Photo-Protection in the Centric Diatom Coscinodiscus granii is Not Controlled by Chloroplast High-Light Avoidance Movement
- 1Marine Biological Section, Department of Biology, University of Copenhagen, Helsingør, Denmark
- 2Plant Functional Biology and Climate Change Cluster, University of Technology Sydney, Sydney, Australia
Diatoms are important phototrophs in the worlds' oceans contributing ~40% of the global primary photosynthetic production. This is partially explained by their capacity to exploit environments with variable light conditions, but there is limited knowledge on how diatoms cope with changes in the spectral composition and intensity of light. In this study, the influence of light quality and high irradiance on photosynthesis in the centric diatom Coscinodiscus granii was investigated with microscopic imaging and variable chlorophyll fluorescence techniques. Determination of the wavelength-dependent functional absorption cross-section of photosystem (PS) II revealed that absorption of blue light (BL) and red light (RL) was 2.3- and 0.8-fold that of white light (WL), respectively. Hence, BL was more efficiently converted into photo-chemical energy. Excessive energy from BL was dissipated via non-photochemical quenching (NPQ) mechanisms, while RL apparently induced only negligible NPQ even at high irradiance. A dose dependent increase of cells exhibiting an altered chloroplast distribution was observed after exposure to high levels of BL and WL, but not RL. However, no effective quantum yield of PSII was measured in the majority of cells with an altered chloroplast distribution, and positive Sytox green® death staining confirmed that most of these cells were dead. We conclude that although C. granii can sustain high irradiance it does not perform chloroplast high-light avoidance movements for photo-protection.
Introduction
Diatoms are responsible for about 40% of the primary production in marine ecosystems and account for up to 20% of global carbon fixation (Nelson and Brzezinski, 1997; Geider et al., 2001). The evolutionary success and high productivity of diatoms (Boyd et al., 2000; Thomas and Dieckmann, 2002; Mock and Valentin, 2004) seem to be closely related to their ability to adapt to environmental fluctuations such as changes in irradiance and spectral composition of the light field (Depauw et al., 2012). The in situ solar irradiance in aquatic systems can be affected on different time scales by various factors such as vertical mixing, wave focusing, and varying cloud cover. Vertical shifts in the water column change the blue light (BL) to red light (RL) ratio, where RL is more strongly absorbed by seawater, while BL penetrates deeper into the water column in the absence of large amounts of dissolved organic carbon and particles (Kirk, 1994). A unique feature of diatoms is the encasement of the cell in a silicate frustule composed of two overlapping sections known as thecae or valves (Schmid and Volcani, 1983). The frustule exhibits special optical properties and this has led to speculations that the frustule nanostructure could affect the photobiology of diatoms (Sumper and Brunner, 2006; De Stefano et al., 2007; Parker and Townley, 2007; Su et al., 2015) and thus potentially modulate their photosynthetic efficiency.
If the photosynthetic antennae absorb too much light, the excessive energy load can cause severe damages to the photosynthetic system, e.g., when electrons are transferred to other acceptors such as O2 causing formation of reactive oxygen species (ROS; Apel and Hirt, 2004). There are two main strategies for phototrophic organisms to deal with excessive light energy (Demming-Adams and Adams, 1992). The first strategy involves mechanisms to dissipate excessive energy by activation of alternative electron sinks such as photorespiration, and by photo-protective mechanisms such as the diadinoxanthin (Ddx) cycle responsible for the remarkable potential of diatoms to release excessive energy as heat (Schumann et al., 2007; Goss and Jakob, 2010). The second strategy involves avoidance mechanisms in order to reduce the harvesting of incident light that cannot be used for photosynthesis. On the long term, such avoidance can be achieved by changes in the pigmentation (e.g., Kiefer, 1973), increased light reflection (e.g., Kasperbauer, 1987), or by reduction of the light harvesting antenna complex (Demming-Adams and Adams, 1992). On the short term, avoidance can also be achieved by light-induced chloroplast re-allocation in the cell (Haupt, 1973; Kasahara et al., 2002). While photo-protection mechanisms are typically controlled by substrate and product feedbacks or redox switches (Schellenberger Costa et al., 2013), light-induced movement is often triggered by photoreceptors (Strasser et al., 2010).
High-light avoidance movement of chloroplasts is primarily induced by BL and high irradiance, while back movement to a more evenly chloroplast distribution in the cell is mediated by far RL (>710 nm) and under low irradiance (e.g., DeBlasio et al., 2003; Gabryś, 2004). Reduction of light exposure through chloroplast high-light avoidance movement can minimize photo-damage and optimize photosynthetic performance (Park et al., 1996; Kasahara et al., 2002; Wada et al., 2003). Chloroplast high-light avoidance movement occurs in higher plants, mosses, ferns, green algae, and dinophytes. It has also been observed in the pennate diatoms Biddulphia pellucida and Seminavis robusta (Gillard et al., 2008), as well as in centric diatom species such as Ditylum blightwellii, Pleurosira laevis, Odontella regia, and Lauderia borealis (Kiefer, 1973; Chen and Li, 1991; Furukawa et al., 1998). The ability of diatoms to re-arrange chloroplasts in a fluctuating light environment might thus maximize their light use efficiency and contribute to the high productivity of diatoms (Boyd et al., 2000; Thomas and Dieckmann, 2002; Mock and Valentin, 2004).
Members of the Coscinodiscaceae family recently observe an increasing interest in the scientific community due to the optical properties and the industrial applicability of the conspicuous ~50–500 μm wide silica frustule surrounding the Coscinodiscus cell (Jeffryes et al., 2015). Hitherto, most studies have focused on empty frustules and to our knowledge the in vivo light environment and how intact living Coscinodiscus cells absorb, propagate and dissipate light energy as a function of irradiance and spectral composition has not yet been analyzed. In this study, photosynthesis-light response curves, non-photochemical quenching (NPQ), and the wavelength-dependent functional absorption cross-section of PS II [Sigma(II)λ] were determined for blue, red and white actinic light in the centric diatom Coscinodiscus granii using variable chlorophyll fluorescence techniques. Mechanisms involved in the stabilization of the cellular light environment and in photo-protection are discussed with emphasis on the observed alteration of the chloroplast distribution in response to high levels of blue and white light.
Materials and Methods
Cultivation, Growth Conditions, and Experimental Set-Up
Semi-continuous cultures of C. granii strain K-1831 (Scandinavian Culture Collection, Copenhagen, Denmark) were grown at 15°C in L1 medium with silica (250 μM Na2SiO3) in 35 permil sterile filtrated seawater (Guillard and Hargraves, 1993). White light fluorescence light tubes (18W/865, Osram, Munich, Germany) provided illumination with an incident photon irradiance (PAR, 400–700 nm) of 100 μmol photons m−2 s−1 from the bottom of a cultivation table in a 14/10 h day/night cycle. Microalgae were maintained in 50 mL polyethylene flasks (Nunc™ cell culture treated EasYFlasks™, Thermo Scientific, Waltham, United States) without any forced aeration or rotation. If not stated otherwise, experiments were performed on three biological replicates of C. granii in the exponential phase.
