Reefs under Siege—the Rise, Putative Drivers, and Consequences of Benthic Cyanobacterial Mats
- 1Ecology Department, Leibniz Centre for Tropical Marine Research (ZMT), Bremen, Germany
- 2Faculty of Biology and Chemistry (FB2), University of Bremen, Bremen, Germany
- 3EPHE, PSL Research University, UPVD-Centre National de la Recherche Scientifique, USR3278 CRIOBE, Perpignan, France
- 4Labex Corail, CRIOBE, Moorea, French Polynesia
- 5Department of Freshwater and Marine Ecology, Institute for Biodiversity and Ecosystem Dynamics, University of Amsterdam, Amsterdam, Netherlands
- 6School of Civil Engineering, University of Queensland, Brisbane, QLD, Australia
Benthic cyanobacteria have commonly been a small but integral component of coral reef ecosystems, fulfilling the critical function of introducing bioavailable nitrogen to an inherently oligotrophic environment. Though surveys may have previously neglected benthic cyanobacteria, or grouped them with more conspicuous benthic groups, emerging evidence strongly indicates that they are becoming increasingly prevalent on reefs worldwide. Some species can form mats comprised by a diverse microbial consortium which allows them to exist across a wide range of environmental conditions. This review evaluates the putative driving factors of increasing benthic cyanobacterial mats, including climate change, declining coastal water quality, iron input, and overexploitation of key consumer and ecosystem engineer species. Ongoing global environmental change can increase growth rates and toxin production of physiologically plastic benthic cyanobacterial mats, placing them at a considerable competitive advantage against reef-building corals. Once established, strong ecological feedbacks [e.g., inhibition of coral recruitment, release of dissolved organic carbon (DOC)] reinforce reef degradation. The review also highlights previously overlooked implications of mat proliferation, which can extend beyond reef health and affect human health and welfare. Though identifying (opportunistic) consumers of mats remains a priority, their perceived low palatability implies that herbivore management alone may be insufficient to control their proliferation and must be accompanied by local measures to improve water quality and watershed management.
Introduction
Without the earliest cyanobacteria that evolved around three billion years ago introducing oxygen to the atmosphere (Brocks et al., 1999), life as we know it would not exist. Today marine cyanobacteria remain an integral component of healthy ecosystems, offering critical functions such as nitrogen fixation and primary production (Charpy et al., 2007). Such roles are particularly important within oligotrophic systems such as coral reefs, where cyanobacteria are profusely embedded in algal turfs and microbial biofilms (Barott et al., 2011; Fricke et al., 2011; Charpy et al., 2012a; Cardini et al., 2014). Under certain environmental conditions, some benthic marine cyanobacteria, which may have previously been a benign component of the benthos, can form dense mats (hereon referred to as benthic cyanobacterial mats) comprising species from a single genus (Paul et al., 2005) or diverse microbial consortia (Echenique-Subiabre et al., 2015). While receiving relatively little attention in benthic reef ecology studies to date, these mats should be considered as major players on degraded or degrading reefs where they are increasingly reported to cover extensive areas (Figure 1; Table 1). Rectifying this oversight is critical because reefs dominated by benthic cyanobacterial mats are likely to produce significantly less of the ecosystem services associated with healthy, coral-dominated reefs. For example, coastal protection and fisheries decline as structural complexity is reduced on degraded reefs (Sheppard et al., 2005; Pratchett et al., 2014). Considering rapid reef degradation worldwide, it is thus important to better understand the biology and ecology of tropical benthic cyanobacterial mats.
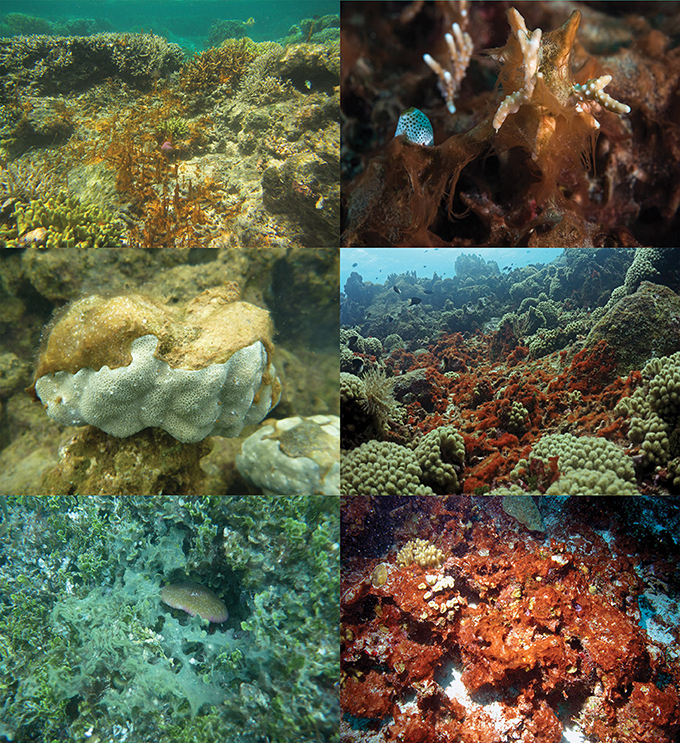
Figure 1. Photographs of benthic cyanobacterial mats on coral reefs. From top-left clockwise: cyanobacterial mat at Coral Coast, Fiji (credits: Victor Bonito); cyanobacterial mat overgrowing coral at Menjangan Island, Bali (credits: Tom Vierus); Oscillatoria-dominated mat at Curaçao (credits: Benjamin Mueller); Oscillatoria-dominated mat at Curaçao (credits: Maggy Nugues); cyanobacterial mat overgrowing Halimeda algae at Ahus Island, Papua New Guinea (credits: Amanda Ford); cyanobacteria mat overgrowing coral at Marovo Lagoon, Solomon Islands (credits: Simon Albert).
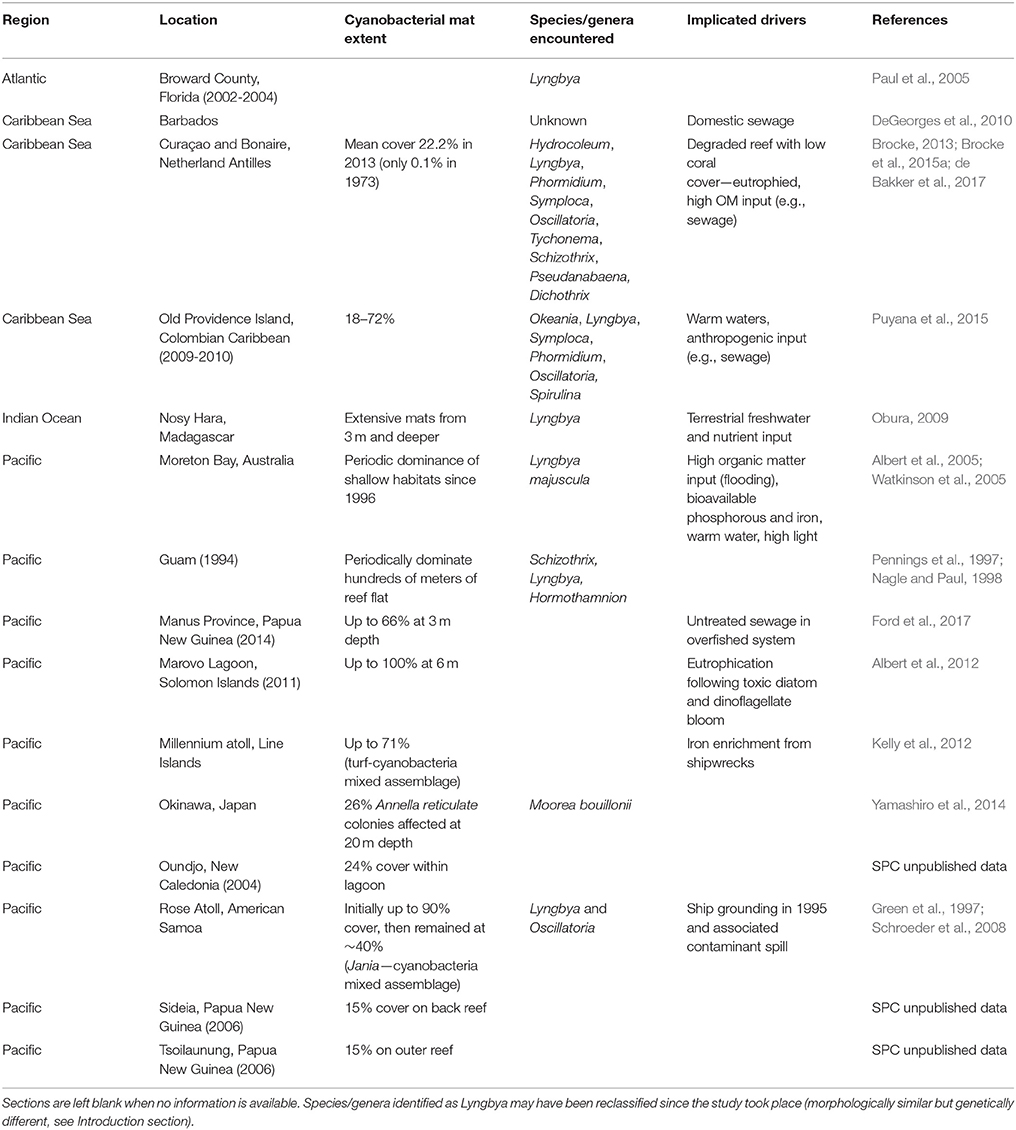
Table 1. Reports of benthic cyanobacterial mats from tropical coasts worldwide, with genus/species identification and implicated drivers given where possible.
Modern (phylo)genetic approaches have revealed that species richness in tropical cyanobacterial mats is very high and includes many unknown species otherwise overlooked by traditional morphology-based taxonomic approaches (Engene et al., 2013a; Echenique-Subiabre et al., 2015). For example, though filamentous mat-forming cyanobacteria have regularly been grouped within the genus Lyngbya (C. Aghard ex Gomont 1892), recent analyses have separated many species from this group into newly-described genera such as Moorea (Engene et al., 2012) and Okeania (Engene et al., 2013b). Within mats, tropical cyanobacteria probably also associate with a consortium of heterotrophic bacteria, as shown for mats in other regions (Stal, 2012). Microbial consortia are likely to enhance production, growth rates, and nutrient cycling over what a single population alone can achieve under similar environmental settings (Paerl et al., 2000). Indeed, mats can exhibit high plasticity in their ability to modify the diversity and composition of their constituting microbial assemblages in response to their local environment (Echenique-Subiabre et al., 2015).
