- 1Alfred Wegener Institute, Helmholtz Centre for Polar and Marine Research, Potsdam, Germany
- 2GFZ German Research Centre for Geosciences, Helmholtz Centre Potsdam, Section 5.3 Geomicrobiology, Potsdam, Germany
- 3GFZ German Research Centre for Geosciences, Helmholtz Centre Potsdam, Section 3.2 Organic Geochemistry, Potsdam, Germany
Microorganisms in Antarctic glacier forefields are directly exposed to the hostile environment of their habitat characterized by extremely low temperatures and changing geochemical conditions. To survive under those stress conditions microorganisms adapt, among others, their cell membrane fatty acid inventory. However, only little is known about the adaptation potential of microorganisms from Antarctic soil environments. In this study, we examined the adaptation of the cell membrane polar lipid fatty acid inventory of Chryseobacterium frigidisoli PB4T in response to changing temperature (0°C to 20°C) and pH (5.5 to 8.5) regimes, because this new strain isolated from an Antarctic glacier forefield showed specific adaptation mechanisms during its detailed physiological characterization. Flavobacteriaceae including Chryseobacterium species occur frequently in extreme habitats such as ice-free oases in Antarctica. C. frigidisoli shows a complex restructuring of membrane derived fatty acids in response to different stress levels. Thus, from 20°C to 10°C a change from less iso-C15:0 to more iso-C17:1ω7 is observed. Below 10°C temperature adaptation is regulated by a constant increase of anteiso-FAs and decrease of iso-FAs. An anteiso- and bis-unsaturated fatty acid, anteiso-heptadeca-9,13-dienoic acid, shows a continuous increase with decreasing cultivation temperatures underlining the particular importance of this fatty acid for temperature adaptation in C. frigidisoli. Concerning adaptation to changing pH conditions, most of the dominant fatty acids reveal constant relative proportions around neutral pH (pH 6–8). Strong variations are mainly observed at the pH extremes (pH 5.5 and 8.5). At high pH short chain saturated iso- and anteiso-FAs increase while longer chain unsaturated iso- and anteiso-FAs decrease. At low pH the opposite trend is observed. The study shows a complex interplay of different membrane components and provides, therefore, deep insights into adaptation strategies of microorganisms from extreme habitats to changing environmental conditions.
Introduction
Microorganisms successfully colonize almost all existing ecological niches, including hostile environments such as hot springs, the deep sea, hot or polar deserts (Rothschild and Mancinelli, 2001). The polar regions are microbial-dominated ecosystems and create perfect conditions for extremophiles (Wynn-Williams, 1996). The Antarctic continent is characterized by extreme climatic conditions, limited nutrient availability, high salinity, low temperatures and low water activity (Cannone et al., 2008). Soil microbial communities are able to respond and adapt to severe environmental conditions such as changing temperature and pH regimes (Ganzert et al., 2011; Bakermans et al., 2012; Bajerski and Wagner, 2013). In general, there are several ways of stress response such as the induction of special heat/cold shock proteins, the production and release of protective compatible solutes or an enhanced/reduced metabolism (Georlette et al., 2004). Another crucial adaptation process is the ability to adjust the cell membrane structure, because a fluid cell membrane is essential for microorganisms to maintain the function of important metabolic systems such as the electron transport chain (Denich et al., 2003). Bacterial cell membranes are mainly formed by polar lipid (PL) bilayers (e.g., phospholipids) best described in the “Mosaic Model” by Singer (Singer, 1972). Microorganisms have developed several mechanisms to change their cell membrane composition in order to maintain cell membrane fluidity and functionality in response to shifting environmental conditions, which is known as homeoviscous adaption (Sinensky, 1974). The membrane PL composition can change regarding the polar head groups or the acyl side chains (Russell, 1984; Boggs, 1986). Microbial PL fatty acid side chains are saturated or monounsaturated (rarely polyunsaturated) fatty acids with 12 to 24 carbon atoms and the acyl side chains can contain branches or ring structures such as cyclopropyl, -pentyl, and –hexyl rings. Thus, the phenotypic adaption of the cell membrane structure can be regulated by the degree of unsaturation, the chain length, branching or cyclisation of bacterial membrane fatty acids (Denich et al., 2003). The adaption to low temperature is realized by a higher proportion of unsaturated fatty acids and a shift to more short chain fatty acids (Suutari and Laakso, 1994). Another adaptation process is the change of iso- and anteiso-branched fatty acids, whereas the proportion of anteiso-fatty acids increases with decreasing temperature (Kaneda, 1991). The incorporation of cis-unsaturation, shorter-chained fatty acids and fatty acids with branches or cycles reduces the melting temperature of the cell membrane leading to an increased fluidity at low temperature (Russell, 1989; Mangelsdorf et al., 2009).