PSII Absorption Cross Section and Chlorophyll Fluorescence Rapid Light Curves
To account for differences in the wavelength specific absorption of light in subsequent experiments, the functional absorption cross section of PS(II), Sigma(II)λ, was determined. Sigma(II)λ was further used to calculate absolute electron transport rates as derived from chlorophyll fluorescence rapid light curves. For this, a 1 mL cell culture sample was transferred to a quartz cuvette, which was then placed in the optical unit of a multicolor pulse amplitude modulated (PAM) chlorophyll fluorescence photosynthesis analyzer (MC-PAM, Heinz Walz GmbH, Effeltrich, Germany) with a long pass filter (RG 665, Heinz Walz GmbH, Effeltrich, Germany) attached to the fluorescence detector. The photon irradiance levels of the LED array for specific program settings were calibrated with a spherical micro quantum sensor (US-SQS/WB, Heinz Walz GmbH, Effeltrich, Germany). Values were corrected using a zero offset measurement on L1 medium. A three-fold gain and a four-fold damping were set for all measurements. Non-actinic pulse-amplitude modulated blue (BL), red (RL), or white measuring light was used. During illumination with actinic light and during application of a 0.6 s saturating light pulse, the measuring light frequency was increased to 100 kHz. Blue measuring light and blue saturation pulses were applied during blue actinic light measurements. Red measuring light and red saturating pulses were used when red or white actinic light was used. The fluorescence yield (F) was recorded prior to a saturation pulse analysis yielding the maximum fluorescence yield in dark adapted cells (Fm) or the maximum fluorescence yield under different levels of AL (Fm′). The effective photochemical quantum yield of PS(II) was calculated as: (Genty et al., 1989).
Sigma(II)λ was derived in the fast acquisition mode of the multicolor PAM, using the script-file Sigma100.FTM (Schreiber et al., 2012) followed by a curve fit applied in the system software PamWin3 (Heinz Walz GmbH, Effeltrich, Germany). The change of Sigma(II)λ values for BL and RL in relation to WL were calculated [Sigma(II)λ BL or RL per Sigma(II)λ WL]. Sigma(II)λ values are presented as antenna per nm2 (Schreiber et al., 2012).
Parameters of the light dependent PSII electron transport were derived from chlorophyll fluorescence data during 10 s irradiance steps, i.e., rapid light curves (Wight and Critchley, 1999). The magnetic stirrer in the quartz cuvette was switched off before the saturation pulse was applied. Absolute electron transport rates at PSII, ETR were calculated as . PAR(II) was calculated as PAR(II) = PAR·0.6022·Sigma(II)λ, where 0.6022·1024 is Avogadro's constant with the dimension of mol−1 (Schreiber et al., 2012).
Curve fits on ETR vs. irradiance curves were performed according to Eilers and Peeters (1988), using the Microsoft Excel add-on Solver (Microsoft Corporation, Redmond, USA). The initial slope, alpha, the irradiance level where PS(II) reaches light saturation, Ek, the maximum electron transport rate of PSII, ETRmax, and the irradiance at ETRmax, PARmax, were calculated from curve fits to the Sigma(II)λ corrected data according to Eilers and Peeters (1988).
Light Stress Experiments
Light stress experiments were performed to estimate color- and dose-dependent effects on an alteration of the chloroplast distribution. Inhibitors were used to inhibit cytoskeleton mediated high-light avoidance movement and back-movement toward an even chloroplast distribution (ECD). Light intensities were adjusted on the base of Sigma(II)λ values as described above. Two milliliter of cell culture (500–1000 cells mL−1) were transferred to each well of a tissue culture plate (TC Plate 24 Well, StandardF, Sarstedt, Nümbrecht, Germany). During the light stress experiments, the lid of the cell culture plate was removed. A monochromatic blue LED (473 ± 13 nm), a red LED (636 ± 11 nm), and a white LED (452–647 nm bandwidth) were used to subject the cells to high light stress and induce an altered chloroplast distribution, here defined as when chloroplasts aggregated within the cortical cytoplasm. Empty frustules and broken cells that released their chloroplasts were not considered. The spectral range of the LED arrays was determined with a calibrated spectroradiometer (SpectriLight, International Light Technologies Inc., Peabody, USA).
Using Sigma(II)λ values and PARmax data from ETR vs. irradiance measurements described above, experimental photon irradiance levels were adjusted to 600 μmol photons m−2 s−1 BL, 1500 μmol photons m−2 s−1 RL, and 1250 μmol photons m−2 s−1 WL. In this way, an equal amount of photons entering PS(II) was ensured. Photosynthetic active radiation (PAR) was measured with a spherical irradiance probe connected to a quantum irradiance meter (model ULM 500, Heinz Walz GmbH, Effeltrich, Germany). Except for a control kept in the dark, actinic BL, RL, or WL was applied for 1 h.
Actin-mediated cytoskeleton movement was inhibited by adding 1 μL of 0.25 μM latrunculin A (LAT-A) solution per mL of culture (cat. no. L12370, Thermo Scientific, Waltham, United States), while myosin-mediated cytoskeleton movement was inhibited with 100 μL of 50 mM 2,3-butanedione monoxime (BDM) solution (cat. no. B0753, Sigma-Aldrich, St. Louis, Missouri, United States) per mL of cell culture (Cartaxana et al., 2008). LAT-A and BDM stock solutions were prepared in DMSO and culture medium, respectively. After the experiment, samples were kept in the dark for 20 h to recover.
A dose-dependent alteration of the chloroplast distribution was estimated by increasing BL from 0 to 1000 μmol photons m−2 s−1. Samples were tested after 10, 30, or 60 min of illumination. Chloroplast distribution patterns were determined at 200x magnification using a light microscope (model Axiostar plus FL, Carl Zeiss, Jena, Germany). In each replicate, 50–100 cells were evaluated.
Cell Imaging and Sytox® Green Lethality Stain
Sytox®green lethality stain was used to test the viability of cells with an altered or an evenly distributed chloroplast pattern. Altered chloroplast distribution in C. granii kept in tissue culture plates (TC Plate 24 Well, StandardF, Sarstedt, Nümbrecht, Germany) was induced by exposure to 600 μmol photons m−2 s−1 BL for 1 h. Samples were then stained with 0.5 μM Sytox® green (Invitrogen™, Carlsbad, California) for 15 min in the dark according to Armbrecht et al. (2014), prior to counting under epifluorescence light. Sytox® green stained cells showing an even or altered chloroplast distribution were determined at 200x magnification using an Olympus BX50 epifluorescence microscope.