Benthic cyanobacterial mats usually inhabit a very small proportion of reef space (e.g., <1%; Bednarz et al., 2015), but can exhibit higher nitrogen fixation rates (8–110 mg nitrogen m−2 day−1) compared to (cyano)bacteria associated with other benthic groups such as algal turfs and fleshy algae (0.44–22.69 mg nitrogen m−2 day−1) or scleractinian corals (0–9.75 mg nitrogen m−2 day−1, Cardini et al., 2014). Furthermore, nitrogen fixation of (seasonal) mats is up to one order of magnitude higher than surrounding sediments and/or rubble (Casareto et al., 2008; Bednarz et al., 2015), and even when they are uncommon (<2% benthic coverage) they have been found to contribute up to 27–64% of benthic nitrogen fixation (Cardini et al., 2016). Heterocystous species that can simultaneously fix nitrogen and photosynthesise are not advantageous in oligotrophic tropical waters, and indeed non-heterocystous planktonic Trichodesmium are the dominant nitrogen fixers in tropical oceans (Staal et al., 2003; Stal, 2012). Mats have been shown to comprise heterocystous and/or non-heterocystous cyanobacteria, with both strategists in some instances fixing nitrogen at similar rates (Charpy et al., 2010, 2012b). There is however a high variation in the ability to fix nitrogen within benthic cyanobacteria (Zehr, 2011), with some common mat-forming types including Moorea in fact lacking the genes involved in nitrogen fixation (Engene et al., 2012). Depending on local environmental conditions, cyanobacterial mats are able to modulate their nitrogen fixation activity (increasing when nitrogen is limiting, decreasing when nitrogen is high) via changes in overall taxonomic composition or the activity of individual nitrogen-fixing species (Paerl, 2008; Charpy et al., 2012b).
A recent surge of reports of benthic cyanobacterial mats occurring or proliferating on coral reefs (Table 1), as well as concern for the detrimental effects of these mats on ecosystem processes, stimulated this scientific review. Surveys conducted over decadal scales (40 years) provide robust evidence that cyanobacterial mats are increasing on some southern Caribbean reefs alongside a decline in organisms such as scleractinian corals (de Bakker et al., 2017). Also in subtropical areas, blooms of benthic cyanobacterial mats which were first restricted to the summer months have become significantly more persistent (Albert et al., 2005; Paul et al., 2005). Increased awareness of the presence of cyanobacteria may account at least partially for the rise of reports (e.g., Duarte et al., 2015). Nonetheless it is clear that mats have received much less attention than other reef organisms (Figure 2A), with the first reef-focused scientific article mentioning cyanobacterial mats only published in 1991 compared to 1930 and 1959 for algae and sponges, respectively according to Web of Science® (Figure 2B). Underreporting may be due to cyanobacteria remaining undistinguished from (i) more conspicuous reef benthic groups such as algal turfs (Kuffner and Paul, 2001; Fong and Paul, 2011) within which they are often dominant (Fricke et al., 2011), (ii) the organisms that they grow over (e.g., fleshy algae—Puyana and Prato, 2013), or (iii) abiotic substrate such as dead coral pavement, sand or rubble which is realistically never bare (Harris, 2015). Arguably, temporary blooms of cyanobacterial mats might have historically been a natural phenomenon on reefs, exhibiting a pulsing nature (Puyana et al., 2015) that may not be prolonged or stable enough to be considered regime shifts themselves. These blooms may however catalyse long-lasting shifts from coral-dominated reefs to other alternative states (Kelly et al., 2012). Even such conspicuous phenomena as mass spawning of corals have only been reported relatively recently (Harrison et al., 1984), underlining the dearth of scientific knowledge on basic ecological phenomena in reefs.
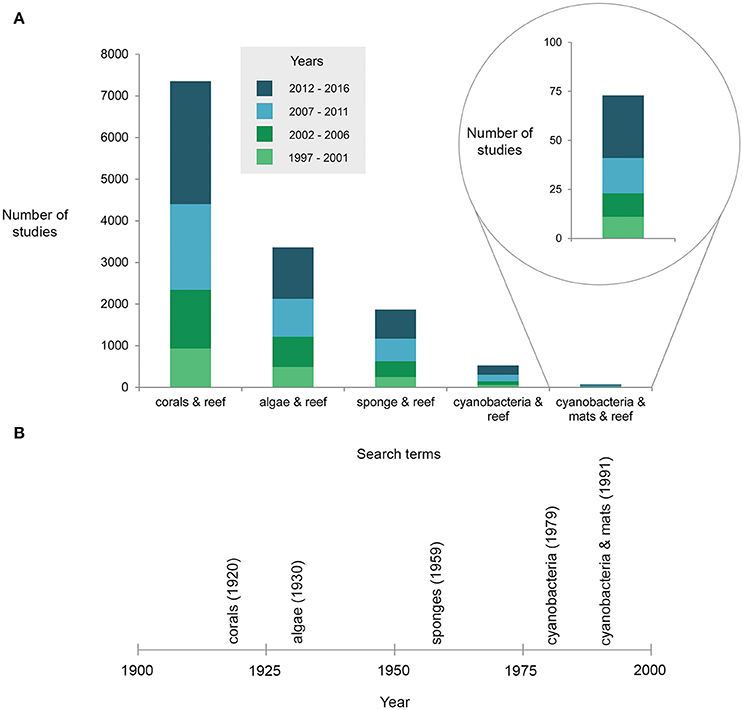
Figure 2. (A) Results from a Web of Science® search depicting the number of articles published since 1997 (displayed in five-yearly intervals) according to various coral reef associated search terms, and (B) the year of the first publication including the search terms (each term searched with ‘reef’).
In light of the indications that the geographic extent and prevalence of benthic cyanobacterial mats are progressively increasing, this review first summarises the factors that may be facilitating this phenomenon. We subsequently evaluate the associated ecological and social implications, and finally identify potential management strategies and future research priorities. An improved understanding of the dynamics behind the proliferation of benthic cyanobacterial mats is critical to shed some light on the factors influencing the probability of recovery toward coral dominance vs. degradation toward alternative states. Though important research gaps remain, this review provides tangible evidence that this emerging benthic phenomenon requires more attention from researchers, managers, and policy-makers.
Factors Promoting Cyanobacterial Mat Proliferation on Reefs
Microorganisms are becoming increasingly abundant on reefs, with a global analysis showing a positive correlation of cell abundances with algal cover (i.e., with reef degradation), stimulated by increased labile dissolved organic carbon (DOC) production (Haas et al., 2016). Mat-forming cyanobacteria likely benefit from a variety of additional inherent properties. Whilst growth rates of most organisms are constrained within a given and often narrow range of conditions, cyanobacterial mats display characteristics that allow them to thrive under a multitude of environmental settings. For example, cyanobacteria species themselves have versatile metabolic capabilities, switching between auto-, hetero-, and mixotrophy (Rippka, 1972). Not only does the physiological and trophic plasticity of mats (Echenique-Subiabre et al., 2015) provide them with a distinct advantage over most benthic organisms, but environmental changes associated with anthropogenic impacts often further favour their proliferation.
Cyanobacteria can dominate algal turf assemblages (Fricke et al., 2011), and are commonly found as epiphytes seeking refuge among complex fleshy algae such as Halimeda which are robust to, and may in fact benefit from, their presence (Barott et al., 2011; Hensley et al., 2013). Consequently, as the integrity of reefs worldwide is compromised by global environmental change, increasing prevalence of algal turfs and other hosts of cyanobacterial epiphytes may provide an important source of, and substrate for, cyanobacteria from which mats can develop. Reef degradation also generates newly available substrate that is easily colonisable by fast-growing cyanobacterial mats (e.g., mats can cover up to 30 km2 within 2–3 months; Albert et al., 2005). Even on a reef where space is highly limited, cyanobacterial mats can overcome this constraint by growing directly over living organisms such as scleractinian corals and fleshy algae (Ritson-Williams et al., 2005; Puyana and Prato, 2013; de Bakker et al., 2016, 2017). Besides benefitting from newly available space and reduced health of other benthic organisms with ongoing reef degradation, benthic cyanobacterial mats can profit directly from increased temperatures and unusual rainfall patterns (Paul, 2008; O'Neil et al., 2012). Additionally, increasingly acidic oceans may stimulate marine cyanobacteria which have high photosynthetic demands (Levitan et al., 2007). Some cyanobacteria exhibit high genetic adaptability to changes in carbon availability, and are able to utilise bicarbonate ions (which increase with ocean acidification) as a carbon source (Badger and Price, 2003; Paerl and Paul, 2012; Sandrini et al., 2016; Visser et al., 2016). Recent experimental evidence found ocean warming in isolation, and when combined with low pH, facilitates proliferation of benthic cyanobacteria relative to algal turfs (Ullah et al., 2018). Similarly, a relative increase of mat-forming cyanobacteria within algal turfs under a low pH and high temperature treatment provides further experimental evidence that future ocean conditions may favour benthic cyanobacterial mat expansion (Bender et al., 2014, but see Hassenrück et al., 2016). On the other hand storms, which will also increase in intensity in the coming decades, may dislodge and remove benthic cyanobacterial mats (Becerro et al., 2006).
Rapidly growing human populations on tropical coastlines are also having a profound effect on coastal marine environments locally through increased sewage input and nutrient runoff driven by land-use change and agricultural development (Burke et al., 2012). Benthic cyanobacterial mats are able to compete for nitrogen when it is available but can also circumvent nitrogen limitation in nitrogen-deficient waters (Paerl, 2008). Although the ability of marine cyanobacteria to fix nitrogen can indeed vary across species (Zehr, 2011), growth of common mat-forming types is not promoted by nitrogen input to the same extent as by iron or phosphorous (Ahern et al., 2007). This implies that the concentrations of other nutrients are relatively more important in controlling cyanobacterial growth.
Because of the ability of many mat-forming cyanobacteria to fix nitrogen when it is limiting, phosphorous enrichment that shifts a system from phosphorous to nitrogen limitation (i.e., transitions from high to low N:P ratio) likely favours growth of cyanobacteria over other primary producers (Figure 3; Schindler, 1977; Kuffner and Paul, 2001). Whilst reefs are often phosphorous limited (Lapointe, 1997; Rosset et al., 2017), this nutrient can enter tropical reef systems via wastewater discharge, aquaculture, and terrestrial runoff from urbanised and agricultural land (Figure 3). Sewage input for instance can strongly shift the canonical Redfield ratio from 16:1 (N:P) to <10 (Lapointe et al., 2005), and has been linked to proliferation of benthic cyanobacterial mats (Ford et al., 2017). Furthermore, occurrence of mats during the rainy season at a site in Curaçao coincided with N:P ratios dropping from 50 to 16 (Brocke et al., 2015a; den Haan et al., 2016). Alongside direct input from terrestrial sources, one pathway recognised to increase levels of phosphorous is release from surface sediments of the marine benthos, where phosphorous is usually bound with stable insoluble iron oxyhydroxides within the oxidised layer (Sundby et al., 1992; Rose and Waite, 2005). Following high organic matter (OM) input (e.g., from sewage) and/or low dissolved oxygen levels at the water-sediment interface, surface sediments become anoxic, leading to iron (III) reduction to bioavailable iron (II), and in turn releasing reactive phosphate and iron (Figure 3; Jensen et al., 1995; Brocke et al., 2015a; Hanington et al., 2016). A similar mechanism likely applies following phytoplankton blooms (caused by eutrophication) which decay and produce particulate OM which settles onto the reef floor. This potentially explains a prolonged shift toward cyanobacterial mats alongside mortality of reef-associated organisms following an extensive phytoplankton bloom (>20 km2) observed within a Solomon Islands lagoon (Albert et al., 2012).
