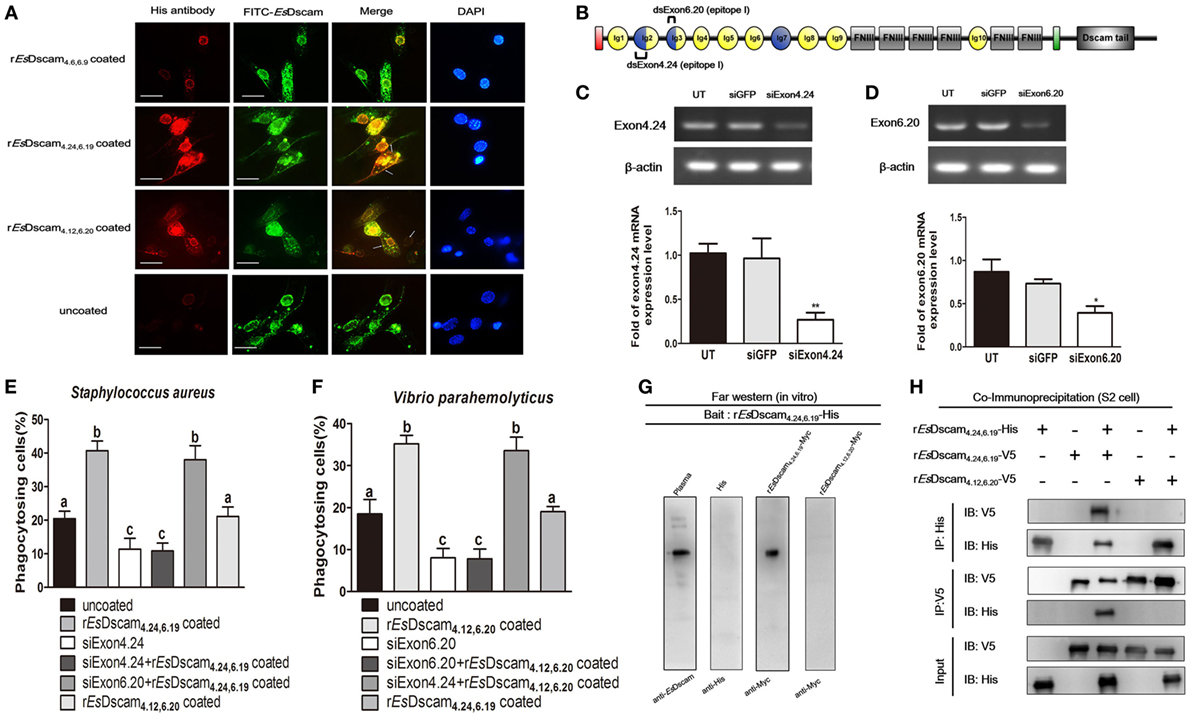
Figure 8. Interaction of membrane-bound and soluble EsDscam is essential for promotion of phagocytosis. (A) Colocalization of Es-mDscam and Es-sDscam in hemocyte surface. Each group that including rEsDscam4.6,6.9, Staphylococcus aureus coated rEsDscam4.24,6.19, and Vibrio parahemolyticus coated rEsDscam4.12,6.20 was used to stimulate cultured crab hemocyte. Hemocytes were collected 1 h later and subjected to immunocytochemistry detected by the antibodies of EsDscam (membrane-bound EsDscam) and His-tag (soluble EsDscam). Scale bar = 15 µm. Data are representative of two independent repeats. (B) Schematic diagram showing the RNAi region recognized by siExon 4.24 and siExon 6.20 primers in the epitope I region. (C,D) Knockdown of exon 4.24 and exon 6.20 expression. Crab hemocyte were transfected with 5 nM exon 4.24 siRNA (left panel) or exon 6.20 siRNA (right panel), with equal amounts of GFP siRNA served as a control. RNA samples were collected at 24 h post-transfection to check the silencing efficiency by RT-PCR with β-actin as a reference. RT-PCR data are representative of two independent repeats. At least three crab were used for each sample, and qRT-PCR were performed three times. The results are expressed as the mean ± SD, data were analyzed by Student’s t-test. **p < 0.01, *p < 0.05. (E,F) Specific membrane-bound EsDscam regulate soluble EsDscam promoted phagocytosis in hemocyte. Rate of hemocyte phagocytosis of (E) Staphylococcus aureus and (F) V. parahemolyticus following knockdown of exon 4.24 and exon 6.20, respectively. Phagocytic rates of hemocytes were calculated according to the formula presented in Section “Materials and Methods.” At least 15 fields were included and data represent the mean ± SD of three independent experiments. The results were analyzed statistically using one-way ANOVA and the letters (a, b, c) presented significant differences (p < 0.05). (G) Interaction between membrane-bound and soluble EsDscam in vitro. The bait protein rEsDscam4.24,6.19-His was first separated by SDS-PAGE, transferred onto a nitrocellulose membrane, and renatured by adding a series of different concentration of guanidine-HCl. The undetected proteins were added to recognize the bait protein on the membrane before the attached proteins were detected by Western blotting using the specific antibodies. Data are representative of two independent repeats. (H) Interaction between membrane-bound and soluble EsDscam in vivo. Lysates from S2 cells transiently transfected with pAc5.1-rEsDscam4.24,6.19-His, together with pAc5.1-rEsDscam4.12,6.20-V5 or pAc5.1-rEsDscam4.24,6.19-V5, were subjected to immunoprecipitation with anti-His Ab or anti-V5 Ab, followed by Western blot analysis with anti-His Ab and anti-V5 Ab. The input controls were also analyzed by Western blot. Data are representative of two independent repeats.