Several studies indicate different and variable adaptations of the PL inventory in response to changing pH regimes: A decrease of iso-C15:0 and iso-C16:0 at increasing pH and no significant effect of the anteiso-branched fatty acids was reported (Bååth and Anderson, 2003) as well as an increase of anteiso-C15:0 and no change of iso-C15:0 at higher pH (Männistö et al., 2007). Furthermore, the proportion of unsaturated fatty acid may increase with rising pH (Männistö et al., 2007). The incorporation of a cyclopropane ring may enhance the stability of the cell membrane at low pH, low temperature or in slowly growing cells (Russell, 1989; Brown et al., 1997). Since these results show no clear picture additional knowledge on the pH adaption of the microbial cell membrane is needed.
Unsaturation can be induced post-synthesis by desaturase as a rapid answer system. Other adaptions like chain length or branching need de novo synthesis and thereby require bacterial growth under extreme conditions (Russell, 1984). Former studies provide a general idea of the mechanisms involved in bacterial cell membrane adaptation to changing environmental condition (reviewed in Denich et al., 2003), but the structural adaptation of microorganism from extreme habitats such as Antarctic soils still remain poorly understood. Furthermore, microorganisms are very divers in their biochemistry and physiology and the adaptation of the fatty acid composition in response to stress varies between different bacteria (Russell, 1984; Denich et al., 2003). Studying the effects of different environmental parameters on the cell membrane structure could give new insights in the adaptation mechanisms of the microorganism to the extreme environment, because microorganisms can apply several mechanisms to maintain the fluidity and functionality of the cell membrane under different conditions.
In this study, we focus on the temperature and pH adaption of the cell membrane composition of Chryseobacterium frigidisoli PB4T, a cold-adapted representative of the Flavobacteriaceae family in the phylum Bacteroidetes, which was isolated from a mineral soil of a glacier forefield of the Larsemann Hills, East Antarctica (Bajerski et al., 2013). Flavobacteriaceae are widely distributed in Antarctic habitats (Abell and Bowman, 2005; Cary et al., 2010) and representatives of the genus Chryseobacterium occur frequently in Antarctic soils and sediments (Teixeira et al., 2010; Zdanowski et al., 2013; Gugliandolo et al., 2016). In an ecological study of the earlier mentioned glacier forefield Chryseobacterium were detected in the culture-independent clone library sequences and several Chryseobacterium strains have been isolated, including C. frigidisoli PB4T (Bajerski et al., 2013). This new isolate from an ice-free Antarctic oasis showed special adaptation mechanisms during the detailed physiological description, among others a novel fatty acid was discovered and identified as anteiso-heptadeca-9,13-dienoic acid, a ω3,7-fatty acid (Mangelsdorf et al., 2017). We investigated its peculiarities for adaptation to low temperatures and pH values, which are relevant properties in Antarctic soils. More precisely, changes in the membrane fatty acid composition of C. frigidisoli were analyzed after incubations of the cells between 0°C and 20°C and in a pH range of 5.5 to 8.5.