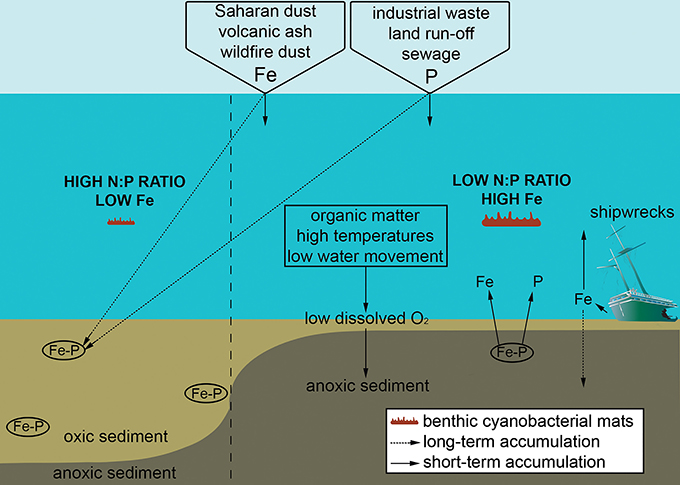
Figure 3. Schematic diagram representing how sedimentary changes support the growth of benthic cyanobacterial mats, focusing on the roles of low dissolved oxygen (O2) at the sediment-water interface leading to sediment anoxia and subsequent release of sedimentary iron (Fe) and phosphate (P). Some main external sources of Fe and P are included, with long-term accumulation within sediments and short-term accumulation in the water column differentiated.
In addition to reactive phosphate, the associated sedimentary release of bioavailable iron (Figure 3) is likely important for mat development. Iron concentrations differ regionally with the degree of exposure to Aeolian dust, ash from volcanic eruptions, emergent basaltic rock associated with past volcanic activity, shipwrecks, dust from wildfires and terrestrial run-off (Abram et al., 2003; Kelly et al., 2012; Roff and Mumby, 2012; Schils, 2012). At areas not naturally exposed to iron from Aeolian dust such as the Pacific, benthic cyanobacterial mats establish quickly following supply of iron to the water column, for instance, through volcanic ash (Schils, 2012). Furthermore, ship groundings on strongly iron-limited reefs in the central Pacific have facilitated long-lasting shifts toward “black reefs” dominated by mixed benthic assemblages of cyanobacteria, turf and fleshy algae, and corallimorphs (Schroeder et al., 2008; Kelly et al., 2012). Where sediments contain iron, growth of cyanobacterial mats can be stimulated following the reduction and release of iron when sediments become anoxic following flooding and/or phytoplankton blooms, as observed at Moreton Bay, Australia (Ahern et al., 2006, 2007, 2008; Hanington et al., 2016). As well as controlling cyanobacterial growth, iron is critical for the nitrogenase enzyme which controls nitrogen fixation (Berman-Frank et al., 2001; Mills et al., 2004; Ahern et al., 2008). Given that cyanobacterial mats grow rapidly following the addition of iron in areas that are naturally iron-limited, and that iron binds readily to phosphate in sediments (thus linked to another key nutrient), iron availability is likely a key factor determining the proliferation of cyanobacterial mats.
Top-down control of benthic cyanobacterial mats is often restricted due to their chemical defences which reduce palatability and deter grazing even where herbivorous fish are abundant (Thacker et al., 1997; Capper et al., 2006a,b, 2016), though it must be noted that data are limited to well-studied species in few locations. Lyngbyatoxin-A is the most extensively researched secondary metabolite in marine benthic cyanobacteria, and although its production varies across different locations, it can have community-wide consequences (Paul et al., 2007). The cover of cyanobacterial mats does not increase, and can even decrease, in the absence of herbivorous fish (i.e., inside herbivore exclusion cages—Wanders, 1977; Thacker et al., 2001). A 3-year experiment in Florida observed distinct summer blooms of benthic cyanobacteria under nutrient enrichment in the presence of herbivores, weaker blooms when nutrient enrichment was combined with herbivore exclusion, and no blooms under herbivore exclusion alone where fleshy algae such as Turbinaria, Sargassum, and Hypnea were relatively more dominant (Zaneveld et al., 2016). These findings suggest that nominally herbivorous fish cannot control the development of cyanobacterial mats, or that high levels of herbivory are in fact necessary for cyanobacteria to maintain dominance over fleshy algae. Some mesograzers and a few reef fish species have been documented to graze on mats directly or on foods experimentally coated in their secondary metabolite extracts (Table 2). In contrast to most studies, a recent review by Clements et al. (2016) proposed that parrotfishes actually target endo- and epilithic cyanobacteria, and provides evidence of direct consumption of mats by Bolbometopon muricatum. The degree to which fishes feed selectively on cyanobacteria may depend on their life stage (Paul et al., 1990), exposure time, and access to alternative food (Thacker et al., 1997). Interestingly, growth rates of benthic cyanobacterial mats on soft sediments are significantly reduced in the presence of sea cucumbers both in aquaria (Uthicke, 1999; Michio et al., 2003) and in situ (Moriarty et al., 1985). Within enclosures containing sea cucumbers in Madagascar, abundance of bacteria and concentration of photosynthetic microorganisms within surface sediments were 50 and 22% lower compared with controls (Plotieau et al., 2013). The expansion of cyanobacterial mats may be limited in the presence of sea cucumbers because of direct consumption (e.g., Sournia, 1976), and bioturbation of benthic sediments. Bioturbation of sediments by sea cucumbers can increase the thickness of the oxic sediment layers under increased temperatures, nutrients, and OM loads (Mactavish et al., 2012; Lee et al., 2017), thus contributing to the continued persistence of healthy sediment-associated microbial communities and sediment integrity. Overexploitation of sea cucumbers across the Indo-Pacific (Anderson et al., 2011) and associated changes in the sediment might thus contribute at least partially to the increasing prevalence of cyanobacterial mats in coastal (sandy) habitats.
Linking Cyanobacterial Mats to Reef Degradation
Recent research on coral reef ecosystem dynamics has primarily focused on the factors driving benthic community shifts whereby reef-building corals are replaced by alternative organisms. Most commonly, coral-dominated reefs shift to systems where fleshy algae establish and become dominant (e.g., Hughes, 1994), but shifts to soft corals, sponges, and algal turfs may also occur (Norström et al., 2009; Jouffray et al., 2015; Smith et al., 2016). As mentioned previously, under scenarios where reefs become dominated by algae, increased labile DOC production stimulates growth of microorganisms (Haas et al., 2016). However, instances in which coral- or algal-dominated reefs shift to systems dominated by benthic cyanobacterial mats have received comparatively little attention. Mats can be ephemeral symptoms of recent reef degradation events resulting in freed space (Schroeder et al., 2008), but can also push stressed systems toward alternative stable states (Albert et al., 2012). For example, elevated nitrogen fixation rates on recently bleached corals or dead coral skeletons imply that cyanobacteria are important colonisers of available substrate and that their nitrogen fixation activity may be sufficient to direct a stressed system toward algal dominance (Davey et al., 2008; Holmes and Johnstone, 2010). The proliferation of benthic cyanobacterial mats on coral reefs has serious direct and indirect effects on numerous reef organisms and ecological processes. Some mats overgrow and smother benthic organisms, including scleractinian corals and fleshy algae (Ritson-Williams et al., 2005; Puyana and Prato, 2013; de Bakker et al., 2016, 2017). Subsequent tissue necrosis of overgrown organisms can occur as a result of oxygen deficiency, contact with allelopathic chemicals, tissue abrasion, or light reduction (Puyana and Prato, 2013). The impact of cyanobacterial mats on localised bleaching and mortality of coral colonies can indeed exceed that of other competitors such as fleshy algae (Titlyanov et al., 2007).
The presence of cyanobacterial mats also directly impairs coral recruitment which is an essential ecological process for reef recovery following disturbances (McClanahan et al., 2012). Recruitment success may be reduced by (i) coral larvae avoiding settling near to cyanobacteria due to negative settlement cues, or (ii) cyanobacteria killing newly settled corals (Kuffner and Paul, 2004; Ritson-Williams et al., 2016). Benthic cyanobacterial mats have been implicated in reducing the recruitment success of both broadcast spawning and brooding corals (Kuffner and Paul, 2004; Kuffner et al., 2006). Importantly, mats can bloom at the same time of year when corals spawn. For instance, in Fiji, both broadcast spawning and cyanobacterial mats occur simultaneously during the warmest mid-summer months (Quinn and Kojis, 2008; Victor Bonito, pers. comm., 2016). Similarly, in Curaçao, spawning takes place in September/October (Van Veghel, 1994), and mats are particularly prevalent in October/November (Brocke et al., 2015a) when the coral spat would be settling on the substrate. At this critical time, the effects of cyanotoxins on coral recruits are also strongly exacerbated by warmer temperatures (Ritson-Williams et al., 2016). Further studies into the temporal prevalence of mats together with information on coral spawning times are needed to assess the extent of this potential threat, as large-scale inhibition of coral recruitment by cyanobacterial mats could have severe implications for the replenishment of coral populations.
The health of adult coral colonies can also be indirectly affected by the development of benthic cyanobacterial mats, for example from increasing levels of bioavailable nitrogen (see introduction—Cardini et al., 2014). Stress may also result from increasing DOC, as mats not only profit from DOC input, but are themselves responsible for releasing high amounts of DOC. For example mats, fleshy algae and algal turfs in Curaçao released 0.59, 0.04, and 0.11 mmol C m−2 h−1, respectively, while corals and bare sediments did not exhibit net DOC production over 24 h (Brocke et al., 2015b). Such amounts may be regarded insignificant when considering the coverage of benthic cyanobacterial mats on healthy reefs (e.g., around 1% of the benthos—Charpy et al., 2010; Bednarz et al., 2015), but are substantial when mats become dominant (e.g., over 50% of the benthos - Thacker and Paul, 2001). Both DOC and bioavailable nitrogen can shift the balance of the benthic community by favouring fast-growing primary producers (e.g., fleshy algae) over scleractinian corals. Island-wide benthic surveys in Curaçao indicated that the abundance of benthic cyanobacterial mats was indeed positively correlated with fleshy algae and negatively correlated with scleractinian corals (Brocke et al., 2015a). At the organism-scale, increased DOC stimulates the activity of coral-associated microbes within the coral mucus, potentially leading to coral tissue hypoxia and subsequent mortality (Kline et al., 2006; Smith et al., 2006). Bacteria within the coral mucus exhibiting the strongest growth responses to DOC are often pathogenic (Morrow et al., 2011). Moreover, during night-time fermentation, certain cyanobacteria (e.g., Oscillatoria, Heyer and Krumbein, 1991) release easily degradable compounds including lactate, ethanol and acetate, which could further enhance heterotrophic metabolism and pathogenic microbes (Haas et al., 2013). Coral (massive Porites) margins in contact with benthic cyanobacteria are characterised by a thick diffusive boundary layer and hypoxia at night, which may in turn facilitate cyanobacterial overgrowth of live corals (Jorissen et al., 2016). Lastly, increased levels of bioavailable nitrogen on reefs may lead to increased bleaching (susceptibility) in corals (Wiedenmann et al., 2013; Rädecker et al., 2015; Pogoreutz et al., 2017), resulting in reinforcing feedbacks that continue to favour reef degradation (Figure 4).