Discussion
A vast repertoire of immune receptors is required to provide adaptive immune defense against an equally vast and rapidly evolving pool of potential pathogens. Antigen receptor diversity in mammals is generated by somatic gene rearrangement and increased in B cells by somatic hyper-mutation. By contrast, these processes are absent in arthropods (46) and the mechanisms responsible for generation of the required receptor repertoire in these species remain to be elucidated. Interestingly, the recent discovery of the role of Dscam in host defense in insects (13, 15, 16) and shrimp (19, 44) has provided important insights.
The extreme diversity of the proteins encoded by Dscam genes is almost unique (47) and despite the consistency of multiple variants within the three exon clusters across all crustaceans, these variants are not conserved across species (19). In species other than D. melanogaster, the numbering of orthologous exon clusters varies due to differences in the positions of exon–exon boundaries (16). Mutually exclusive alternative splicing of Dscam pre-mRNA ensures that each mRNA contains only one of the possible variants from each of the three alternative exon clusters (14, 48). Across species, these alternatively spliced exons encode the N-terminal regions of the Ig2 and Ig3 domains and the entire Ig7 domain. These domains are located in the extracellular portion of the protein, with the potential to produce more than 10,000 splice forms from a single gene. However, there are major differences in types of alternative splicing from chelicerates to pancrustaceans, even within pancrustaceans there could be room for differences in the mode of expression resulting in modulations of Dscam role in immunity (49).
The discovery of the role of Dscam in insect immunity may obscures the classical strict clarification between innate and adaptive immunity (13, 16, 40, 50). Accumulating evidence implicates Dscam might be involved in immunity against non-self-molecules in long-lived crustaceans, such as crab and shrimp. Dscam shows a typically rapid non-specific immune response to pathogen-associated molecular patterns such as LPS and beta-1,3-glucan (43, 44). However, in contrast to most innate immune factors, the induction of Dscam is not always an immediate response to stimulation by pathogens including viruses and bacteria (20, 27, 51). In this study, heat-inactivated bacteria were used for most of the experiment due to previously reported methods (16, 25, 32) and its low toxicity on primary cultured hemocyte, and we think Dscam will respond to live pathogens in the same manner since heat-killed bacteria still retain the key components and the bacterial clearance assay by using live bacteria confirm the function of Dscam on bacteria binding and phagocytosis promotion. Our study indicates that the role of Dscam in immunity is dictated not only by overall expression levels but also by the combination of Dscam isoforms. We found that some of the pathogen-induced Dscam isoforms that were highly induced after challenge with a particular pathogen showed significantly greater and more specific binding ability to that same pathogen, as well as efficient bacterial clearance. Although diversification is clearly critical for the generation of a sufficiently large pathogen receptor repertoire to allow discrimination among an equally large number of potential antigens, evidence that this level of specificity exists in pancrustacean immunity is limited (52). However, due to its extreme variability, the Dscam gene represents the only known system that could, at least theoretically, provide the required receptor diversity in pancrustaceans.