Materials and Methods
Study Site
The Larsemann Hills are an ice-free oasis situated in the Prydz Bay region in East Antarctica (69°30′S, 76°20′E; Stüwe et al., 1989). The study site is characterized by severe climatic conditions with low temperatures between -29°C and 0°C and little precipitation of about 250 mm a-1 (Hodgson et al., 2001). Soil formation processes could not be observed and the studied material can be classified as dry and oligotrophic weathering debris. A detailed site description is given in an environmental study dealing with bacterial succession in two glacier forefields of the Larsemann Hills (Bajerski and Wagner, 2013). The studied bacterial strain was isolated from the weathering debris of the glacier forefield called “Black Valley Transect” in 446 m distance from the glacier (S 69°24.315; E 76°20.295; Bajerski et al., 2013). The harvested profile was characterized by a black organic mat mixed with coarse-grained sandy material at the surface and sandy permanently frozen ground in 1–6 cm depth. The soil pH of the study site ranged from acidic (pH 4.9) to alkaline (8.3), but the studied strain originated from a sampling site with almost neutral pH (6.8).
Bacterial Strains
Chryseobacterium frigidisoli strain PB4T was chosen to examine the effect of changing temperature and pH on the fatty acid composition of the bacterial cell membrane. This strain was described as a novel psychrotolerant bacterium (Bajerski et al., 2013) being able to grow between 0 and 25°C with an optimum growth at 20°C (Figure 1A). The gram-negative bacterium grows at pH values from pH 5.5–8.5 with optimum at pH 6.5 (Figure 1B). The turbidity of the cell culture was measured photometrical at 600 nm to determine the optical density (OD) during an incubation in R2A media (0.05% Proteose peptone, 0.05% Casamino acids, 0.05% yeast extract, 0.05% dextrose, 0.05% soluble starch, 0.03% dipotassium phosphate, 0.005% magnesium sulfate 7× H2O, 0.03% sodium pyruvate (w/v); pH 7.2; Reasoner and Geldreich, 1985). The specific growth rate μ was determined in the exponential growth phase (linear range) using the measured ODs and was calculated as follows:
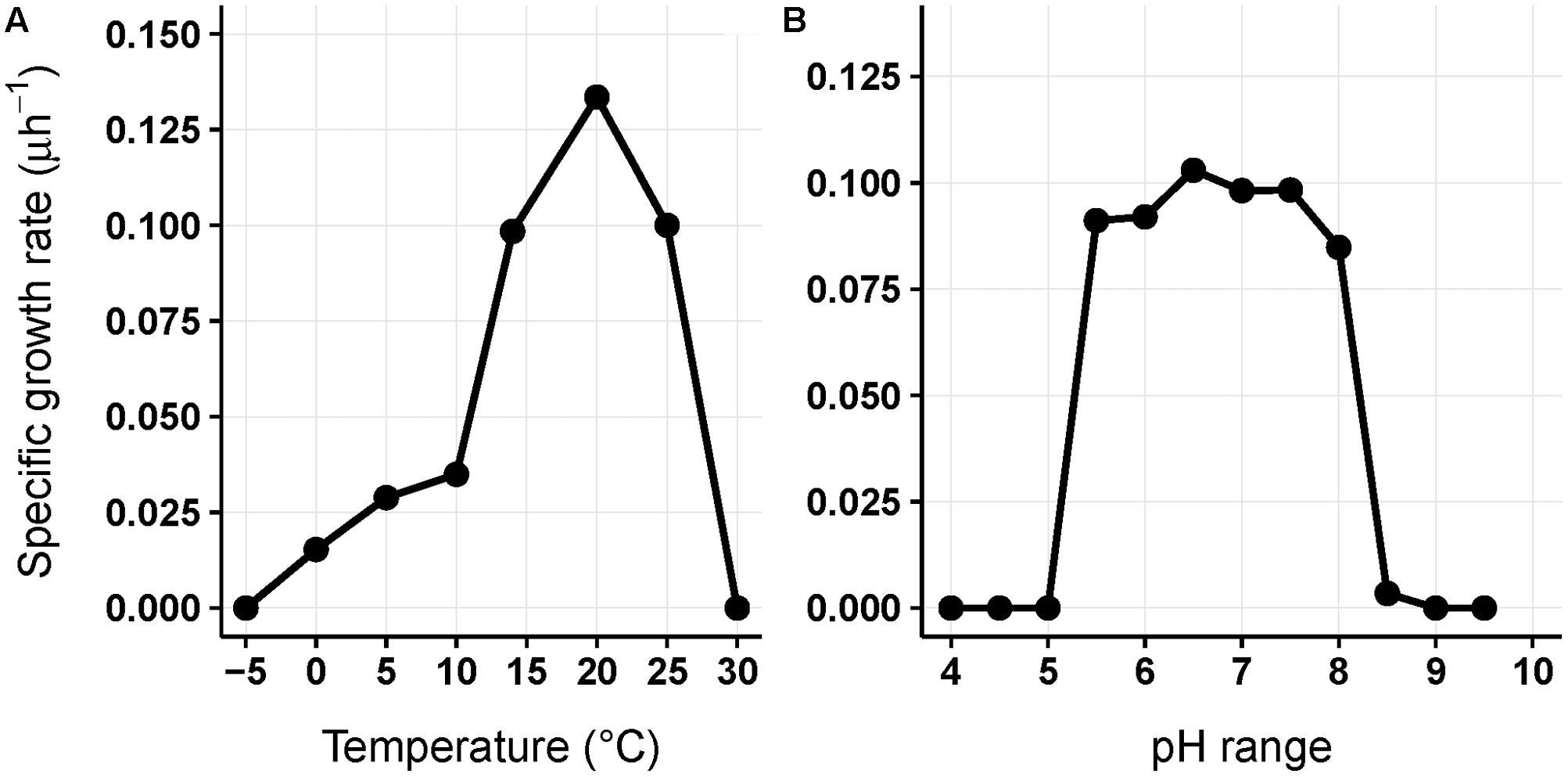
FIGURE 1. Physiological characteristics of strain Chryseobacterium frigidisoli PB4T; specific growth rate in dependence of the temperature (A) and pH (B), respectively. The specific growth rate μ was determined by measuring turbidity photometrically at 600 nm during incubation in R2A media.