Single Cell Analysis of Variable Chlorophyll Fluorescence
Variable chlorophyll fluorescence was perform in order to estimate the photosynthetic capacity of cells with an altered or with an even chloroplast distribution, respectively. Single-cell variable chlorophyll fluorescence was investigated with a red-green-blue (RGB) pulse-amplitude-modulated (PAM) chlorophyll fluorescence imaging system (RGB Microscopy-IPAM, Heinz Walz GmbH, Germany) mounted on an epi-fluorescence microscope (model Axiostar plus FL, Carl Zeiss, Jena, Germany). The setup is described in detail by Trampe et al. (2011). Measurements were performed with a 20x objective (Plan-Apochromate, Carl Zeiss GmbH, Germany). Non-actinic blue measuring light was used and the effective PSII quantum yield (YII) was determined with a high intensity saturating BL pulse. The maximum fluorescence yield (Fm) correction factor was set to 1.03, to account for signal losses due to LED heating. The Y(II) values were calculated using the system software (ImagingWin, Heinz Walz GmbH, Germany). For each sample, quantitative analysis of variable chlorophyll fluorescence was performed on 10 cells with evenly distributed chloroplasts and 10 cells with an altered chloroplast distribution. Only up to 5 cells with an altered chloroplast distribution were analyzed in the control group because of lower occurrence.
Statistical Analysis
Significant differences among treatment groups were determined with One- or Two-way analysis of variance (ANOVA) followed by Holm Sidak post-hoc tests. Data were log10-transformed to normality if necessary. The ANOVA results are presented with F and p-values. Post-hoc results are indicated with p-values. Differences at the p < 0.05 level are reported as significant. All statistical tests were carried out with SigmaPlot 11.0 (Systat Software, Inc., Richmond, USA).
Results
Functional PSII Absorption Cross Section and PSII Activity as a Function of Irradiance.
Significant differences in Sigma(II)λ were observed between BL (3.5 ± 0.4 nm2) and both RL (1.3 ± 0.2 nm2; p < 0.001) and WL (1.5 ± 0.1 nm2; p < 0.001), but not between RL and WL (p = 0.291; Table 1). Sigma(II)λ was 2.3 ± 0.4 fold higher in blue light than in white light, while Sigma(II)λ was 0.9 ± 0.2 fold lower in red light than in white light (Table 1). Parameters of rapid light curves derived from chlorophyll fluorescence analysis revealed significantly higher alpha in BL (0.44 ± 0.19 μmol e− μmol−1 photons) compared to RL (0.08 ± 0.02; p = 0.006) and WL (0.06 ± 0.01; p = 0.03; Table 1). A trend of higher ETRmax under BL (41.4 ± 5.2 μmol e− m−2 s−1) as compared to RL (35.1 ± 7.1 μmol e− m−2 s−1) and WL (32.3 ± 5.7 μmol e− m−2 s−1) was not significant (p = 0.543). The light saturation index Ik was significantly different between BL (124.4 μmol photons m−2 s−1) and RL (458.2 μmol photons m−2 s−1; p < 0.001) and between BL and WL (572 ± 70 μmol photons m−2 s−1; p = 0.003), but not between RL and WL (p = 0.149; Table 1). Significant differences in PARmax between BL (552 ± 40 μmol photons m−2 s−1; p = 0.003) and RL (993 ± 71 μmol photons m−2 s−1) and between BL and WL (1145 ± 141 μmol photons m−2 s−1; p = 0.010), but not between RL and WL (p = 0.250) were observed (Table 1). Absolute ETR(II) was higher at BL than at RL and WL (Figure 1A). The dose-dependent increase of NPQ was higher with BL as compared to WL, while RL induced almost no NPQ (Figure 1B).
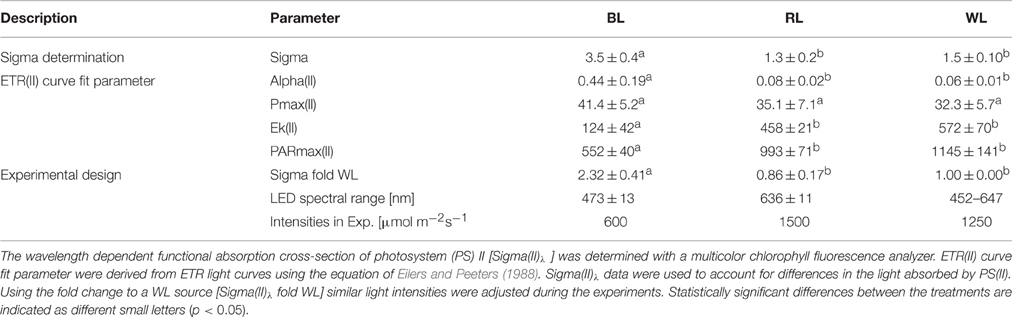
Table 1. Photosynthetic characteristics of Coscinodiscus granii incubated under blue (BL), red (RL), and white (WL) light.
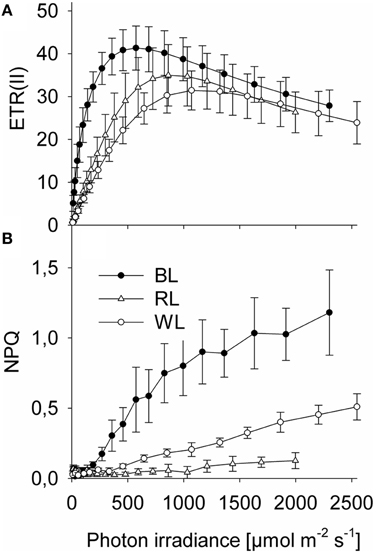
Figure 1. Rapid chlorophyll fluorescence light-response curves of Coscinodiscus granii under blue, red, and white light. (A) Absolute PSII electron transport rates, ETR; (B) Non photochemical quenching, NPQ. Average values and standard error (n = 3) are indicated.
Light Stress Experiments
An altered chloroplast distribution (Figures 2B,D) was observed in 32 ± 5% of cells after 1 h exposure to BL, which was significantly higher than in the control group (5 ± 2%; p < 0.001) (Figure 3A). No significant differences were found between RL-induced altered chloroplast distribution (8 ± 3%; p = 0.289) and the control group. An increase cells with altered chloroplast distribution (to 19 ± 2%) after 1 h exposure to WL was significant (p < 0.001; Figure 3A). No statistical significance was found when comparing the frequency of altered chloroplast distribution after 1 h of high light exposure and after 20 h of recovery in the dark [F(1, 23) = 0.819; p = 0.379; Figure 3A]. The frequency of cells with altered chloroplast distribution in amounted to 22 ± 4% for BL, 9 ± 4% for RL, and 19 ± 15% for WL samples, respectively. The presence of LAT-A did not significantly affect the altered chloroplast distribution as compared to untreated samples [F(1, 23) = 0.793; p = 0.386; Figure 3B]. After 20 h of recovery in the dark, altered chloroplast distribution increased significantly in all samples treated with latrunculin A (LAT-A) [F(1, 23) = 31.825; p < 0.001]. Compared to untreated samples, presence of butanedione monoxime (BDM) significantly increased the altered chloroplast distribution [F(1, 23) = 31.778; p < 0.001; Figure 3C], and in the presence of BDM cells with an altered chloroplast distribution increased further after a 20 h dark recovery period [F(1, 23) = 29.153; p < 0.001].