The topology of the eight Ig domains at the N-terminal of Dscam in D. melanogaster (21) has been elucidated by negative-staining electron microscopy. Averaged images of several isoforms obtained using this technique revealed multiple distinct configurations. By contrast, class averages of the N-terminal four Ig domains in the proteins revealed a horseshoe shape. Moreover, hemocytes of immunologically challenged D. melanogaster (13) and Litopenaeus vannamei (31) exhibited higher variability in the Ig2 and Ig3 domains of Dscam compared with those in the untreated controls, while few Ig7 variants were detected. For these reasons, we produced recombinant proteins covering only the Ig1–Ig4 region to study the immunological functions of EsDscam. Interestingly, we found bacteria-specific binding activity is essential for Ig1–Ig4 domain-associated promotion of phagocytosis by soluble EsDscam. Furthermore, although truncated recombinant EsDscam proteins retained the ability to bind bacteria, the capacity to promote phagocytosis was abolished, possibly due to the absence of the region required for interaction with the phagocytic receptor on the hemocyte surface. Further investigations showed that RNAi-mediated knockdown of membrane-bound EsDscam in hemocytes resulted in significant inhibition of soluble EsDscam-mediated phagocytosis, which suggests a possible interaction between these two EsDscam protein forms.
Importantly, parts of the Ig2 and Ig3 domains together form two surface epitopes (I and II) at either side of the conserved horseshoe structure, which are encoded partly by exon cluster 4 and partly by exon cluster 6 (21, 23). Epitope I is located on the composite surface that crucial for Dscam isoform interactions, while epitope II is located on the opposite face with the potential to interact with antigens. To study the possible interaction between membrane-bound and soluble EsDscam, membrane-bound EsDscam that share the same alternatively spliced exon as the soluble EsDscam were knocked-down, and results revealed that the ability of membrane-bound EsDscam to regulate the promoted phagocytosis by soluble EsDscam was strictly alternatively spliced exon-specific. Furthermore, membrane-bound EsDscam was found to be colocalized with soluble EsDscam, and binding between Dscam isoforms was observed only in the same protein.
In conclusion, our study showed that EsDscam is upregulated following bacterial challenge, and bacteria-specific EsDscam isoforms are produced via alternative splicing. Subsequently, bacterial-induced specific soluble EsDscam isoforms bind with the original bacteria and enhance phagocytosis by hemocytes via the membrane-bound EsDscam that shares the same extracellular regions with the soluble EsDscam that functions as the phagocytic receptor (Figure 9).
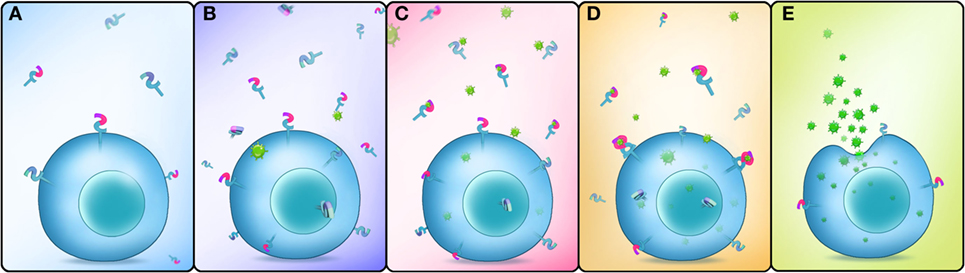
Figure 9. Schematic representation of Dscam-regulated pathogen-specific phagocytosis. (A) Lower concentrations of membrane-bound and soluble EsDscam in normal hemocytes and cell-free hemolymph. (B) The expression of membrane-bound and soluble EsDscam (including bacteria-specific induced and non-specific induced EsDscam isoforms) is highly induced after bacterial infection. (C) Bacteria-specific induced soluble EsDscam isoforms bind with high affinity to the original bacteria, while the other soluble isoforms show weak or no binding with the bacteria. (D) Bacteria-specific binding soluble EsDscam isoforms interact with the membrane-bound EsDscam containing the same extracellular region. (E) EsDscam regulates pathogen-specific phagocytosis in crab hemocyte.
Ethics Statement
This study was carried out in accordance with the recommendations of Ministry of Science and Technology of the People’s Republic of China on animal care guidelines. The protocol was approved by East China Normal University Animal Care and Use Committee (Protocol license number: AR2012/12017).
Author Contributions
Conceived and designed the experiments: W-WL, QW, and X-JL. Performed the experiments: X-JL, LY, DL, and Y-TZ. Analyzed the data and wrote the paper: X-JL, QW, and W-WL.