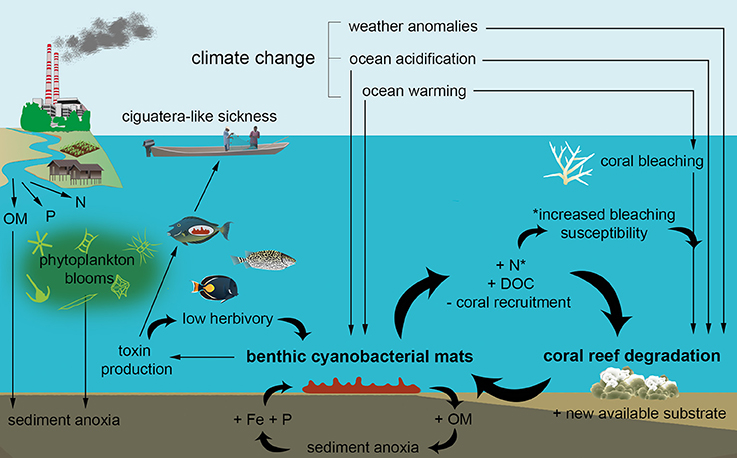
Figure 4. Schematic diagram showing the putative local and global drivers of benthic cyanobacterial mat growth on coral reefs, as well as the associated ecological consequences. Direct positive feedbacks that reinforce reef degradation and benthic mat growth are represented by thick curved arrows. Reef degradation will in turn drive coastal communities increasingly toward land-use changes such as agricultural development, further facilitating nutrient release and reinforcing the cycle. Abbreviations within the figure: N, nitrogen; DOC, dissolved organic carbon; Fe, iron; OM, organic matter; P, phosphate.
Recent evidence from the Caribbean suggests that benthic cyanobacterial mats may not be limited to shallow reef environments and can occur at depths of up to 40 m (de Bakker et al., 2017). Though the ability of marine benthic cyanobacteria to grow under reduced light remains relatively unknown, cyanobacteria (e.g., Planktothrix) have been identified as the group within phytoplankton communities which can grow best under low light conditions (Mur et al., 1999). Such findings could challenge the potential for the “deep reef refugia hypothesis” (Bongaerts et al., 2010), which proposes that deeper corals that are less affected by heat and light stress could restock degraded shallow areas in the future through vertical genetic connectivity, underlining the importance of further information on the ecology of mesophotic benthic cyanobacteria.
Cyanobacterial mats can also affect reef fish communities. Recent experimental data indicated that climate change-driven shifts toward benthic cyanobacteria could ultimately lead to food web collapse due to reduced energy flow to higher trophic levels (Ullah et al., 2018). A major die-off of juvenile rabbitfish Siganus argenteus and Siganus spinus occurred as mats became dominant on coral reefs around Guam, which was attributed to starvation (Nagle and Paul, 1998). Furthermore, aquaria experiments revealed that the rabbitfish Siganus fuscescens chose to starve rather than to consume Moorea producens containing lyngbyatoxin-A (Capper et al., 2006b). Contrastingly, proliferation of benthic cyanobacterial mats following a ship grounding at Rose Atoll, American Samoa, led to long-term increases in the local abundance and biomass of herbivorous fish (Green et al., 1997; Schroeder et al., 2008). These observations may be due to the cyanobacteria being mixed with algal turfs and/or the additional shelter offered by the wreckage.
“Social-Ecological Traps” Associated with Cyanobacterial Mats
Benthic marine cyanobacteria may pose direct threats to human health, similar to their freshwater counterparts (e.g., Bell and Codd, 1994). Summertime blooms of benthic cyanobacterial mats on some New Caledonian inshore reefs have been identified as the causative agents of ciguatera-like disease outbreaks in coastal human populations (Laurent et al., 2008). Symptoms included (clinically similar to ciguatera) gastrointestinal disorders, fatigue, limb and joint pain, reversal of hot and cold sensations, and some cardiovascular symptoms. In fact the sickness seemed to manifest in more severe symptoms than ciguatera, with one third of sufferers being hospitalised which was far higher than usual in the area. Instead of containing the common ciguatera causative agent (i.e., the dinoflagellate Gambierdiscus), these mats were dominated by Hydrocoleum, a common cyanobacterium within tropical mats (Abed et al., 2006; Echenique-Subiabre et al., 2015). Hydrocoleum was subsequently found to produce cyanotoxins with very similar characteristics to cigua- and paralysing-toxins (Laurent et al., 2008). Several fish species, some of which are prime targets of fisheries, carried the cyanotoxins, and giant clams were also intoxicated (see Méjean et al., 2010, Table 2). A subsequent study encompassing New Caledonia, French Polynesia and Vanuatu confirmed that the link between benthic cyanobacterial mats and ciguatera-like sickness is rather widespread in the Pacific (Laurent et al., 2012). An estimated 500,000 Pacific islanders have suffered from ciguatera symptoms between 1973 and 2008, with a 60% increase in documented cases between 1973-1983 and 1998-2008 (Skinner et al., 2011), underlining the urgency of identifying management practices that can control the causal organisms. Bathers and fishers exposed to coastal waters where benthic cyanobacterial mats are common have also often reported severe dermatitis and asthma-like symptoms (Osborne et al., 2001).
Rapidly growing coastal populations will continue to drive increased nutrient and OM input into coastal waters over the coming decades. In the absence of wastewater treatment and land-use management, poor water quality could facilitate benthic cyanobacterial mat growth which will likely be magnified under future climate change. Combined, the stressful environmental conditions and development of benthic cyanobacterial mats can negatively impact the health of coral-dominated systems. Together with the inhibition of coral recruitment, ecological feedbacks favouring cyanobacterial growth and reef degradation could be further strengthened by increasing bioavailable nitrogen and DOC (Figure 4). Degraded reefs have reduced structural complexity, impairing their capacity to protect shorelines from wave energy (Sheppard et al., 2005) and to sustain productive fisheries (Graham and Nash, 2013; Pratchett et al., 2014). The accumulation of ciguatera-like toxins in fishes in connection with cyanobacterial mats may also pose limits to the utilisation of fishery resources. As human communities are faced with progressively degraded coastal resources, they may increasingly resort to terrestrial resources such as agriculture, facilitating further reductions in coastal water quality from increased nutrient run-off. These practices can promote a social-ecological trap with self-reinforcing feedbacks as has been similarly described for reefs that become dominated by fleshy algae (Cinner, 2011). Escape routes to shift algal-dominated systems back toward a more desirable state include adaptive fisheries management (Mumby et al., 2006; Hughes et al., 2007; Cinner, 2011), and where positive feedbacks are particularly strong additional “shock” events (e.g., storms) can be necessary to reset the system by clearing space for coral recruits to settle (Edmunds and Carpenter, 2001; Graham et al., 2013). However, where mechanisms that facilitate mat proliferation are in place, fast-growing cyanobacteria could rapidly pre-empt space even where herbivorous fishes are abundant, thus truncating coral recruitment. As local fishing regulations alone may be insufficient to counteract cyanobacterial proliferation, escaping a social-ecological trap whereby cyanobacterial mats have developed extensively may be very challenging. These feedbacks may call for integrated efforts to regulate appropriate fisheries and manage watersheds.
Avoiding Reefs Slipping to Slime
Coral reefs and their associated ecosystem services would be better conserved if environmental conditions facilitating development and prevalence of benthic cyanobacterial mats can be prevented. In concert with global actions, an assortment of relatively cost-effective local management practices (e.g., Richmond et al., 2007; Klein et al., 2010) may reduce the likelihood and temporal duration of mats. In particular, mat development seems to be facilitated when systems enter a low N:P ratio and when iron concentrations increase, implying that managers should reduce the level of phosphate and iron of terrestrial origin entering reefs. This could be achieved by limiting the use of certain fertilisers, reducing soil erosion, and protecting mangroves. Furthermore, studies have shown that nutrient and OM input can trigger the release of iron and phosphate following oxygen depletion within sediments (Brocke et al., 2015a; Hanington et al., 2016). Where iron is already present in sediments (e.g., in volcanic areas, reefs exposed to Aeolian dust), targeted management of nutrient and OM input may thus be particularly important. Sewage is a major source of both nutrients and OM, and just as benthic cyanobacterial mats can increase significantly close to a point of sewage input, improved sewage treatment can reverse mats to grazable algal turfs, as shown in Barbados (DeGeorges et al., 2010). In addition to limiting growth of cyanobacterial mats (and unfavourable algae), improvements in water quality could reduce the otherwise stimulating effects of increasing carbon dioxide and temperature on cyanobacterial growth (Visser et al., 2016), thus indirectly reducing the effects of climate change which cannot be tackled at the local level. Furthermore, managing reefs with an integrated approach to build resilience to climate change impacts (e.g., McClanahan et al., 2012) may be beneficial in promoting resilience against the initiation and establishment of cyanobacterial mats after disturbances.
Current knowledge implies that management of reef fish communities alone could be futile in counteracting benthic cyanobacterial mats. However, following a shift toward a cyanobacteria–algal turf mixed assemblage following a ship grounding at Rose Atoll, biomass of some surgeonfish and parrotfish species showed a counterintuitive increase (Green et al., 1997; Schroeder et al., 2008). In situ video observations aimed at quantifying herbivory on mats may identify novel opportunistic species, similar to the unexpected finding that batfish act as key herbivores following blooms of fleshy Sargassum algae (Bellwood et al., 2006). Such knowledge could support protective measures aimed at regulating the fishery of important consumers of cyanobacteria where mats are becoming increasingly prevalent. For example, B. muricatum has been observed directly feeding on mats (Clements et al., 2016), adding to the body of evidence that this heavily targeted species warrants special protection where it is threatened (e.g., Bellwood et al., 2003). Herbivorous fish species may vary greatly in their sensitivity to cyanotoxins, and prior or repeated exposure may even allow for some adaptation (Thacker et al., 1997; Capper et al., 2006a). Trade-offs between growth and defence drive differential anti-herbivore toxin production in fleshy algae across areas with different water qualities (Van Alstyne and Pelletreau, 2000). Although specific studies are currently scarce, cyanotoxin production usually increases with conditions that favour cyanobacterial growth, such as increased temperatures (Kaebernick and Neilan, 2001). A combined approach incorporating toxin production and functional genes could investigate how toxin production varies across environmental gradients and whether this results from changes in mat composition or functional gene expression (Golubic et al., 2010; Echenique-Subiabre et al., 2015). Such patterns would suggest predictable differences in cyanobacteria palatability with water quality management or over environmental gradients.
Considering the potentially limited control of benthic cyanobacterial mats by reef fishes, management policies may have to pertain to more than herbivorous fishes and explicitly address other organisms capable of hindering mat proliferation. One example of such an organism in sandy environments (e.g., reef flats, lagoons, patch reefs) is sea cucumbers. Their bioturbation activity may moderate the development of anoxic sediment conditions (particularly under additional heat stress—Lee et al., 2017) that could otherwise facilitate the release of iron and phosphate (Brocke et al., 2015a). Experimental manipulations should quantify densities of sea cucumbers that are necessary to maintain sediment integrity under declining water quality and ocean warming scenarios. Whilst many mesograzers (e.g., sea hares) recognised to directly consume cyanobacteria likely have a relatively small impact on large-spread mats, their potential to regulate mat growth should be further explored alongside other larger grazing species. For example, the generalist sea urchin Diadema seems undeterred by cyanobacterial secondary metabolites (Capper et al., 2016), and perhaps the loss of this species throughout the Caribbean has helped facilitate the proliferation of mats across the region.