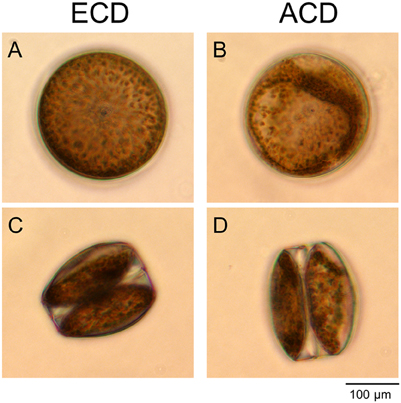
Figure 2. Chloroplast distribution in cells of Coscinodiscus granii. (A,C) Even chloroplast distribution (ECD); (B,D) An altered chloroplast distribution (ACD) can be observed after harmful light doses in some of the cells. View from the top (A,B) and from the side (C,D).
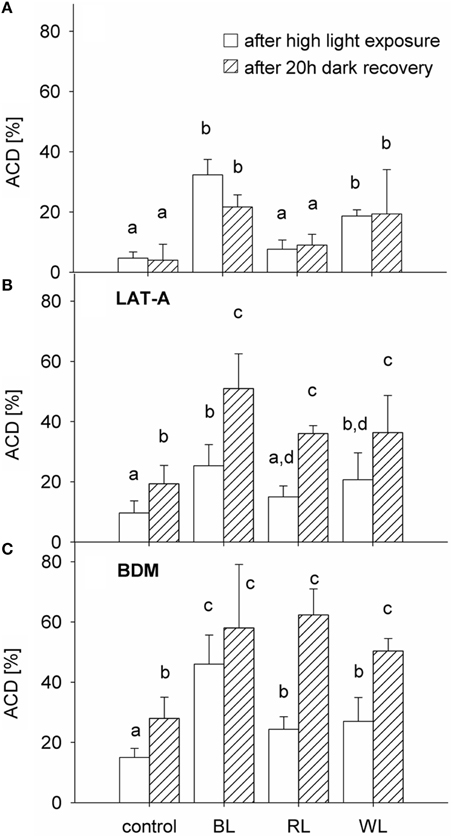
Figure 3. Proportion of Coscinodiscus granii cells with an altered chloroplast distribution (ACD), after 1 h exposure to either 600 μmol m−2 s−1 BL, 1500 μmol m−2 s−1 RL, or 1250 μmol m−2 s−1 WL (after exposure) and after 20 h of recovery in the dark (after 20 h recovery) (A) in absence and presence of the cytoskeleton inhibitors LAT-A (B) and BDM (C). Average values and standard error (n = 3) are indicated. Statistically significant differences between the treatments are indicated as different small letters (p < 0.05).
Time and Dose-Dependent Induction of Altered Chloroplast Distribution Patterns
Both time and dose of BL-exposure did significantly increase altered chloroplast distribution patterns [F(2, 53) = 33.866; p < 0.001; Figure 4]. Compared to the control (dark), there was no significant increase in the altered chloroplast distribution pattern after 10 min of BL-exposure, even under a photon irradiance of 1000 μmol photons m−2 s−1 (p = 0.275). After 30 min of BL-exposure, there was a significantly increased frequency of cells with altered chloroplast distribution [12 ± 5% at 700 μmol photons m−2 s−1 (p = 0.001), and of 18 ± 4% at 1000 μmol photons m−2 s−1 (p < 0.001)]. A significantly increased frequency of cells with altered chloroplast distribution to 14 ± 3% after 60 min was found under lower photon irradiance of 300 μmol photons m−2 s−1 of BL (p < 0.001). After 60 min of BL exposure, the frequency of altered chloroplast distribution did further increase reaching maximum values of 37 ± 5% under a photon irradiance of 1000 μmol photons m−2 s−1 of BL (p < 0.001).
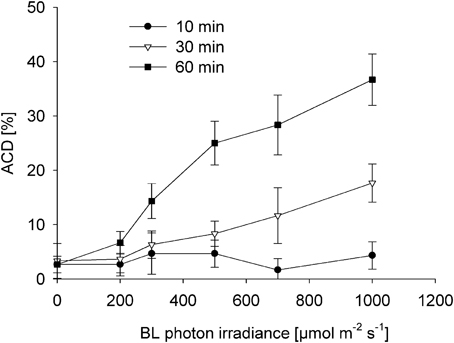
Figure 4. Induction of altered chloroplast distribution (ACD) in Coscinodiscus granii after 10, 30, and 60 min of exposure to increasing BL photon irradiance levels. Average values and standard error (n = 3) are indicated.
Single Cell Variable Chlorophyll Fluorescence and Cell Mortality
Cells with altered chloroplast distribution exhibited a significant decrease in Y(II), independently from whether cells were treated with high levels of blue light (600 μmol m−2 s−1 BL) for 1 h, or if they were kept in the dark [F(1, 19) = 727.167; p < 0.001; Figure 5A]. In untreated cells, Y(II) decreased from 0.44 ± 0.02 in cells with evenly distributed chloroplasts to 0.00 ± 0.01 in cells with altered chloroplast distribution (p < 0.001; compare Figure 6). After BL exposure, Y(II) decreased from 0.35 ± 0.03 in cells with evenly distributed chloroplasts to 0.00 ± 0.08 in cells with altered chloroplast distribution (p < 0.001). Recovery for 20 h in the dark did not significantly change the Y(II) of cells with altered chloroplast distribution [F(1, 39) = 0.199; p = 0.658; Figure 5B].
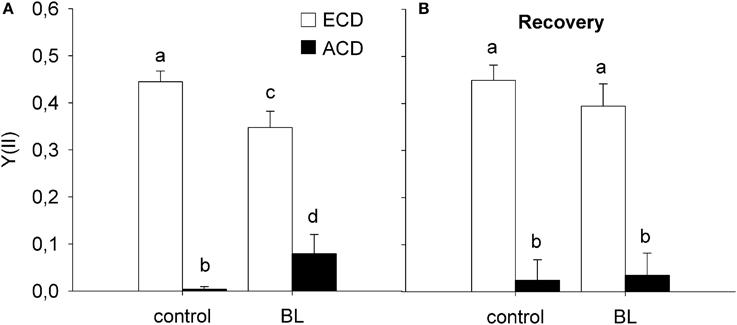
Figure 5. Variable chlorophyll fluorescence in cells of Coscinodiscus granii showing an even chloroplast distribution (ECD) or an altered chloroplast distribution (ACD) after exposure to high blue light (A) and after recovery in the dark (B). Statistically significant differences between the treatments are indicated as different small letters (p < 0.05).