Conflict of Interest Statement
The authors declare that the research was conducted in the absence of any commercial or financial relationships that could be construed as a potential conflict of interest.
Acknowledgments
We thank the National Pathogen Collection Center for Aquatic Animals (Shanghai Ocean University, Shanghai, China) for providing bacteria strains and Prof. Erjun Ling (from Chinese Academy of Sciences) for assistance with S2 cells cultures.
Funding
This work was supported by the National Natural Science Foundation of China (Grant No. 31602189 and 31672639).
Supplementary Material
The Supplementary Material for this article can be found online at https://www.frontiersin.org/articles/10.3389/fimmu.2018.00801/full#supplementary-material.
Figure S1. The 3D structure of the EsDscam protein (N-terminal Ig1–Ig8) and the location of epitopes I and II of the horseshoe configuration. (A) The 3D structure of the EsDscam protein (N-terminal Ig1–Ig8). A 3D homology model of EsDscam was generated using SWISS-MODEL (http://swissmodel.expasy.org/) (53) based on the crystal structure of Dscam from Drosophila (PDB ID: 3dmk.1.A) as a template (22). The 3D structure of EsDscam was then visualized using the PyMOL molecular graphics system (54). The Ig domains are shown in different colors. (B) Structural comparison of modeled EsDscam and Drosophila melanogaster Dscam. EsDscam (red); D. melanogaster Dscam (green). (C) Location of epitopes I and II; epitope I of exon 4 (yellow); epitope II of exon 4 (red); epitope I of exon 6 (purple); epitope II of exon 6 (green). (D) Sequences of the 11 peptides used in the bacteria-binding assay. (E) The similarity and classification of exon cluster 4 and exon cluster 6. (Left panel) Exon cluster 4 was divided artificially into five groups, (right panel) exon cluster 6 was divided artificially into six groups.
Figure S2. Preparation of specific anti-EsDscam antibody and loading control for various tissues. (A) Agarose gel electrophoresis (1% w/v) of the FNIII3–FNIII6 region of EsDscam. Lane M1: 100–2,000 bp DNA maker. Lane 1: 1,455 bp cDNA amplification product. Lane 2: double digestion of recombinant plasmid pMD19-T–FNIII3–FNIII6 with EcoRI and XhoI restriction enzymes. Lane 3: double digestion of plasmid pET-28a with EcoRI and XhoI restriction enzymes. Lane 4: the PCR product of recombinant plasmid pET-28a–FNIII3–FNIII6. (B) Analysis of the recombinant EsDscamFNIII3–FNIII6 protein expression. Lane M2: 12–120 kDa protein markers. Lanes 1–4: recombinant protein expression under different induction conditions: 1 mM IPTG at 37°C; 1 mM IPTG at 30°C; 0.25 mM IPTG at 37°C, and 0.25 mM IPTG at 30°C, respectively. The arrow indicates the target protein. Lane 5: total proteins from uninduced cells. Lane 6: total proteins from induced cells harboring pET-28a (control). The molecular weight of induced protein is 62 kDa. (C) Purification of the recombinant EsDscamFNIII3–FNIII6 protein. Lane M3: 17–245 kDa protein markers. Lane 7: supernatant containing induced pET-28a–FNIII3–FNIII6. Lane 8: flow-through eluate of the supernatant. Lanes 9–17: protein purified from eluates containing different concentrations of imidazole. (D) EsDscam antibody titers; preimmune serum was used as the control. (E) Western blot analysis of the specific binding of the purified recombinant protein with antiserum. Lanes 1–3: 10, 8, and 2 µg. (F) Protein loading control. (G)The specificity for detecting His antibodies used in immunofluorescence. Lane M: Protein marker. Lane 1: The total protein of hemocytes.
Figure S3. Exon 4 and exon 6 partitions of epitope I (magenta) and epitope II (cyan) in Eriocheir sinensis. In exon cluster 4, the 12 amino acids between the conserved 7Q and 18V were considered to belong to epitope I, and the 18 amino acids (approximately) after 43W were considered to belong to epitope II. In exon cluster 6, the eight amino acids after 15R were considered to belong to epitope I, and the eight amino acids before the conserved LLC motif were considered to belong to epitope II.