Research attention should be directed to clarifying the role of benthic cyanobacterial mats as symptoms vs. drivers of reef degradation. Benthic monitoring surveys need to improve their capacity to distinguish benthic cyanobacterial mats from other benthic groups, and whenever possible different cyanobacterial morphotypes should be recorded separately. Samples should be collected for subsequent genetic analyses to better clarify the taxonomic diversity within mats. This increased level of detail in benthic surveys will allow for the detection of changes in benthic cyanobacterial communities, in turn providing critical information on their potential as a bioindicator of coral reef degradation. A study on Southern Caribbean reefs in Colombia, for instance, suggested that >15% benthic cover of cyanobacteria indicates degradation (Puyana et al., 2015). As overgrowth does not always lead to the death of the underlying organism, studies should identify the characteristics of coral-cyanobacteria contacts. Detailed chemical characterisation of cyanobacterial mats is also important to predict future outbreaks of ciguatera-like sickness (Laurent et al., 2008, 2012). Concurrently, to improve the understanding of the link between human health and cyanobacterial mats, samples of mats and seafood should be collected for identification to assess human pathogenicity where significant cyanobacteria cover is observed.
Future Research
We identify four key research areas that present tangible opportunities for novel future research. First, to prioritise local actions that minimise development and persistence of cyanobacterial mats, the relative contributions of different components of global environmental change on cyanobacterial mat prevalence and toxicity require further experimental testing, as well as improved information on the occurrence and extent of marine benthic cyanobacteria. Secondly, opportunistic consumption of cyanobacterial mats by key functional groups of herbivores such as reef fishes and echinoderms needs to be explored, and species warranting special conservation policies should be identified where mats are prevalent. Thirdly, experiments can determine which densities of sea cucumbers can effectively decrease the formation of cyanobacterial mats to inform managers of minimum stock sizes and harvest quotas. Lastly, the relative importance of benthic cyanobacterial mats as a driver or symptom of coral reef degradation needs to be better understood. To achieve these four goals, mats need to be reliably and consistently identified by monitoring programmes and researchers, and no longer grouped together with algal types or abiotic substrate. New developments in the use of molecular tools, such as DNA barcoding, may provide important tools in this regard. Once this is achieved, the temporal and spatial scale of such surveys can be expanded to better understand the ephemeral or stable nature of mats at different areas, to verify putative drivers and controls, and to better link the occurrence of benthic cyanobacterial mats to the biology and ecology of other reef organisms, such as stony corals.
Concluding Remarks
While an increase in awareness of the presence of benthic cyanobacterial mats may account partially for the recent increase of reports, they appear to be important players in coral reef degradation. The proliferation and establishment of these mats carries important implications for both ecosystem and human health. A wealth of factors are facilitating mat development and persistence, including increasing availability of space on degrading reefs and declining water quality. Projected climate change conditions will likely favour growth of cyanobacterial mats while being detrimental to other benthic reef organisms. Systems where human populations and reefs are closely linked and that experience cyanobacterial blooms may be particularly prone to enter social-ecological traps with strong positive feedbacks. Not only can these traps threaten ecosystem services and human wellbeing, but they can be very challenging to escape from. Considering the serious risk that benthic cyanobacterial blooms pose directly to the future of reefs and their associated ecosystem services as well as human health, further focused research and resources for this topic are important.
Author Contributions
AF proposed the original idea of this review. AF, MN, PV, SB, SA, and SF each then contributed to developing the concept of the review. AF developed the initial draft, and MN, PV, SB, SA, and SF critically revised the draft. Following AF's inclusion of revisions, MN, PV, SB, SA, and SF approved the final version for submission. AF, MN, PV, SB, SA, and SF all agree to be accountable for all aspects of the work.
Funding
AF, SB, and SF would like to thank the (German) Federal Ministry of Education and Research (BMBF) for funding through the “Nachwuchsgruppen Globaler Wandel 4 + 1” (REPICORE, grant number 01LN1303A).
Conflict of Interest Statement
The authors declare that the research was conducted in the absence of any commercial or financial relationships that could be construed as a potential conflict of interest.
Acknowledgments
AF, SB, and SF would like to thank the (German) Federal Ministry of Education and Research (BMBF) for funding through the “Nachwuchsgruppen Globaler Wandel 4 + 1” (REPICORE, grant number 01LN1303A). We would also like to thank the two reviewers for their constructive input. A version of this manuscript has already been published online within the lead author's doctoral dissertation (Ford, 2017).
References
Abed, R. M. M., Al-Thukair, A., and de Beer, D. (2006). Bacterial diversity of a cyanobacterial mat degrading petroleum compounds at elevated salinities and temperatures. FEMS Microbiol. Ecol. 57, 290–301. doi: 10.1111/j.1574-6941.2006.00113.x
Abram, N. J., Gagan, M. K., McCulloch, M. T., Chappell, J., and Hantoro, W. S. (2003). Coral reef death during the 1997 indian ocean dipole linked to Indonesian wildfires. Science 301, 953–955. doi: 10.1126/science.1083841
Ahern, K. S., Ahern, C. R., and Udy, J. W. (2007). Nutrient additions generate prolific growth of Lyngbya majuscula (cyanobacteria) in field and bioassay experiments. Harmful Algae 6, 134–151. doi: 10.1016/j.hal.2006.08.004
Ahern, K. S., Ahern, C. R., and Udy, J. W. (2008). In situ field experiment shows Lyngbya majuscula (cyanobacterium) growth stimulated by added iron, phosphorus and nitrogen. Harmful Algae 7, 389–404. doi: 10.1016/j.hal.2007.08.006
Ahern, K. S., O'Neil, J. M., Udy, J. W., and Albert, S. (2006). Effects of iron additions on filament growth and productivity of the cyanobacterium Lyngbya majuscula. Mar. Freshwater Res. 57, 167–176. doi: 10.1071/MF05022
Albert, S., Dunbabin, M., and Skinner, M. (2012). “Benthic shift in a solomon island's lagoon: corals to cyanobacteria,” in Proceedings of the 12th International Coral Reef Symposium 2012 (Cairns, QLD), 9–13.
Albert, S., O'Neil, J. M., Udy, J. W., Ahern, K. S., O'Sullivan, C. M., and Dennison, W. C. (2005). Blooms of the cyanobacterium Lyngbya majuscula in coastal Queensland, Australia: disparate sites, common factors. Mar. Pollut. Bull. 51, 428–437. doi: 10.1016/j.marpolbul.2004.10.016
Anderson, S. C., Flemming, J. M., Watson, R., and Lotze, H. K. (2011). Serial exploitation of global sea cucumber fisheries. Fish Fish. 12, 317–339. doi: 10.1111/j.1467-2979.2010.00397.x
Badger, M. R., and Price, G. D. (2003). CO2 concentrating mechanisms in cyanobacteria: molecular components, their diversity and evolution. J. Exp. Bot. 54, 609–622. doi: 10.1093/jxb/erg076
Barott, K. L., Rodriguez-Brito, B., Janouškovec, J., Marhaver, K. L., Smith, J. E., Keeling, P., et al. (2011). Microbial diversity associated with four functional groups of benthic reef algae and the reef-building coral Montastrea annularis. Environ. Microbiol. 13, 1192–1204. doi: 10.1111/j.1462-2920.2010.02419.x
Becerro, M. A., Bonito, V., and Paul, V. J. (2006). Effects of monsoon-driven wave action on coral reefs of Guam and implications for coral recruitment. Coral Reefs 1, 193–199. doi: 10.1007/s00338-005-0080-7
Bednarz, V., van Hoytema, N., Cardini, U., Naumann, M., Al-Rshaidat, M., and Wild, C. (2015). Dinitrogen fixation and primary productivity by carbonate and silicate reef sand communities of the Northern Red Sea. Mar. Ecol. Prog. Ser. 527, 47–57. doi: 10.3354/meps11224
Bell, S. G., and Codd, G. A. (1994). Cyanobacterial toxins and human health. Rev. Med. Microbiol. 5, 256–264.
Bellwood, D. R., Hoey, A. S., and Choat, J. H. (2003). Limited functional redundancy in high diversity systems: resilience and ecosystem function on coral reefs. Ecol. Lett. 6, 281–285. doi: 10.1046/j.1461-0248.2003.00432.x
Bellwood, D. R., Hughes, T. P., and Hoey, A. S. (2006). Sleeping functional group drives coral reef recovery. Curr. Biol. 16, 2434–2439. doi: 10.1016/j.cub.2006.10.030
Bender, D., Diaz-Pulido, G., and Dove, S. (2014). Warming and acidification promote cyanobacterial dominance in turf algal assemblages. Mar. Ecol. Prog. Ser. 517, 271–284. doi: 10.3354/meps11037
Berman-Frank, I., Lundgren, P., Chen, Y.-B., Küpper, H., Kolber, Z., Bergman, B., et al. (2001). Segregation of nitrogen fixation and oxygenic photosynthesis in the marine cyanobacterium Trichodesmium. Science 294, 1534–1537. doi: 10.1126/science.1064082
Bongaerts, P., Ridgway, T., Sampayo, E. M., and Hoegh-Guldberg, O. (2010). Assessing the “deep reef refugia” hypothesis: focus on Caribbean reefs. Coral Reefs 29, 309–327. doi: 10.1007/s00338-009-0581-x
Brocke, H. J. (2013). Little Things Become Big: Drivers and Impacts of Benthic Cyanobacterial Blooms on Coral Reefs. Ph.D., dissertation, University of Bremen, Germany.
Brocke, H. J., Polerecky, L., de Beer, D., Weber, M., Claudet, J., and Nugues, M. M. (2015a). Organic matter degradation drives benthic cyanobacterial mat abundance on Caribbean coral reefs. PLoS ONE 10:e0125445. doi: 10.1371/journal.pone.0125445
Brocke, H. J., Wenzhoefer, F., de Beer, D., Mueller, B., van Duyl, F. C., and Nugues, M. M. (2015b). High dissolved organic carbon release by benthic cyanobacterial mats in a Caribbean reef ecosystem. Sci. Rep. 5:8852. doi: 10.1038/srep08852
Brocks, J. J., Logan, G. A., Buick, R., and Summons, R. E. (1999). Archean molecular fossils and the early rise of eukaryotes. Science 285, 1033–1036. doi: 10.1126/science.285.5430.1033
Burke, L., Reytar, K., Spalding, M., and Perry, A. (2012). Reefs at Risk Revisited. Washington, DC: World Resources Institute.
Capper, A., Cruz-Rivera, E., Paul, V. J., and Tibbetts, I. R. (2006b). Chemical deterrence of a marine cyanobacterium against sympatric and non-sympatric consumers. Hydrobiologia 553, 319–326. doi: 10.1007/s10750-005-1129-x
Capper, A., Erickson, A. A., Ritson-Williams, R., Becerro, M. A., Arthur, K. A., and Paul, V. J. (2016). Palatability and chemical defences of benthic cyanobacteria to a suite of herbivores. J. Exp. Mar. Biol. Ecol. 474, 100–108. doi: 10.1016/j.jembe.2015.09.008
Capper, A., Tibbetts, I. R., O'Neil, J. M., and Shaw, G. R. (2006a). Feeding preference and deterrence in rabbitfish Siganus fuscescens for the cyanobacterium Lyngbya majuscula in Moreton Bay, south-east Queensland, Australia. J. Fish Biol. 68, 1589–1609. doi: 10.1111/j.0022-1112.2006.01048.x
Cardini, U., Bednarz, V. N., Foster, R. A., and Wild, C. (2014). Benthic N2 fixation in coral reefs and the potential effects of human-induced environmental change. Ecol. Evol. 4, 1706–1727. doi: 10.1002/ece3.1050
Cardini, U., Bednarz, V. N., van Hoytema, N., Rovere, A., Naumann, M. S., Al-Rshaidat, M. M., et al. (2016). Budget of primary production and dinitrogen fixation in a highly seasonal Red Sea coral reef. Ecosystems 19, 771–785.