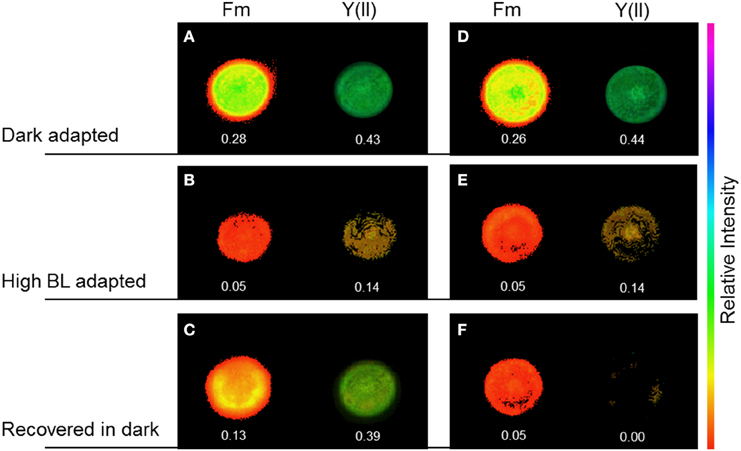
Figure 6. Maximal chlorophyll fluorescence yield, Fm′, and effective PSII quantum yield, Y(II), in Coscinodiscus granii cells during alteration of the chloroplast arrangement under high BL photon irradiance. In cells with an even chloroplast distribution (ECD) (A–D), Y(II) decreased upon illumination with BL for 30 min (B) and recovered after 30 min in the dark (C). After an alteration of the chloroplast distribution, Y(II) decreased after illumination with BL for 30 min (E) and did not recover after 30 min in the dark (F).
Sytox® Green Lethality Staining
Sytox® green showed positive staining for only 7 ± 4% of cells with an even chloroplast distribution. The percentage cells showing an altered chloroplast distribution pattern that were stained with Sytox® green was significantly higher [F(1, 5) = 27.505; p = 0.006] reaching 51 ± 9% (Figure 7).
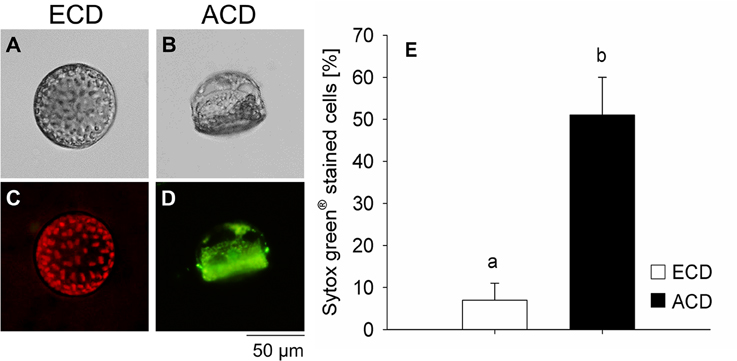
Figure 7. Lethality test using Sytox® green staining on Coscinodiscus granii cells with an even chloroplast distribution (ECD) (A,C) or an altered chloroplast distribution (ACD) (B,D). (E) Proportion of cells that were positively tested on lethality. (C) Cells with an ECD always showed a pronounced chlorophyll auto-fluorescence. Average values and standard error (n = 3) are indicated. Statistically significant differences between the treatments are indicated as different small letters (p < 0.05).
Discussion
In the present study, effects of BL, RL, and WL on photosynthesis, and light-induced alteration of the chloroplast distribution in the centric diatom C. granii were investigated. Blue light was channeled much more efficiently to PSII than WL and RL based on Sigma(II)λ measurements (Table 1). This observation is in accordance with studies on the pennate diatom Phaeodactylum tricornutum (Schellenberger Costa et al., 2013) and other phytoplankton species (Gilbert et al., 2000). Photosynthetic electron transport from PSII, ETR(II) saturated sooner under BL, and reached much higher levels than under RL or WL (Table 1; Figure 1A). We also observed a higher energy dissipation via NPQ under BL (Figure 1B) while RL-induced NPQ was very low and did not significantly increase with irradiance. Overall, high Ek and high ETRmax observed under all applied spectral regimes indicate an outstanding photosynthetic capacity of C. granii (Table 1), indicating that this diatom can employ efficient mechanisms to dissipate excessive irradiance and/or to prevent damages to the photosynthetic apparatus.
BL, RL, and WL were used to induce an alteration of the chloroplast distribution in C. granii. Based on measurements of Sigma(II)λ we could adjust the actinic light levels under the different spectral regimes ensuring equal amounts of light absorbed by the PS(II) antennae, i.e., 600 μmol m−2 s−1 of BL, 1500 μmol m−2 s−1 of RL, and 1250 μmol m−2 s−1 of WL; all irradiance levels were well beyond the saturation point of photosynthesis in Coscinodiscus (Table 1).
Under low light conditions, the chloroplasts were evenly distributed within the cortical stroma (Figures 2A,C). Only high doses of BL and WL promoted an alteration of the intracellular chloroplast arrangement in a significant number of cells by aggregation of chloroplasts in the cortical cytoplasm (Figures 2B,D). Interestingly, the number of cells showing an alteration of the chloroplast arrangement was not increased when high RL was applied (Figure 3A), although incident RL irradiances were much higher (Table 1). High-light avoidance movement of chloroplasts and reversion toward an evenly distribution under low light can reduce light-induced stress and optimize photosynthetic performance under fluctuating light regimes (Haupt, 1973). Chloroplast movement is mediated by photoreceptors that have been found in diatoms (Schnitzler Parker et al., 2004; Montsant et al., 2005; Bowler et al., 2008; Depauw et al., 2012). It was therefore expected that C. granii performed chloroplast high-light avoidance movement under high light stress. Yogamoorthi (2007) observed a dose-dependent alteration of the chloroplast arrangement in Coscinodiscus gigas toward a more aggregated state after treatment with UV-B irradiation. In our study, an increase of cells exhibiting an altered/aggregated chloroplast arrangement was observed depending on irradiance level, exposure time and light color. However, we did not observe any reversion toward a more even chloroplast distribution pattern even after a recovery of 20 h in the dark (Figure 3A). Such reversion could be expected under low light conditions or upon far RL illumination (Kasahara et al., 2004) and in the diatom Pleurosira laevis, reversal from an aggregated chloroplast distribution toward a more even distribution has also been observed under green light (Shihira-Ishikawa et al., 2007).