Figure S4. The expression and purification of rEsDscam isoforms (Ig1–Ig4) and truncated rEsDscam4.24,6.19 protein. (A,B) Recombinant expression plasmids: pET-28a-rEsDscam4.24,6.19, pET-28a-rEsDscam4.12,6.20, pET-28a-rEsDscam4.6,6.9, pET-28a-rIg1-2, pET-28a-rIg2-3, and pET-28a-rIg3-4. The constructs were generated using the protocols described in Figure S2 in Supplementary Material. (C,D) Recombinant proteins expression and purification. (C) Lanes 1–4: recombinant protein expression under different induction conditions: 1 mM IPTG at 37°C; 1 mM IPTG at 30°C; 0.25 mM IPTG at 37°C, and 0.25 mM IPTG at 30°C, respectively. Lane 5: supernatant of induced pET-28a-rEsDscams. Lane 6: precipitation of induced pET-28a-rEsDscams. Lane 7: precipitation of induced pET-28a-rEsDscams. Lane 8: flow-through eluate of the precipitate. Lanes 9–18: protein purified from eluates containing different concentrations of imidazole. (D) Lane 1: total proteins from uninduced cells. Lanes 2–5: recombinant protein expression under different induction conditions: 1 mM IPTG at 37°C; 1 mM IPTG at 30°C; 0.25 mM IPTG at 37°C, and 0.25 mM IPTG at 30°C, respectively. Lane 6: precipitation of induced proteins. Lane 7: induced proteins in culture supernatants. Lane 8: induced proteins in culture supernatants. Lane 9: flow-through eluate of the supernatant. Lanes 10–19: protein purified from eluates containing different concentrations of imidazole.
Footnotes
- ^http://bioinf.cs.ucl.ac.uk/psipred/ (Accessed: April 16, 2015).
- ^http://weblogo.berkeley.edu/ (Accessed: May 12, 2015).
References
1. Azumi K, De Santis R, De Tomaso A, Rigoutsos I, Yoshizaki F, Pinto MR, et al. Genomic analysis of immunity in a Urochordate and the emergence of the vertebrate immune system: “waiting for Godot”. Immunogenetics (2003) 55(8):570–81. doi:10.1007/s00251-003-0606-5
2. Du Pasquier L. Speculations on the origin of the vertebrate immune system. Immunol Lett (2004) 92(1):3–9. doi:10.1016/j.imlet.2003.10.012
3. Guo P, Hirano M, Herrin BR, Li JX, Yu CL, Sadlonova A, et al. Dual nature of the adaptive immune system in lampreys. Nature (2009) 459:796–801. doi:10.1038/nature08068
4. Cooper D, Eleftherianos I. Memory and specificity in the insect immune system: current perspectives and future challenges. Front Immunol (2017) 8:539. doi:10.3389/fimmu.2017.00539
5. Kurtz J, Franz K. Innate defence: evidence for memory in invertebrate immunity. Nature (2003) 425:37–8. doi:10.1038/425037a
6. Little TJ, O’Connor B, Colegrave N, Watt K, Read AF. Maternal transfer of strain-specific immunity in an invertebrate. Curr Biol (2003) 13(6):489–92. doi:10.1016/S0960-9822(03)00163-5
7. Sadd BM, Kleinlogel Y, Schmid-Hempel R, Schmid-Hempel P. Trans-generational immune priming in a social insect. Biol Lett (2005) 1(4):386–8. doi:10.1098/rsbl.2005.0369
8. Sadd BM, Schmid-Hempel P. Facultative but persistent trans-generational immunity via the mother’s eggs in bumblebees. Curr Biol (2007) 17(24):R1046–7. doi:10.1016/j.cub.2007.11.007
9. Freitak D, Schmidtberg H, Dickel F, Lochnit G, Vogel H, Vilcinskas A. The maternal transfer of bacteria can mediate trans-generational immune priming in insects. Virulence (2014) 5(4):547–54. doi:10.4161/viru.28367
10. Salmela H, Amdam GV, Freitak D. Transfer of immunity from mother to offspring is mediated via egg-yolk protein vitellogenin. PLoS Pathog (2015) 11(7):e1005015. doi:10.1371/journal.ppat.1005015
11. Pham LN, Dionne MS, Shirasu-Hiza M, Schneider DS. A specific primed immune response in Drosophila is dependent on phagocytes. PLoS Pathog (2007) 3(3):e26. doi:10.1371/journal.ppat.0030026