Casareto, B. E., Charpy, B., Langlade, M. J., Suzuki, T., Ohba, H., Niraula, M., et al. (2008). “Nitrogen fixation in coral reef environments,” in Proceedings of the 11th International Coral Reef Symposium 2012 (Fort Lauderdale), 890–894.
Charpy, L., Alliod, R., Rodier, M., and Golubic, S. (2007). Benthic nitrogen fixation in the SW New Caledonia lagoon. Aquat. Microb. Ecol. 47, 73–81. doi: 10.3354/ame047073
Charpy, L., Casareto, B. E., Langlade, M. J., and Suzuki, Y. (2012a). Cyanobacteria in coral reef ecosystems: a review. J. Mar. Biol. 19:259571. doi: 10.1155/2012/259571
Charpy, L., Palinska, K. A., Abed, R. M. M., Langlade, M. J., and Golubic, S. (2012b). Factors influencing microbial mat composition, distribution and dinitrogen fixation in three western Indian Ocean coral reefs. Eur. J. Phycol. 47, 51–66. doi: 10.1080/09670262.2011.653652
Charpy, L., Palinska, K. A., Casareto, B., Langlade, M. J., Suzuki, Y., Abed, R. M. M., et al. (2010). Dinitrogen-fixing cyanobacteria in microbial mats of two shallow coral reef ecosystems. Microb. Ecol. 59, 174–186. doi: 10.1007/s00248-009-9576-y
Cinner, J. E. (2011). Social-ecological traps in reef fisheries. Glob. Environ. Change 21, 835–839. doi: 10.1016/j.gloenvcha.2011.04.012
Clements, K. D., German, D. P., Piché, J., Tribollet, A., and Choat, J. H. (2016). Integrating ecological roles and trophic diversification on coral reefs: multiple lines of evidence identify parrotfishes as microphages. Biol. J. Linn. Soc. 120, 729–751. doi: 10.1111/bij.12914
Cruz-Rivera, E., and Paul, V. J. (2002). “Coral reef benthic cyanobacteria as food and refuge, diversity, chemistry and complex interactions,” Proceedings of the 9th International Coral Reef Symposium 2002 (Bali), 515–520.
Cruz-Rivera, E., and Paul, V. J. (2006). Feeding by coral reef mesograzers: algae or cyanobacteria? Coral Reefs 25, 617–627. doi: 10.1007/s00338-006-0134-5
Davey, M., Holmes, G., and Johnstone, R. (2008). High rates of nitrogen fixation (acetylene reduction) on coral skeletons following bleaching mortality. Coral Reefs 27, 227–236. doi: 10.1007/s00338-007-0316-9
de Bakker, D. M., Meesters, E. H., van Bleijswijk, J. D. L., Luttikhuizen, P. C., Breeuwer, H. J. A. J., and Becking, L. E. (2016). Population genetic structure, abundance, and health status of two dominant benthic species in the Saba Bank National Park, Caribbean Netherlands: Montastraea cavernosa and Xestospongia muta. PloS ONE 11:e0155969. doi: 10.1371/journal.pone.0155969
de Bakker, D. M., van Duyl, F. C., Bak, R. P. M., Nugues, M. M., Nieuwland, G., and Meesters, E. H. (2017). 40 years of benthic community change on the Caribbean reefs of Curaçao and Bonaire: the rise of slimy cyanobacterial mats. Coral Reefs 36, 355–367. doi: 10.1007/s00338-016-1534-9
DeGeorges, A., Goreau, T. J., and Reilly, B. (2010). Land-sourced pollution with an emphasis on domestic sewage: lessons from the Caribbean and implications for coastal development on Indian Ocean and Pacific coral reefs. Sustainability 2, 2919–2949. doi: 10.3390/su2092919
den Haan, J., Huisman, J., Brocke, H. J., Goehlich, H., Latjinhouwers, K. R. W., van Heeringen, S., et al. (2016). Nitrogen and phosphorous uptake rates of different species from a coral reef community after a nutrient pulse. Sci. Rep. 6:28821. doi: 10.1038/srep28821
Duarte, C. M., Fulweiler, R. W., Lovelock, C. E., Martinetto, P., Saunders, M. I., Pandolfi, J. M., et al. (2015). Reconsidering ocean calamities. BioScience 65, 130–139. doi: 10.1093/biosci/biu198
Echenique-Subiabre, I., Villeneuve, A., Golubic, S., Turquet, J., Humbert, J.-F., and Gugger, M. (2015). Influence of local and global environmental parameters on the composition of cyanobacterial mats in a tropical lagoon. Microb. Ecol. 69, 234–244. doi: 10.1007/s00248-014-0496-0
Edmunds, P. J., and Carpenter, R. C. (2001). Recovery of Diadema antillarum reduces macroalgal cover and increases abundance of juvenile corals on a Caribbean reef. Proc. Natl. Acad. Sci. U.S.A. 98, 5067–5071. doi: 10.1073/pnas.071524598
Engene, N., Gunasekera, S. P., Gerwick, W. H., and Paul, V. J. (2013a). Phylogenetic inferences reveal a large extent of novel biodiversity in chemically rich tropical marine cyanobacteria. Appl. Environ. Microb. 79, 1882–1888. doi: 10.1128/AEM.03793-12
Engene, N., Paul, V. J., Byrum, T., Gerwick, W. H., Thor, A., and Ellisman, M. H. (2013b). Five chemically rich species of tropical marine cyanobacteria of the genus Okeania gen. nov. (Oscillatoriales, Cyanoprokaryota). J. Phycol. 49, 1095–1106. doi: 10.1111/jpy.12115
Engene, N., Rottacker, E. C., Kaštovský, J., Byrum, T., Choi, H., Ellisman, M. H., et al. (2012). Moorea producens gen. nov., sp. nov. and Moorea bouillonii comb. nov., tropical marine cyanobacteria rich in bioactive secondary metabolites. Int. J. Syst. Evol. Micr. 62, 1171–1178. doi: 10.1099/ijs.0.033761-0
Fong, P., and Paul, V. J. (2011). “Coral reef algae,” in Coral Reefs: An Ecosystem in Transition, eds Z. Dubinsky and N. Stambler (Dordrecht, NL: Springer), 241–272.
Ford, A. K. (2017). Influences of Pacific Island Human Communities on Benthic Coral Reef Functioning and Resilience. Ph.D., dissertation. University of Bremen, Germany.
Ford, A. K., van Hoytema, N., Moore, B. R., Pandihau, L., Wild, C., and Ferse, S. C. A. (2017). High sedimentary oxygen consumption indicates that sewage input from small islands drives benthic community shifts on overfished reefs. Environ. Conserv. 44, 405–411. doi: 10.1017/S0376892917000054
Fricke, A., Teichberg, M., Beilfuss, S., and Bischof, K. (2011). Succession patterns in algal turf vegetation on a Caribbean coral reef. Bot. Mar. 54, 111–126. doi: 10.1515/bot.2011.021
Golubic, S., Abed, R. M. M., Palińska, K., Pauillac, S., Chinain, M., and Laurent, D. (2010). Marine toxic cyanobacteria: diversity, environmental responses and hazards. Toxicon 56, 836–841. doi: 10.1016/j.toxicon.2009.07.023
Graham, N. A., Bellwood, D. R., Cinner, J. E., Hughes, T. P., Norström, A. V., and Nyström, M. (2013). Managing resilience to reverse phase shifts in coral reefs. Front. Ecol. Environ. 11, 541–548. doi: 10.1890/120305
Graham, N. A. J., and Nash, K. L. (2013). The importance of structural complexity in coral reef ecosystems. Coral Reefs 32, 315–326. doi: 10.1007/s00338-012-0984-y
Green, A. L., Burgett, J., Molina, M., Palawski, D., and Gabrielson, P. (1997). The Impact of a Ship Grounding and Associated Fuel Spill at Rose Atoll National Wildlife Refuge, American Samoa. U.S. Fish and Wildlife Service Report, Honolulu, Hawaii.
Haas, A. F., Fairoz, M. F. M., Kelly, L. W., Nelson, C. E., Dinsdale, E. A., Edwards, R. A., et al. (2016). Global microbialization of coral reefs. Nat. Microbiol. 1:16042. doi: 10.1038/NMICROBIOL.2016.42
Haas, A. F., Nelson, C. E., Rohwer, F., Wegley-Kelly, L., Quistad, S. D., Carlson, C. A., et al. (2013). Influence of coral and algal exudates on microbially mediated reef metabolism. Peer. J. 1:e108. doi: 10.7717/peerj.108
Hanington, P., Rose, A., and Johnstone, R. (2016). The potential of benthic iron and phosphorus fluxes to support the growth of a bloom forming toxic cyanobacterium Lyngbya majuscula, Moreton Bay, Australia. Mar. Freshwater Res. 67, 1918–1927. doi: 10.1071/MF15219
Harris, J. L. (2015). The Ecology of Turf Algae on Coral Reefs. Ph.D., dissertation, University of California (CA).
Harrison, P. L., Babcock, R. C., Oliver, J. K., Bull, G. D., Wallace, C. C., and Willis, B. L. (1984). Mass spawning in the tropical reef corals. Science 223, 1186–1189. doi: 10.1126/science.223.4641.1186
Hassenrück, C., Fink, A., Lichtschlag, A., Tegetmeyer, H. E., de Beer, D., and Ramette, A. (2016). Quantification of the effects of ocean acidification on sediment microbial communities in the environment: the importance of ecosystem approaches. FEMS Microbiol. Ecol. 92:fiw027. doi: 10.1093/femsec/fiw027
Hensley, N. M., Elmasri, O. L., Slaughter, E. I., Kappus, S., and Fong, P. (2013). Two species of Halimeda, a calcifying genus of tropical macroalgae, are robust to epiphytism by cyanobacteria. Aquat. Ecol. 47, 433–440. doi: 10.1007/s10452-013-9456-x
Heyer, H., and Krumbein, W. E. (1991). Excretion of fermentation products in dark and anaerobically incubated cyanobacteria. Arch. Microbiol. 155, 284–287. doi: 10.1007/BF00252213
Holmes, G., and Johnstone, R. W. (2010). The role of coral mortality in nitrogen dynamics on coral reefs. J. Exp. Mar. Biol. Ecol. 387, 1–8. doi: 10.1016/j.jembe.2010.03.006
Hughes, T. P. (1994). Catastrophes, phase shifts, and large-scale degradation of a Caribbean coral reef. Science 265, 1547–1551.
Hughes, T. P., Rodrigues, M. J., Bellwood, D. R., Ceccarelli, D., Hoegh-Guldberg, O., McCook, L., et al. (2007). Phase shifts, herbivory, and the resilience of coral reefs to climate change. Curr. Biol. 17, 360–365. doi: 10.1016/j.cub.2006.12.049
Jensen, H. S., Mortensen, P. B., Andersen, F., Rasmussen, E., and Jensen, A. (1995). Phosphorus cycling in a coastal marine sediment, Aarhus Bay, Denmark. Limnol. Oceanogr. 40, 908–917.