Both, high-light avoidance movements and back movement are mediated through the cytoskeleton. While high-light avoidance movement is mediated by myosin, back movement is regulated by actin filaments (Sato et al., 2001; Takagi, 2003; Krzeszowiec et al., 2007; Paves and Truve, 2007). In presence of inhibitors of both actin- and myosin-mediated movements, we found an increasing number of C. granii cells with an altered chloroplast pattern (Figures 3B,C). These results are in contrast to the observation of Shihira-Ishikawa et al. (2007), describing inhibition of BL-induced high-light avoidance movement of chloroplasts with myosin-disrupting agents, and inhibition of green light (GL)-induced back movement using actin-inhibitors in the centric diatom Pleurosira laevis. After recovery in the dark, we observed that the amount of C. granii cells with an altered chloroplast distribution even increased in presence of cytoskeleton inhibitors (Figures 3B,C), indicating potential lethal effects of such long-term incubation. It was also surprising that the number of cells showing an alteration in chloroplast arrangement did not increase within 10 min at high BL doses (Figure 4) and only after 30 min at >700 μmol photons m−2 s−1, did the number of cells with an altered chloroplast pattern increase significantly. Earlier studies showed that high-light avoidance chloroplast movement is induced within a few minutes in terrestrial phototrophs (Kasahara et al., 2002; Wada et al., 2003) as well as in micro- and macro-algae (Haupt, 1973), including diatoms (e.g., Furukawa et al., 1998). Fast induction of chloroplast high-light avoidance movement might prevent photo-induced damages of the photo apparatus on the short term but if such movement would take too long, the system could already be damaged.
To estimate the photosynthetic state of cells with an altered chloroplast distribution, measurements of variable chlorophyll fluorescence were performed on the cellular scale. Surprisingly, cells with an altered chloroplast distribution showed a dramatic loss PSII quantum yield, Y(II) (Figures 5A, 6). While a reduction could be interpreted as a physiological protection to high light (Genty et al., 1989), total loss of Y(II) rather indicates absence of photochemical quenching and an inactive photosynthetic apparatus. This could be explained by severe damage due to excessive light. Furthermore, Y(II) of cells with an altered chloroplast distribution did not recover even after a dark period of 20 h (Figure 5B) and application of the SYTOX® green stain revealed that a high proportion of cells with an altered chloroplast arrangement were actually dead (Figure 7).
The cause for alterations in the chloroplast distribution in C. granii remains unclear. After exposure to high irradiance, alteration of the chloroplast arrangement occurred only after a period of >30 min and then the alteration process happened within seconds, occasionally followed by a cracking of the frustule and the release of chloroplasts. This observation indicates a time-dependent accumulation of stress under high light exposure, until a critical concentration threshold is reached triggering rapid movement and aggregation of chloroplasts. We speculate that such stress could be related with the formation of reactive oxygen species, combined with a dramatic decrease of the luminal redox state to a level, which can no longer be buffered by protection mechanisms such as the Ddx cycle. We note that the light doses needed to induce such an apparently irreversible alteration of the chloroplast arrangement reflect irradiance maxima occurring in the aquatic environment of Coscinodiscus. Nevertheless, it is even more astonishing that a majority of cells did neither show an alteration of their chloroplast arrangement (Figure 3A), nor a significant decrease in variable chlorophyll fluorescence even after long term exposure of 1 h (Figure 4). Such apparent high light tolerance of C. granii was supported by the observation that cells with an evenly distributed chloroplast pattern did not show a positive Sytox®green death staining even after 1 h of exposure to high BL irradiance (Figure 7).
Our results suggest that C. granii can sustain high irradiance. However, this capacity is not promoted by chloroplast high-light avoidance movements, and Coscinodiscus may thus use other mechanisms to stabilize their cellular light environment. The effective cross-sections for photochemistry, of large diatom species such as Coscinodiscus is usually lower compared to smaller cells, hence showing lower susceptibility to photo inactivation (Key et al., 2010). Furthermore, diatoms have been shown to have exceptionally high capacity for rapid and large induction of the xanthophyll cycle under light stress leading to the thermal dissipation of harmful excess energy (Ruban et al., 2004). The silica frustule stabilizes the diatom cell and protects against predation (Hamm et al., 2003), but the frustule of diatoms also has unique optical properties (e.g., Fuhrmann et al., 2004; De Stefano et al., 2007; Lettieri et al., 2008; Chen et al., 2015), and recent work suggest that the silica frustule also plays a role in adaptation to spectral light changes (Su et al., 2015). High photosynthetic efficiencies in the centric diatom C. granii thus might be promoted by physiological mechanisms and by structural features on the microscale, but not by chloroplast high-light avoidance movement.
Author Contributons
Experimental design (JG, PC, MK), experimental work (JG, PC), data analysis (JG, PC, MK). JG wrote manuscript with input from PC and MK.
Funding
This study was supported by a Sapere-Aude Advanced grant from the Danish Council for Independent Research Natural Sciences (MK) and by the Carlsberg Foundation (MK).
Conflict of Interest Statement
The authors declare that the research was conducted in the absence of any commercial or financial relationships that could be construed as a potential conflict of interest.
Acknowledgments
We thank Gert Hansen for providing cultures from the Scandinavian Culture Collection and Erik Trampe for help and introduction to variable chlorophyll fluorescence microscopy. We further thank Sonia Cruz, Mads Lichtenberg, and Klaus Koren for valuable input during the project conception and Sofie L. Jakobsen for technical assistance. We appreciate the significant improvement of this manuscript by two reviewers.