To study temperature adaption the strain was incubated at 0, 5, 10, 14, 20°C in R2A medium (pH 7.2). The impact of changing pH values on the membrane composition was analyzed using the R2A medium (Reasoner and Geldreich, 1985) modified with the following buffers: glycine (pH 4.0–5.0 and pH 10.0), MES [2-(N-morpholino)ethanesulfonic acid; pH 5.0–6.5], HEPES [4-(2-hydroxyethyl)-1-piperazineethanesulfonic acid; pH 7.0-8.0], and BTP ([1,3-bis(tris(hydroxymethyl)methylamino)propane]; pH 8.5–9.5; Hoaki et al., 1994). Growth in dependence of pH was detected at pH 5.5, 6.0, 6.5, 7.0, 7.5, 8.0, and 8.5 after incubation at 18°C (in the range of the temperature optimum). For PL extraction cells from biological triplicates were harvested at the transition from late exponential to early stationary growth phase by centrifuging the combined cultures for 5 min at 10.000 g, washing with sterilized tab water and centrifuging again.
Analytical Procedures
The cell pellets of C. frigidisoli cultivated under different temperature and pH conditions were extracted with a flow blending system using a solvent mixture of methanol (MeOH)/dichloromethane (DCM)/ammonium acetate buffer with a ratio of 2:1:0.8 (v/v) for 5 min (modified after Bligh and Dyer, 1959). Afterwards, the solvent ratio of the extract was changed to 1:1:0.9 by adding DCM and ammonium acetate buffer resulting into a phase separation of an organic and an aqueous phase. Subsequently, the aqueous phase was re-extracted three times with DCM. The combined organic phases were concentrated using a Turbo Vap system (Zymark). Afterwards, the extract was chromatographically separated into fractions of different polarity resulting, among other fractions, into a polar lipid (PL) fraction as described in Zink and Mangelsdorf (2004). This standard protocol was used mainly to clean up the PL fraction and to remove free fatty acids from the extract, thus they cannot mix up with the side chain fatty acids from the polar membrane lipids. Here we focused on the PL fraction since it contains the main cell membrane lipids of C. frigidisoli. An aliquot of the PL fraction was used for trans-esterification to obtain the methylated membrane fatty acids by following the method described by Müller et al. (1990). The aliquot of the PL fraction was dissolved in 50 μl of DCM/MeOH (9:1 v/v) in a 2 ml vial. Afterward 50 μl of trimethylsulfoniumhydroxid (TMSH) was added and the vial was sealed before placing in an oven for 2 h at 70°C. The trans-esterified (methylated) fatty acids were dried and subsequently dissolved in 50 μl DCM before analysis on a gas chromatographic system (Trace GC Ultra, Thermo Electron) coupled to a DSQ Thermo Electron Quadrupole mass spectrometer. The GC was equipped with a cold injection system operating in the splitless mode and a SGE BPX 5 fused silica capillary column (50 m length, 0.22 mm ID, 0.25 μm film thickness) using the following temperature program: initial temperature 50°C, 1 min isotherm, heating rate 3°C/min to 310°C, held isothermal for 30 min. Helium was used as a carrier gas with a constant flow rate of 1 mL min-1. The injector temperature was programmed from 50 to 300°C at a rate of 10°C s-1. Full scan mass spectra were recorded from m/z 50 to 600 at a scan rate of 2.5 scans s-1.