Jorissen, H., Skinner, C., Osinga, R., de Beer, D., and Nugues, M. M. (2016). Evidence for water-mediated mechanisms in coral-algal interactions. Proc. Biol. Sci. 283, 400–417. doi: 10.1098/rspb.2016.1137
Jouffray, J. B., Nyström, M., Norström, A. V., Williams, I. D., Wedding, L. M., Kittinger, J. N., et al. (2015). Identifying multiple coral reef regimes and their drivers across the Hawaiian archipelago. Philos. T. R. Soc. B. 370:20130268. doi: 10.1098/rstb.2013.0268
Kaebernick, M., and Neilan, B. A. (2001). Ecological and molecular investigations of cyanotoxin production. FEMS Microbiol. Ecol. 35, 1–9. doi: 10.1111/j.1574-6941.2001.tb00782.x
Kelly, L. W., Barott, K. L., Dinsdale, E., Friedlander, A. M., Nosrat, B., Obura, D., et al. (2012). Black reefs: iron-induced phase shifts on coral reefs. ISME J. 6, 638–649. doi: 10.1038/ismej.2011.114
Klein, C. J., Ban, N. C., Halpern, B. S., Beger, M., Game, E. T., Grantham, H. S., et al. (2010). Prioritizing land and sea conservation investments to protect coral reefs. PLoS ONE 5:e12431. doi: 10.1371/journal.pone.0012431
Kline, D. I., Kuntz, N. M., Breitbart, M., Knowlton, N., and Rohwer, F. (2006). Role of elevated organic carbon levels and microbial activity in coral mortality. Mar. Ecol. Prog. Ser. 314, 119–125. doi: 10.3354/meps314119
Klumpp, D. W., and Polunin, N. V. (1989). Partitioning among grazers of food resources within damselfish territories on a coral reef. J. Exp. Mar. Biol. Ecol. 125, 145–169. doi: 10.1016/0022-0981(89)90040-3
Kuffner, I. B., and Paul, V. J. (2001). Effects of nitrate, phosphate and iron on the growth of macroalgae and benthic cyanobacteria from Cocos Lagoon, Guam. Mar. Ecol. Prog. Ser. 222, 63–72. doi: 10.3354/meps222063
Kuffner, I. B., and Paul, V. J. (2004). Effects of the benthic cyanobacterium Lyngbya majuscula on larval recruitment of the reef corals Acropora surculosa and Pocillopora damicornis. Coral Reefs 23, 455–458. doi: 10.1007/s00338-004-0416-8
Kuffner, I. B., Walters, L. J., Becerro, M. A., Paul, V. J., Ritson-Williams, R., and Beach, K. S. (2006). Inhibition of coral recruitment by macroalgae and cyanobacteria. Mar. Ecol. Prog. Ser. 323, 107–117. doi: 10.3354/meps323107
Lapointe, B. E. (1997). Nutrient thresholds for bottom-up control of macroalgal blooms on coral reefs in Jamaica and southeast Florida. Limnol. Oceanogr. 42, 1119–1131.
Lapointe, B. E., Barile, P. J., Littler, M. M., Littler, D. S., Bedford, B. J., and Gasque, C. (2005). Macroalgal blooms on southeast Florida coral reefs I. Nutrient stoichiometry of the invasive green alga Codium isthmocladum in the wider Caribbean indicates nutrient enrichment. Harmful Algae 4, 1092–1105. doi: 10.4319/lo.1997.42.5<uscore>part<uscore>2.1119
Laurent, D., Kerbrat, A. S., Darius, H. T., Girard, E., Golubic, S., Benoit, E., et al. (2008). Are cyanobacteria involved in Ciguatera fish poisoning-like outbreaks in New Caledonia? Harmful Algae 7, 827–838. doi: 10.1016/j.hal.2008.04.005
Laurent, D., Kerbrat, A. S., Darius, H. T., Rossi, F., Yeeting, B., Haddad, M., et al. (2012). “Ciguatera Shellfish Poisoning (CSP): a new ecotoxicological phenomenon from cyanobacteria to humans via giant clams,” in Food Chains: New Research, eds M. A. Jensen and D. W. Muller (New York, NY: Nova Science Publishers), 1–44.
Lee, S., Ferse, S. C. A., Ford, A. K., Wild, C., and Mangubhai, S. (2017). “Effect of sea cucumber density on the health of reef-flat sediments,” in Fiji's Sea Cucumber Fishery: Advances in Science for Improved Management, eds S. Mangubhai, W. Lalanvanua, and S. W. Purcell (Suva, FJ: Wildlife Conservation Society), 54–61.
Levitan, O., Rosenberg, G., Setlik, I., Setlikova, E., Grigel, J., Klepetar, J., et al. (2007). Elevated CO2 enhances nitrogen fixation and growth in the marine cyanobacterium Trichodesmium. Glob. Change Biol. 13, 531–538. doi: 10.1111/j.1365-2486.2006.01314.x
Mactavish, T., Stenton-Dozey, J., Vopel, K., and Savage, C. (2012). Deposit-feeding sea cucumbers enhance mineralization and nutrient cycling in organically-enriched coastal sediments. PloS ONE 7:e50031. doi: 10.1371/journal.pone.0050031
Marnane, M. J., and Bellwood, D. R. (1997). Marker technique for investigating gut throughput rates in coral reef fishes. Mar. Biol. 129, 15–22.
McClanahan, T. R., Donner, S. D., Maynard, J. A., MacNeil, A., Graham, N. A. J., Maina, J., et al. (2012). Prioritizing key resilience indicators to support coral reef management in a changing climate. PLoS ONE 7:e42884. doi: 10.1371/journal.pone.0042884
Méjean, A., Peyraud-Thomas, C., Kerbrat, A. S., Golubic, S., Pauillac, S., Chinain, M., et al. (2010). First identification of the neurotoxin homoanatoxin-a from mats of Hydrocoleum lyngbyaceum (marine cyanobacterium) possibly linked to giant clam poisoning in New Caledonia. Toxicon 56, 829–835. doi: 10.1016/j.toxicon.2009.10.029
Michio, K., Kengo, K., Yasunori, K., Hitoshi, M., Takayuki, Y., Hideaki, Y., et al. (2003). Effects of deposit feeder Stichopus japonicus on algal bloom and organic matter contents of bottom sediments of the enclosed sea. Mar. Pollut. Bull. 47, 118–125. doi: 10.1016/S0025-326X(02)00411-3
Mills, M. M., Ridame, C., Davey, M., La Roche, J., and Geider, R. J. (2004). Iron and phosphorus co-limit nitrogen fixation in the eastern tropical North Atlantic. Nature 429, 292–294. doi: 10.1038/nature02550
Moriarty, D. J. W., Pollard, P. C., Hunt, W. G., Moriarty, C. M., and Wassenberg, T. J. (1985). Productivity of bacteria and microalgae and the effect of grazing by holothurians in sediments on a coral reef flat. Mar. Biol. 85, 293–300. doi: 10.1007/s00338-011-0747-1
Morrow, K. M., Paul, V. J., Liles, M. R., and Chadwick, N. E. (2011). Allelochemicals produced by Caribbean macroalgae and cyanobacteria have species-specific effects on reef coral microorganisms. Coral Reefs 30, 309–320. doi: 10.1007/BF00393250
Mumby, P. J., Dahlgren, C. P., Harborne, A. R., Kappel, C. V., Micheli, F., Brumbaugh, D. R., et al. (2006). Fishing, trophic cascades, and the process of grazing on coral reefs. Science 311, 98–101. doi: 10.1126/science.1121129
Mur, L. R., Skulberg, O. M., and Utkilen, H. (1999). “Cyanobacteria in the environment,” in Toxic Cyanobacteria in Water: A Guide to Their Public Health Consequences, Monitoring and Management, eds I. Chorus and J. Bartram (London: E and FN Spon), 25–52.
Nagle, D. G., and Paul, V. J. (1998). Chemical defense of a marine cyanobacterial bloom. J. Exp. Mar. Biol. Ecol. 225, 29–38. doi: 10.1016/S0022-0981(97)00205-0
Norström, A. V., Nyström, M., Lokrantz, J., and Folke, C. (2009). Alternative states on coral reefs: beyond coral-macroalgal phase shifts. Mar. Ecol. Prog. Ser. 376, 295–306. doi: 10.3354/meps07815
O'Neil, J. M., Davis, T. W., Burford, M. A., and Gobler, C. J. (2012). The rise of harmful cyanobacteria blooms: the potential roles of eutrophication and climate change. Harmful Algae 14, 313–334. doi: 10.1016/j.hal.2011.10.027
Obura, D. O. (2009). Coral Reef Resilience Assessment of the Nosy Hara Marine Protected Area, Northwest Madagascar. Gland: IUCN.
Osborne, N. J. T., Webb, P. M., and Shaw, G. R. (2001). The toxins of Lyngbya majuscula and their human and ecological health effects. Environ. Int. 27, 381–392. doi: 10.1016/S0160-4120(01)00098-8
Paerl, H. (2008). “Nutrient and other environmental controls of harmful cyanobacterial blooms along the freshwater – marine continuum,” in Cyanobacterial Harmful Algal Blooms, State of the Science and Research Needs, ed H. K. Hudnell (New York, NY: Springer), 217–237.
Paerl, H. W., and Paul, V. J. (2012). Climate change: links to global expansion of harmful cyanobacteria. Water Res. 46, 1349–1363. doi: 10.1016/j.watres.2011.08.002
Paerl, H. W., Pinckney, J. L., and Steppe, T. F. (2000). Cyanobacterial-bacterial mat consortia: examining the functional unit of microbial survival and growth in extreme environments. Environ. Microbiol. 2, 11–26.
Paul, V. J. (2008). “Global warming and cyanobacterial harmful algal blooms,” in Cyanobacterial Harmful Algal Blooms: State of the Science and Research Needs, ed H. K. Hudnell (New York, NY: Springer), 239–257.
Paul, V. J., Arthur, K. E., Ritson-Williams, R., Ross, C., and Sharp, K. (2007). Chemical defenses: from compounds to communities. Biol. Bull. 213, 226–251. doi: 10.2307/25066642
Paul, V. J., Nelson, S., and Sanger, H. (1990). Feeding preferences of adult and juvenile rabbitfish Siganus argenteus in relation to chemical defenses of tropical seaweeds. Mar. Ecol. Prog. Ser. 60, 23–34.
Paul, V. J., Thacker, R. W., Banks, K., and Golubic, S. (2005). Benthic cyanobacterial bloom impacts the reefs of South Florida (Broward County, USA). Coral Reefs 24, 693–697. doi: 10.1007/s00338-005-0061-x
Pennings, S. C., Pablo, S. R., and Paul, V. J. (1997). Chemical defenses of the tropical, benthic marine cyanobacterium Hormothamnion enteromorphoides: diverse consumers and synergisms. Limnol. Oceanogr. 42, 911–917. doi: 10.4319/lo.1997.42.5.0911
Plotieau, T., Baele, J., Vaucher, R., Hasler, C., Koudad, D., and Eeckhaut, I. (2013). Analysis of the impact of Holothuria scabra intensive farming on sediment. Cah. Biol. Mar. 54, 703–711.