References
Apel, K., and Hirt, H. (2004). Reactive oxygen species: metabolism, oxidative stress, and signal transduction. Annu. Rev. Plant Biol. 55, 373–399. doi: 10.1146/annurev.arplant.55.031903.141701
Armbrecht, L. H., Smetacek, V., Assmy, P., and Klaas, C. (2014). Cell death and aggregate formation in the giant diatom Coscinodiscus wailesii (Gran & Angst, 1931). J. Exp. Mar. Biol. Ecol. 452, 31–39. doi: 10.1016/j.jembe.2013.12.004
Bowler, C., Allen, A. E., Badger, J. H., Grimwood, J., Jabbari, K., Kuo, A., et al. (2008). The Phaeodactylum genome reveals the evolutionary history of diatom genomes. Nature 456, 239–244. doi: 10.1038/nature07410
Boyd, P. W., Watson, A. J., Law, C. S., Abraham, E. R., Trull, T., Murdoch, R., et al. (2000). A mesoscale phytoplankton bloom in the polar Southern Ocean stimulated by iron fertilization. Nature 407, 695–702. doi: 10.1038/35037500
Cartaxana, P., Brotas, V., and Serôdio, J. (2008). Effects of two motility inhibitors on the photosynthetic activity of the diatoms Cylindrotheca closterium and Pleurosigma angulatum. Diatoms Res. 23, 65–74. doi: 10.1080/0269249X.2008.9705737
Chen, S. T., and Li, C. W. (1991). Relationships between the movements of chloroplasts and cytoskeletons in diatoms. Botanica Marina 34, 505–511. doi: 10.1515/botm.1991.34.6.505
Chen, X., Wang, C., Baker, E., and Sun, C. (2015). Numerical and experimental investigation of light trapping effect of nanostructured diatom frustules. Sci. Rep. 5, 11977. doi: 10.1038/srep11977
DeBlasio, S. L., Mullen, J. L., Luesse, D. R., and Hangarter, R. P. (2003). Phytochrome modulation of blue light-induced chloroplast movements in Arabidopsis. Plant Physiol. 133, 1471–1479. doi: 10.1104/pp.103.029116
Demming-Adams, B., and Adams, W. W. (1992). Photoprotection and other responses of plants to high light stress. Annu. Rev. Plant Physiol. 43, 599–626. doi: 10.1146/annurev.pp.43.060192.003123
Depauw, F. A., Rogato, A., D'Alcalá, M. R., and Falciatore, A. (2012). Exploring the molecular basis of responses to light in marine diatoms. J. Exp. Bot. 63, 1575–1591. doi: 10.1093/jxb/ers005
De Stefano, L., Rea, I., Rendina, I., De Stefano, M., and Moretti, L. (2007). Lensless light focusing with the centric marine diatom Coscinodiscus walesii. Opt. Express 15, 18082–18088. doi: 10.1364/OE.15.018082
Eilers, P. H. C., and Peeters, J. C. H. (1988). A model for the relationship between light intensity and the rate of photosynthesis in phytoplankton. Ecol. Modell. 42, 199–215. doi: 10.1016/0304-3800(88)90057-9
Fuhrmann, T., Landwehr, S., El Rharbi-Kucki, M., and Sumper, M. (2004). Diatoms as living photonic crystals. Appl. Phys. B 78, 257–260. doi: 10.1007/s00340-004-1419-4
Furukawa, T., Watanabe, M., and Shihira-Ishikawa, I. (1998). Green- and blue-light-mediated chloroplast migration in the centric diatomPleurosira laevis. Protoplasma 203, 214–220. doi: 10.1007/BF01279479
Gabryś, H. (2004). Blue light-in duced ori en ta tion move ments of chloroplasts in higher plants: re cent prog ress in the study of their mechanisms. Acta Physiol. Plant. 26, 473–478. doi: 10.1007/s11738-004-0038-3
Geider, R. J., Delucia, E. H., Falkowski, P. G., Finzi, A. C., Grime, J. P., Grace, J., et al. (2001). Primary productivity of planet earth: biological determinants and physical constraints in terrestrial and aquatic habitats. Glob. Chang. Biol. 7, 849–882. doi: 10.1046/j.1365-2486.2001.00448.x
Genty, B., Briantais, J.-M., and Baker, N. R. (1989). The relationship between the quantum yield of photosynthetic electron transport and quenching of chlorophyll fluorescence. Biochim. Biophys. Acta 990, 87–92. doi: 10.1016/S0304-4165(89)80016-9
Gilbert, M., Wilhelm, C., and Richter, M. (2000). Bio-optical modelling of oxygen evolution using in vivo fluorescence: comparison of measured and calculated photosynthesis/irradiance (P-I) curves in four representative phytoplankton species. J. Plant Physiol. 157, 307–314. doi: 10.1016/S0176-1617(00)80052-8
Gillard, J., Devos, V., Huysman, M. J. J., De Veylder, L., D'Hondt, S., Martens, C., et al. (2008). Physiological and transcriptomic evidence for a close coupling between chloroplast ontogeny and cell cycle progression in the pennate diatom Seminavis robusta. Plant Physiol. 148, 1394–1411. doi: 10.1104/pp.108.122176
Goss, R., and Jakob, T. (2010). Regulation and function of xanthophyll cycle-dependent photoprotection in algae. Photosyn. Res. 106, 103–122. doi: 10.1007/s11120-010-9536-x
Guillard, R. R. L., and Hargraves, P. E. (1993). Stichocrysis immobilis is a diatom, not a chrysophyte. Phycologica 32, 234–236. doi: 10.2216/i0031-8884-32-3-234.1
Hamm, C. E., Merkel, R., Springer, O., Jurkojc, P., Maier, C., Prechtel, K., et al. (2003). Architecture and material properties of diatom shells provide effective mechanical protection. Nature 421, 841–843. doi: 10.1038/nature01416
Jeffryes, C., Agathos, S. N., and Rorrer, G. (2015). Biogenic nanomaterials from photosynthetic microorganisms. Curr. Opin. Biotechnol. 33, 23–31. doi: 10.1016/j.copbio.2014.10.005
Kasahara, M., Kagawa, T., and Oikawa, K. (2002). Chloroplast avoidance movement reduces photodamage in plants. Lett. Nat. 420, 829–832. doi: 10.1038/nature01213
Kasahara, M., Kagawa, T., Sato, Y., Kiyosue, T., and Wada, M. (2004). Phototropins mediate blue and red light-induced chloroplast movements in Physcomitrella patens. Plant Physiol. 135, 1388–1397. doi: 10.1104/pp.104.042705
Kasperbauer, M. J. (1987). Far-red light reflection from green leaves and effects on phytochrome-mediated assimilate partitioning under field conditions. Plant Physiol. 85, 350–354. doi: 10.1104/pp.85.2.350
Key, T., McCarthy, A., Campbell, D. A., Six, C., Roy, S., and Finkel, Z. V. (2010). Cell size trade-offs govern light exploitation strategies in marine phytoplankton. Environ. Microbiol. 12, 95–104. doi: 10.1111/j.1462-2920.2009.02046.x
Kiefer, D. A. (1973). Chlorophyll a fluorescence in marine centric diatoms: responses of chloroplasts to light and nutrient stress. Mar. Biol. 23, 39–46. doi: 10.1007/BF00394110
Krzeszowiec, W., Rajwa, B., Dobrucki, J., and Gabryś, H. (2007). Actin cytoskeleton in Arabidopsis thaliana under blue and red light. Biol. Cell 99, 251–260. doi: 10.1042/BC20060077
Lettieri, S., Setaro, A., De Stefano, L., De Stefano, M., and Maddalena, P. (2008). The gas-detection properties of light-emitting diatoms. Adv. Funct. Mater. 18, 1257–1264. doi: 10.1002/adfm.200701124
Mock, T., and Valentin, K. (2004). Photosynthesis and cold acclimation: molecular evidence from a polar diatom. J. Phycol. 40, 732–741. doi: 10.1111/j.1529-8817.2004.03224.x
Montsant, A., Jabbari, K., Maheswari, U., and Bowler, C. (2005). Comparative genomics of the pennate diatom Phaeodactylum tricornutum. Plant Physiol. 137, 500–513. doi: 10.1104/pp.104.052829
Nelson, D. M., and Brzezinski, M. A. (1997). Diatom growth and productivity in an oligo-trophic midocean gyre: a 3-yr record from the Sargasso Sea near Bermuda. Limnol. Oceanogr. 42, 473–486. doi: 10.4319/lo.1997.42.3.0473
Park, Y. I., Chow, W. S., and Anderson, J. M. (1996). Chloroplast movement in the shade plant tradescantia albiflora helps protect photosystem II against light stress. Plant Physiol. 111, 867–875.