Statistics
Correlation and significance of the derived data was calculated with IBM SPSS Statistics 21 analyzing bivariate correlation using the Pearson correlation coefficient and testing two-tailed significance.
Results and Discussion
Fatty Acid Inventory of C. frigidisoli PB4T
The detected polar membrane lipids of C. frigidisoli are phosphatidylethanolamines, ornithine lipids as well as flavolipins and flavocristamides. The major fatty acids (>10%, Figures 2, 3 and Tables 1, 2) determined in this study obtained after saponification of membrane lipids are iso- and anteiso-pentadecanoic acid (iso-, anteiso-C15:0), iso-2-hydroxypentadecanoic acid (iso-2OH-C15:0), iso-heptadeca-9-enoic acid (iso-C17:1ω7) and a novel fatty acid, which, to our knowledge, has not been previously described. The structure of the newly discovered fatty acid was identified as anteiso-heptadeca-9,13-dienoic acid, a ω3,7-fatty acid with a branching position directly at the ω3 double bond. The experiments, which were performed to identify the detailed structure of the newly discovered fatty acid, are described elsewhere (Mangelsdorf et al., 2017). The identified major fatty acids are in accordance with the fatty acid composition of the strain description that identified iso-2OH-C15:0 and iso-C15:0 as the major fatty acids (Bajerski et al., 2013). Minor variations between this study and the presented results can be explained by different harvesting time points. In the strain description of Bajerski et al. (2013) cells were harvested at the end of the exponential growth phase, whereas in this study cell material was collected at the transition from late exponential to early stationary growth phase. The predominance of iso-2OH-C15:0 and iso-C15:0 at optimum growth seem to be shifted to other C15 and longer unsaturated C17 fatty acids under sub-optimal conditions (stress/stationary phase). While iso-C15:0 is predominant in many Chryseobacterium species (Vandamme et al., 1994), the high amount of iso-2OH-C15:0 appears to be distinctive in C. frigidisoli. It should be noted that the iso-C17:1ω7 fatty acid was wrongly labeled as iso-C17:1ω8 in Bajerski et al. (2013). Other fatty acids such as iso-tridecanoic acid (iso-C13:0), iso-tetradecanoic acid (iso-C14:0), pentadecanoic acid (C15:0), iso-hexadecenoic acid (iso-C16:1, presumably iso-C16:1ω6), iso-hexadecanoic acid (iso-C16:0), iso-2-methoxy-pentadecanoic acid (iso-2methoxy-C15:0), anteiso-2-hydroxy-pentadecanoic acid (anteiso-2OH-C15:0), hexadecanoic acid (C16:0), anteiso-heptadec-9-enoic acid (anteiso-C17:1ω7), octadec-9-enoic acid (C18:1ω9) and octadecanoic acid (C18:0) occur only in minor to trace amounts accounting in total for less than 10 % of the total cell membrane derived fatty acids pattern (Tables 1, 2). In some of the minor unsaturated components the exact double bond position could not be assigned to a standard or identified by derivatization experiments (Mangelsdorf et al., 2017), thus, they are simply labeled as unsaturated fatty acids (Figures 3, 4 and Tables 1, 2, respectively).