Pogoreutz, C., Rädecker, N., Cárdenas, A., Gärdes, A., Voolstra, C. R., and Wild, C. (2017). Sugar enrichment provides evidence for a role of nitrogen fixation in coral bleaching. Glob. Change Biol. 23, 3838–3848. doi: 10.1111/gcb.13695
Pratchett, M. S., Hoey, A. S., and Wilson, S. K. (2014). Reef degradation and the loss of critical ecosystem goods and services provided by coral reef fishes. Curr. Opin. Env. Sust. 7, 37–43. doi: 10.1016/j.cosust.2013.11.022
Puyana, M., Acosta, A., Bernal-Sotelo, K., Velásquez-Rodríguez, T., and Ramos, F. (2015). Spatial scale of cyanobacterial blooms in old providence Island, Colombian Caribbean. Univ. Sci. 20, 83–105. doi: 10.11144/Javeriana.SC20-1.sscb
Puyana, M., and Prato, J. (2013). Overgrowth of reef organisms by benthic cyanobacteria in the Colombian Caribbean. Mutis 3, 58–60. doi: 10.21789/22561498.885
Quinn, N. J., and Kojis, B. L. (2008). “Variation in coral recruitment on Fijian reefs,” in Proceedings of the 11th International Coral Reef Symposium 2012 (Fort Lauderdale), 459–463.
Rädecker, N., Pogoreutz, C., Voolstra, C. R., Wiedenmann, J., and Wild, C. (2015). Nitrogen cycling in corals: the key to understanding holobiont functioning? Trends Microbiol. 23, 490–497. doi: 10.1016/j.tim.2015.03.008
Richmond, R. H., Rongo, T., Golbuu, Y., Victor, S., Idechong, N., Davis, G., et al. (2007). Watersheds and coral reefs: conservation science, policy, and implementation. BioScience 57, 598–607. doi: 10.1641/B570710
Rippka, R. (1972). Photoheterotrophy and chemoheterotrophy among unicellular blue-green algae. Arch. Mikroiol. 87, 93–98.
Ritson-Williams, R., Paul, V. J., and Bonito, V. (2005). Marine benthic cyanobacteria overgrow coral reef organisms. Coral Reefs 24, 629. doi: 10.1007/s00338-005-0059-4
Ritson-Williams, R., Ross, C., Paul, V. J., Fabricius, K., Negri, A., and Hoegh-Guldberg, O. (2016). Elevated temperature and allelopathy impact coral recruitment. PLoS ONE 11:e0166581. doi: 10.1371/journal.pone.0166581
Roff, G., and Mumby, P. J. (2012). Global disparity in the resilience of coral reefs. Trends Ecol. Evol. 27, 404–413. doi: 10.1016/j.tree.2012.04.007
Rose, A. L., and Waite, T. D. (2005). Reduction of organically complexed ferric iron by superoxide in a simulated natural water. Environ. Sci. Technol. 39, 2645–2650. doi: 10.1021/es048765k
Rosset, S., Wiedenmann, J., Reed, A. J., and D'Angelo, C. (2017). Phosphate deficiency promotes coral bleaching and is reflected by the ultrastructure of symbiotic dinoflagellates. Mar. Pollut. Bull. 118, 180–187. doi: 10.1016/j.marpolbul.2017.02.044
Sandrini, G., Ji, X., Verspagen, J. M. H., Tann, R. P., Slot, P. C., Luimstra, V. M., et al. (2016). Rapid adaptation of harmful cyanobacteria to rising CO2. Proc. Natl. Acad. Sci. U.S.A. 113, 9315–9320. doi: 10.1073/pnas.1602435113
Schils, T. (2012). Episodic eruptions of volcanic ash trigger a reversible cascade of nuisance species outbreaks in pristine coral habitats. PLoS ONE 7:e46639. doi: 10.1371/journal.pone.0046639
Schroeder, R. E., Green, A. L., DeMartini, E. E., and Kenyon, J. C. (2008). Long-term effects of a ship-grounding on coral reef fish assemblages at Rose Atoll, American Samoa. B. Mar. Sci. 82, 345–364.
Sheppard, C., Dixon, D. J., Gourlay, M., Sheppard, A., and Payet, R. (2005). Coral mortality increases wave energy reaching shores protected by reef flats: examples from the Seychelles. Estuar. Coast. Shelf. S. 64, 223–234. doi: 10.1016/j.ecss.2005.02.016
Skinner, M. P., Brewer, T. D., Johnstone, R., Fleming, L. E., and Lewis, R. J. (2011). Ciguatera fish poisoning in the Pacific Islands (1998 to 2008). PLoS Neglect. Trop. D. 5:e1416. doi: 10.1371/journal.pntd.0001416
Smith, J. E., Brainard, R., Carter, A., Grillo, S., Edwards, C., Harris, J., et al. (2016). Re-evaluating the health of coral reef communities: baselines and evidence for human impacts across the central Pacific. Proc. R. Soc. B. Biol. Sci. 283:20151985. doi: 10.1098/rspb.2015.1985
Smith, J. E., Shaw, M., Edwards, R. A., Obura, D., Pantos, O., Sala, E., et al. (2006). Indirect effects of algae on coral: algae-mediated, microbe-induced coral mortality. Ecol. Lett. 9, 835–845. doi: 10.1111/j.1461-0248.2006.00937.x
Sournia, A. (1976). Ecologie et productivité d'une Cyanophycée en milieu corallien: Oscillatoria limosa Agardh. Phycologia 15, 363–366.
Staal, M., Meysman, F. J. R., and Stal, L. J. (2003). Temperature excludes N2-fixing heterocystous cyanobacteria in the tropical oceans. Nature 425, 504–507. doi: 10.1038/nature01999
Stal, L. J. (2012). “Cyanobacterial mats and stromatolites,” in The Ecology of Cyanobacteria II. Their Diversity in Space and Time, ed B. Whitton (Dordrecht, NL: Springer), 65–126.
Sundby, B., Gobeil, C., Silverberg, N., and Alfonso, M. (1992). The phosphorus cycle in coastal marine sediments. Limnol. Oceanogr. 37, 1129–1145.
Thacker, R., Ginsburg, D., and Paul, V. (2001). Effects of herbivore exclusion and nutrient enrichment on coral reef macroalgae and cyanobacteria. Coral Reefs 19, 318–329. doi: 10.1007/s003380000122
Thacker, R., Nagle, D., and Paul, V. (1997). Effects of repeated exposures to marine cyanobacterial secondary metabolites on feeding by juvenile rabbitfish and parrotfish. Mar. Ecol. Prog. Ser. 147, 21–29.
Thacker, R. W., and Paul, V. J. (2001). Are benthic cyanobacteria indicators of nutrient enrichment? Relationships between cyanobacterial abundance and environmental factors on the reef flats of Guam. B. Mar. Sci. 69, 497–508.
Titlyanov, E. A., Yakovleva, I. M., and Titlyanova, T. V. (2007). Interaction between benthic algae Lyngbya bouillonii, Dictyota dichotoma and scleractinian coral Porites lutea in direct contact. J. Exp. Mar. Biol. Ecol. 342, 282–291. doi: 10.1016/j.jembe.2006.11.007
Ullah, H., Nagelkerken, I., Goldenberg, S. U., and Fordham, D. A. (2018). Climate change could drive marine food web collapse through altered trophic flows and cyanobacterial proliferation. PLoS Biol. 16:e2003446. doi: 10.1371/journal.pbio.2003446
Uthicke, S. (1994). Ecology of two sediment feeding holothurians, Holothuria (Halodeima) atra (Jaeger 1883) and Stichopus chloronotus (Brand, 1835), on reefs near Lizard Island, Australia. SPC Bêche-de-Mer Info. Bull. 6, 19–20.
Uthicke, S. (1999). Sediment bioturbation and impact of feeding activity of Holothuria (Halodeima) atra and Stichopus chloronotus, two sediment feeding holothurians, at Lizard Island, Great Barrier Reef. B. Mar. Sci. 64, 129–141.
Van Alstyne, K. L., and Pelletreau, K. N. (2000). Effects of nutrient enrichment on growth and phlorotannin production in Fucus gardneri embryos. Mar. Ecol. Prog. Ser. 206, 33–43. doi: 10.3354/meps206033
Van Veghel, M. L. J. (1994). Reproductive characteristics of the polymorphic Caribbean reef building coral Montastrea annularis. Gametogenesis and spawning behavior. Mar. Ecol. Prog. Ser. 109, 209–219.
Visser, P. M., Verspagen, J. M. H., Sandrini, G., Stal, L. J., Matthijs, H. C. P., Paerl, H. W., et al. (2016). How rising CO2 and global warming may stimulate harmful cyanobacterial blooms. Harmful Algae 54, 145–159. doi: 10.1016/j.hal.2015.12.006
Wanders, J. B. W. (1977). The role of benthic algae in the shallow reef of Curaçao (Netherlands Antilles) III: the significance of grazing. Aquat. Bot. 3, 357–390. doi: 10.1016/0304-3770(77)90040-7
Watkinson, A. J., O'Neil, J. M., and Dennison, W. C. (2005). Ecophysiology of the marine cyanobacterium, Lyngbya majuscula (Oscillatoriaceae) in Moreton Bay, Australia. Harmful Algae 4, 697–715. doi: 10.1016/j.hal.2004.09.001
Wiedenmann, J., D'Angelo, C., Smith, E., Hunt, A., Legiret, F.-E., Postle, A., et al. (2013). Nutrient enrichment can increase the susceptibility of reef corals to bleaching. Nat. Clim. Change 3, 160–164. doi: 10.1038/nclimate1661
Yamashiro, H., Isomura, N., and Sakai, K. (2014). Bloom of the cyanobacterium Moorea bouillonii on the gorgonian coral Annella reticulata in Japan. Sci. Rep. 4:6032. doi: 10.1038/srep06032
Zaneveld, J. R., Burkepile, D. E., Shantz, A. A., Pritchard, C. E., McMinds, R., Payet, J. P., et al. (2016). Overfishing and nutrient pollution interact with temperature to disrupt coral reefs down to microbial scales. Nat. Commun. 7:11833. doi: 10.1038/ncomms11833
Keywords: coral reefs, ecosystem degradation, alternative states, ecological feedbacks, global environmental change, social-ecological traps, local management
Citation: Ford AK, Bejarano S, Nugues MM, Visser PM, Albert S and Ferse SCA (2018) Reefs under Siege—the Rise, Putative Drivers, and Consequences of Benthic Cyanobacterial Mats. Front. Mar. Sci. 5:18. doi: 10.3389/fmars.2018.00018
Received: 16 October 2017; Accepted: 16 January 2018;
Published: 02 February 2018.
Edited by:
Hajime Kayanne, The University of Tokyo, JapanReviewed by:
Deron Burkepile, University of California, Santa Barbara, United StatesUlisse Cardini, Stazione Zoologica Anton Dohrn, Italy
Copyright © 2018 Ford, Bejarano, Nugues, Visser, Albert and Ferse. This is an open-access article distributed under the terms of the Creative Commons Attribution License (CC BY). The use, distribution or reproduction in other forums is permitted, provided the original author(s) and the copyright owner are credited and that the original publication in this journal is cited, in accordance with accepted academic practice. No use, distribution or reproduction is permitted which does not comply with these terms.
*Correspondence: Amanda K. Ford, amanda.ford@leibniz-zmt.de