Parker, A. R., and Townley, H. E. (2007). Biomimetics of photonic nanostructures. Nat. Nanotechnol. 2, 347–353. doi: 10.1038/nnano.2007.152
Paves, H., and Truve, E. (2007). Myosin inhibitors block accumulation movement of chloroplasts in Arabidopsis thaliana leaf cells. Protoplasma 230, 165–169. doi: 10.1007/s00709-006-0230-y
Ruban, A., Lavaud, J., Rousseau, B., Guglielmi, G., Horton, P., and Etienne, A. L. (2004). The super-excess energy dissipation in diatom algae: comparative analysis with higher plants. Photosyn. Res. 82, 165–175. doi: 10.1007/s11120-004-1456-1
Sato, Y., Wada, M., and Kadota, A. (2001). Choice of tracks, microtubules and/or actin filaments for chloroplast photo-movement is differentially controlled by phytochrome and a blue light receptor. J. Cell Sci. 114(Pt 2), 269–279. Retrieved from http://www.ncbi.nlm.nih.gov/pubmed/11148129
Schellenberger Costa, B., Jungandreas, A., Jakob, T., Weisheit, W., Mittag, M., and Wilhelm, C. (2013). Blue light is essential for high light acclimation and photoprotection in the diatom Phaeodactylum tricornutum. J. Exp. Bot. 64, 483–493. doi: 10.1093/jxb/ers340
Schmid, A.-M. M., and Volcani, B. E. (1983). Wall morphogenesis in coscinodiscus wailesii gran and angst. I. valve morphology and development of its architecture. J. Phycol. 19, 387–402. doi: 10.1111/j.0022-3646.1983.00387.x
Schnitzler Parker, M., Armbrust, E. V., Piovia-Scott, J., and Keil, R. G. (2004). Induction of photorespiration by light in the centric diatom Thalassiosira weissflogii (Bacillariophyceae): molecular characterization and physiological consequences. J. Phycol. 40, 557–567. doi: 10.1111/j.1529-8817.2004.03184.x
Schreiber, U., Klughammer, C., and Kolbowski, J. (2012). Assessment of wavelength-dependent parameters of photosynthetic electron transport with a new type of multi-color PAM chlorophyll fluorometer. J. Photosyn. Res. 113, 127–144. doi: 10.1007/s11120-012-9758-1
Schumann, A., Goss, R., Jakob, T., and Wilhelm, C. (2007). Investigation of the quenching efficiency of diatoxanthin in cells of Phaeodactylum tricornutum (Bacillariophyceae) with different pool sizes of xanthophyll cycle pigments. Phycologia 46, 113–117. doi: 10.2216/06-30.1
Shihira-Ishikawa, I., Nakamura, T., Higashi, S. I., and Watanabe, M. (2007). Distinct responses of chloroplasts to blue and green laser microbeam irradiations in the centric diatom Pleurosira laevis. Photochem. Photobiol. 83, 1101–1109. doi: 10.1111/j.1751-1097.2007.00167.x
Strasser, B., Sánchez-Lamas, M., Yanovsky, M. J., Casal, J. J., and Cerdán, P. D. (2010). Arabidopsis thaliana life without phytochromes. Proc. Natl. Acad. Sci. U.S.A. 107, 4776–4781. doi: 10.1073/pnas.0910446107
Su, Y., Friis, S. M. M., Lundholm, N., and Ellegaard, M. (2015). Implications for photonic applications of diatom growth and frustule nanostructure changes in response to different light wavelengths. Nano Res. 8, 2363–2372. doi: 10.1007/s12274-015-0746-6
Sumper, M., and Brunner, E. (2006). Learning from diatoms: nature's tools for the production of nanostructured silica. Adv. Funct. Mater. 16, 17–26. doi: 10.1002/adfm.200500616
Takagi, S. (2003). Actin-based photo-orientation movement of chloroplasts in plant cells. J. Exp. Biol. 206, 1963–1969. doi: 10.1242/jeb.00215
Thomas, D. N., and Dieckmann, G. S. (2002). Antarctic Sea ice–a habitat for extremophiles. Science 295, 641–644. doi: 10.1126/science.1063391
Trampe, E., Kolbowski, J., Schreiber, U., and Kühl, M. (2011). Rapid assessment of different oxygenic phototrophs and single-cell photosynthesis with multicolour variable chlorophyll fluorescence imaging. Mar. Biol. 158, 1667–1675. doi: 10.1007/s00227-011-1663-1
Wada, M., Kagawa, T., and Sato, Y. (2003). Chloroplast movement. Annu. Rev. Plant Biol. 54, 455–468. doi: 10.1146/annurev.arplant.54.031902.135023
Wight, A. J., and Critchley, C. (1999). Rapid light curves: a new fluorescence method to assess the state of the photosynthetic apparatus. J. Photosyn. Res. 59, 63–72. doi: 10.1023/A:1006188004189
Yogamoorthi, A. (2007). Artificial UV-B induced changes in pigmentation of marine diatom Coscinodiscus gigas. J. Environ. Biol. 28, 327–30. Retrieved from: http://www.ncbi.nlm.nih.gov/pubmed/17915774
Keywords: PSII functional absorption cross-section, spectral quality, light stress, non-photochemical quenching, photosynthesis, high-light avoidance movement, diatoms
Citation: Goessling JW, Cartaxana P and Kühl M (2016) Photo-Protection in the Centric Diatom Coscinodiscus granii is Not Controlled by Chloroplast High-Light Avoidance Movement. Front. Mar. Sci. 2:115. doi: 10.3389/fmars.2015.00115
Received: 12 October 2015; Accepted: 10 December 2015;
Published: 08 January 2016.
Edited by:
Karla B. Heidelberg, University of Southern California, USACopyright © 2016 Goessling, Cartaxana and Kühl. This is an open-access article distributed under the terms of the Creative Commons Attribution License (CC BY). The use, distribution or reproduction in other forums is permitted, provided the original author(s) or licensor are credited and that the original publication in this journal is cited, in accordance with accepted academic practice. No use, distribution or reproduction is permitted which does not comply with these terms.
*Correspondence: Johannes W. Goessling, johannesg@bio.ku.dk