12. Vogel C, Teichmann SA, Chothia C. The immunoglobulin superfamily in Drosophila melanogaster and Caenorhabditis elegans and the evolution of complexity. Development (2003) 130(25):6317. doi:10.1242/dev.00848
13. Watson FR, Püttmann-Holgado R, Thomas F, Lamar DL, Hughes M, Kondo M, et al. Extensive diversity of Ig-superfamily proteins in the immune system of insects. Science (2005) 309(5742):1874–8. doi:10.1126/science.1116887
14. Schmucker D, Clemens JC, Shu H, Worby CA, Xiao J, Muda M, et al. Drosophila Dscam is an axon guidance receptor exhibiting extraordinary molecular diversity. Cell (2000) 101(6):671–84. doi:10.1016/S0092-8674(00)80878-8
15. Dong Y, Cirimotich CM, Pike A, Chandra R, Dimopoulos G. Anopheles NF-κB-regulated splicing factors direct pathogen-specific repertoires of the hypervariable pattern recognition receptor AgDscam. Cell Host Microbe (2012) 12(4):521–30. doi:10.1016/j.chom.2012.09.004
16. Dong Y, Taylor HE, Dimopoulos G. AgDscam, a hypervariable immunoglobulin domain-containing receptor of the Anopheles gambiae innate immune system. PLoS Biol (2006) 4(7):e229. doi:10.1371/journal.pbio.0040229
17. Peuß R, Wensing KU, Woestmann L, Eggert H, Milutinovic B, Sroka MG, et al. Down syndrome cell adhesion molecule 1: testing for a role in insect immunity, behaviour and reproduction. R Soc Open Sci (2016) 3(4):160138. doi:10.1098/rsos.160138
18. Chou PH, Chang HS, Chen IT, Lee CW, Hung HY, Wang HC. Penaeus monodon Dscam (PmDscam) has a highly diverse cytoplasmic tail and is the first membrane-bound shrimp Dscam to be reported. Fish Shellfish Immunol (2011) 30(4–5):1109–23. doi:10.1016/j.fsi.2011.02.009
19. Ng TH, Chiang YA, Yeh YC, Wang HC. Review of Dscam-mediated immunity in shrimp and other arthropods. Dev Comp Immunol (2015) 48:306–14. doi:10.1016/j.dci.2014.07.017
20. Ng TH, Hung HY, Chiang YA, Lin JH, Chen YN, Chuang YC, et al. WSSV-induced crayfish Dscam shows durable immune behavior. Fish Shellfish Immunol (2014) 40(1):78–90. doi:10.1016/j.fsi.2014.06.023
21. Meijers R, Puettmann-Holgado R, Skiniotis G, Liu JH, Walz T, Wang JH, et al. Structural basis of Dscam isoform specificity. Nature (2007) 449(7161):487–91. doi:10.1038/nature06147
22. Sawaya MR, Wojtowicz WM, Andre I, Qian B, Wu W, Baker D, et al. A double S shape provides the structural basis for the extraordinary binding specificity of Dscam isoforms. Cell (2008) 134(6):1007–18. doi:10.1016/j.cell.2008.07.042
23. Brites D, Encinas-Viso F, Ebert D, Du Pasquier L, Haag CR. Population genetics of duplicated alternatively spliced exons of the Dscam gene in Daphnia and Drosophila. PLoS One (2011) 6(12):e27947. doi:10.1371/journal.pone.0027947
24. McGuffin LJ, Bryson K, Jones DT. The PSIPRED protein structure prediction server. Bioinformatics (2000) 16(4):404–5. doi:10.1093/bioinformatics/16.4.404
25. Zhu YT, Jin XK, Fang ZY, Zhang X, Li D, Li WW, et al. A novel Eriocheir sinensis primary hemocyte culture technique and its immunoreactivity after pathogen stimulation. Aquaculture (2015) 446:140–7. doi:10.1016/j.aquaculture.2015.04.032
26. Huss HH. Control of indigenous pathogenic bacteria in seafood. Food Control (1997) 8(2):91–8. doi:10.1016/S0956-7135(96)00079-5
27. Hung HY, Ng TH, Lin JH, Chiang YA, Chuang YC, Wang HC. Properties of Litopenaeus vannamei Dscam (LvDscam) isoforms related to specific pathogen recognition. Fish Shellfish Immunol (2013) 35(4):1272–81. doi:10.1016/j.fsi.2013.07.045
28. Li X, Yao F, Zhang W, Cheng C, Chu B, Liu Y, et al. Identification, expression pattern, cellular location and potential role of the caveolin-1 gene from Artemia sinica. Gene (2014) 540(2):161–70. doi:10.1016/j.gene.2014.02.055
29. Reichelt P, Schwarz C, Donzeau M. Single step protocol to purify recombinant proteins with low endotoxin contents. Protein Expr Purif (2006) 46(2):483–8. doi:10.1016/j.pep.2005.09.027
30. Livak KJ, Schmittgen TD. Analysis of relative gene expression data using real-time quantitative PCR and the 2-ΔΔCT method. Methods (2001) 25(4):402–8. doi:10.1006/meth.2001.1262
31. Chou PH, Chang HS, Chen IT, Lin HY, Chen YM, Yang HL, et al. The putative invertebrate adaptive immune protein Litopenaeus vannamei Dscam (LvDscam) is the first reported Dscam to lack a transmembrane domain and cytoplasmic tail. Dev Comp Immunol (2009) 33(12):1258–67. doi:10.1016/j.dci.2009.07.006
32. Wang XW, Zhao XF, Wang JX. C-type lectin binds to beta-integrin to promote hemocytic phagocytosis in an invertebrate. J Biol Chem (2014) 289(4):2405–14. doi:10.1074/jbc.M113.528885
33. Rämet M, Pearson A, Manfruelli P, Li X, Koziel H, Göbel V, et al. Drosophila scavenger receptor CI is a pattern recognition receptor for bacteria. Immunity (2001) 15(6):1027–38. doi:10.1016/S1074-7613(01)00249-7
34. Zhang XW, Wang Y, Wang XW, Wang L, Mu Y, Wang JX. A C-type lectin with an immunoglobulin-like domain promotes phagocytosis of hemocytes in crayfish Procambarus clarkii. Sci Rep (2016) 6:29924. doi:10.1038/srep29924
35. Bao W, Kumagai Y, Niu H, Yamaguchi M, Miura K, Rikihisa Y. Four VirB6 paralogs and VirB9 are expressed and interact in Ehrlichia chaffeensis-containing vacuoles. J Bacteriol (2009) 191(1):278–86. doi:10.1128/JB.01031-08
36. Wang XW, Gao J, Xu YH, Xu JD, Fan ZX, Zhao XF, et al. Novel pattern recognition receptor protects shrimp by preventing bacterial colonization and promoting phagocytosis. J Immunol (2017) 198(8):3045–57. doi:10.4049/jimmunol.1602002
37. Li C, Chen Y, Weng S, Li S, Zuo H, Yu X, et al. Presence of tube isoforms in Litopenaeus vannamei suggests various regulatory patterns of signal transduction in invertebrate NF-κB pathway. Dev Comp Immunol (2014) 42(2):174–85. doi:10.1016/j.dci.2013.08.012
38. Wang PH, Wan DH, Gu ZH, Deng XX, Weng SP, Yu XQ, et al. Litopenaeus vannamei tumor necrosis factor receptor-associated factor 6 (TRAF6) responds to Vibrio alginolyticus and white spot syndrome virus (WSSV) infection and activates antimicrobial peptide genes. Dev Comp Immunol (2011) 35(1):105. doi:10.1016/j.dci.2010.08.013
39. Zuo H, Yuan J, Chen Y, Li S, Su Z, Wei E, et al. A microRNA-mediated positive feedback regulatory loop of the NF-κB pathway in Litopenaeus vannamei. J Immunol (2016) 196(9):3842. doi:10.4049/jimmunol.1502358
40. Brites D, McTaggart S, Morris K, Anderson J, Thomas K, Colson I, et al. The Dscam homologue of the crustacean Daphnia is diversified by alternative splicing like in insects. Mol Biol Evol (2008) 25(7):1429–39. doi:10.1093/molbev/msn087
41. Holt RA, Subramanian GM, Halpern A, Sutton GG, Charlab R, Nusskern DR, et al. The genome sequence of the malaria mosquito Anopheles gambiae. Science (2002) 298:129. doi:10.1126/science.1076181
42. Graveley BR, Kaur A, Gunning D, Zipursky SL, Rowen L, Clemens JC. The organization and evolution of the dipteran and hymenopteran Down syndrome cell adhesion molecule (Dscam) genes. RNA (2004) 10(10):1499–506. doi:10.1261/rna.7105504
43. Jin XK, Li WW, Wu MH, Guo XN, Li S, Yu AQ, et al. Immunoglobulin superfamily protein Dscam exhibited molecular diversity by alternative splicing in hemocytes of crustacean, Eriocheir sinensis. Fish Shellfish Immunol (2013) 35(3):900–9. doi:10.1016/j.fsi.2013.06.029
44. Li D, Yu AQ, Li XJ, Zhu YT, Jin XK, Li WW, et al. Antimicrobial activity of a novel hypervariable immunoglobulin domain-containing receptor Dscam in Cherax quadricarinatus. Fish Shellfish Immunol (2015) 47(2):766–76. doi:10.1016/j.fsi.2015.10.025
45. Du Pasquier L. Immunology. Insects diversify one molecule to serve two systems. Science (2005) 309(5742):1826–7. doi:10.1126/science.1118828
46. Stuart LM, Ezekowitz RA. Phagocytosis and comparative innate immunity: learning on the fly. Nat Rev Immunol (2008) 8(2):131–41. doi:10.1038/nri2240
47. Amitage SA, Kurtz J, Brites D, Dong Y, Du Pasquier L, Wang HC. Dscam1 in pancrustacean immunity: current status and a look to the future. Front Immunol (2017) 8:662. doi:10.3389/fimmu.2017.00662
48. Yang Y, Zhan L, Zhang W, Sun F, Wang W, Tian N, et al. RNA secondary structure in mutually exclusive splicing. Nat Struct Mol Biol (2011) 18(2):159–68. doi:10.1038/nsmb.1959
49. Yuan Y, Meng Y, Ma H, Hou S, Cao G, Hong W, et al. A large family of Dscam genes with tandemly arrayed 5′ cassettes in Chelicerata. Nat Commun (2016) 7:11252. doi:10.1038/ncomms11252
50. Milutinovic B, Peuss R, Ferro K, Kurtz J. Immune priming in arthropods: an update focusing on the red flour beetle. Zoology (Jena) (2016) 119(4):254–61. doi:10.1016/j.zool.2016.03.006
51. Chiang YA, Hung HY, Lee CW, Huang YT, Wang HC. Shrimp Dscam and its cytoplasmic tail splicing activator serine/arginine (SR)-rich protein B52 were both induced after white spot syndrome virus challenge. Fish Shellfish Immunol (2013) 34(1):209–19. doi:10.1016/j.fsi.2012.10.021
52. Armitage SA, Peuß R, Kurtz J. Dscam and pancrustacean immune memory—a review of the evidence. Dev Comp Immunol (2015) 48(2):315–23. doi:10.1016/j.dci.2014.03.004
53. Biasini M, Bienert S, Waterhouse A, Arnold K, Studer G, Schmidt T, et al. SWISS-MODEL: modelling protein tertiary and quaternary structure using evolutionary information. Nucleic Acids Res (2014) 42(Web Server issue):W252–8. doi:10.1093/nar/gku340
Keywords: membrane-bound Down syndrome cell adhesion molecule, soluble Down syndrome cell adhesion molecule, specific-binding, pathogen, phagocytosis, Eriocheir sinensis
Citation: Li X-J, Yang L, Li D, Zhu Y-T, Wang Q and Li W-W (2018) Pathogen-Specific Binding Soluble Down Syndrome Cell Adhesion Molecule (Dscam) Regulates Phagocytosis via Membrane-Bound Dscam in Crab. Front. Immunol. 9:801. doi: 10.3389/fimmu.2018.00801
Received: 10 January 2018; Accepted: 03 April 2018;
Published: 18 April 2018
Edited by:
Miki Nakao, Kyushu University, JapanReviewed by:
Hai-Peng Liu, Xiamen University, ChinaIkuo Hirono, Tokyo University of Marine Science and Technology, Japan
Copyright: © 2018 Li, Yang, Li, Zhu, Wang and Li. This is an open-access article distributed under the terms of the Creative Commons Attribution License (CC BY). The use, distribution or reproduction in other forums is permitted, provided the original author(s) and the copyright owner are credited and that the original publication in this journal is cited, in accordance with accepted academic practice. No use, distribution or reproduction is permitted which does not comply with these terms.
*Correspondence: Qun Wang, qwang@bio.ecnu.edu.cn;
Wei-Wei Li, wwli@bio.ecnu.edu.cn
†Present address: You-Ting Zhu, Laboratory of Fish Immunology and Disease Control, College of Fisheries and Life Science, Shanghai Ocean University, Shanghai, China