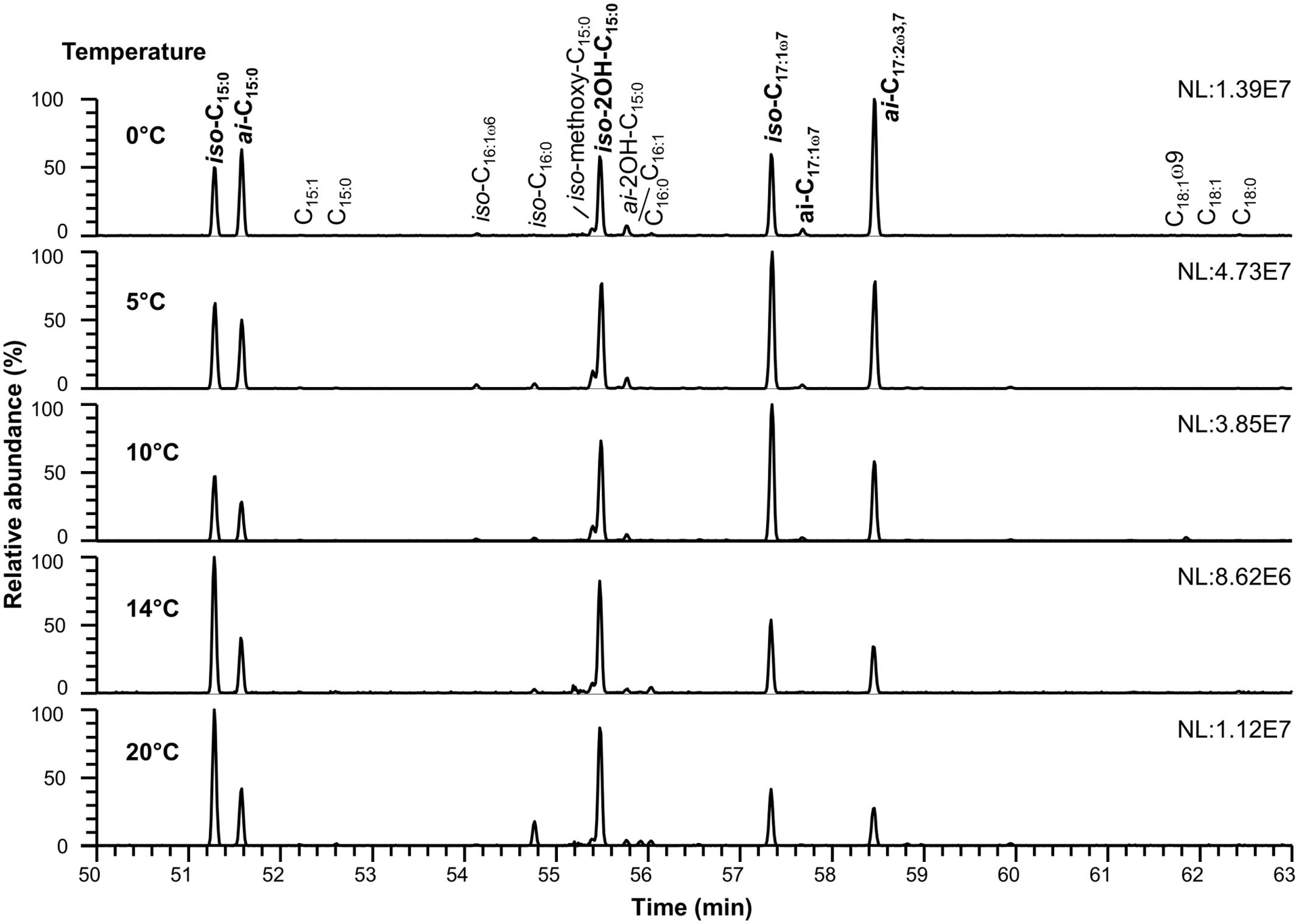
FIGURE 2. Cell membrane derived fatty acid inventory of C. frigidisoli PB4T at different temperatures. To study temperature adaption the strain was incubated at 0, 5, 10, 14, 20°C in R2A medium (pH 7.2). The major fatty acids (>10%) are shown in bold. For a detailed list of all membrane fatty acids see Table 1.
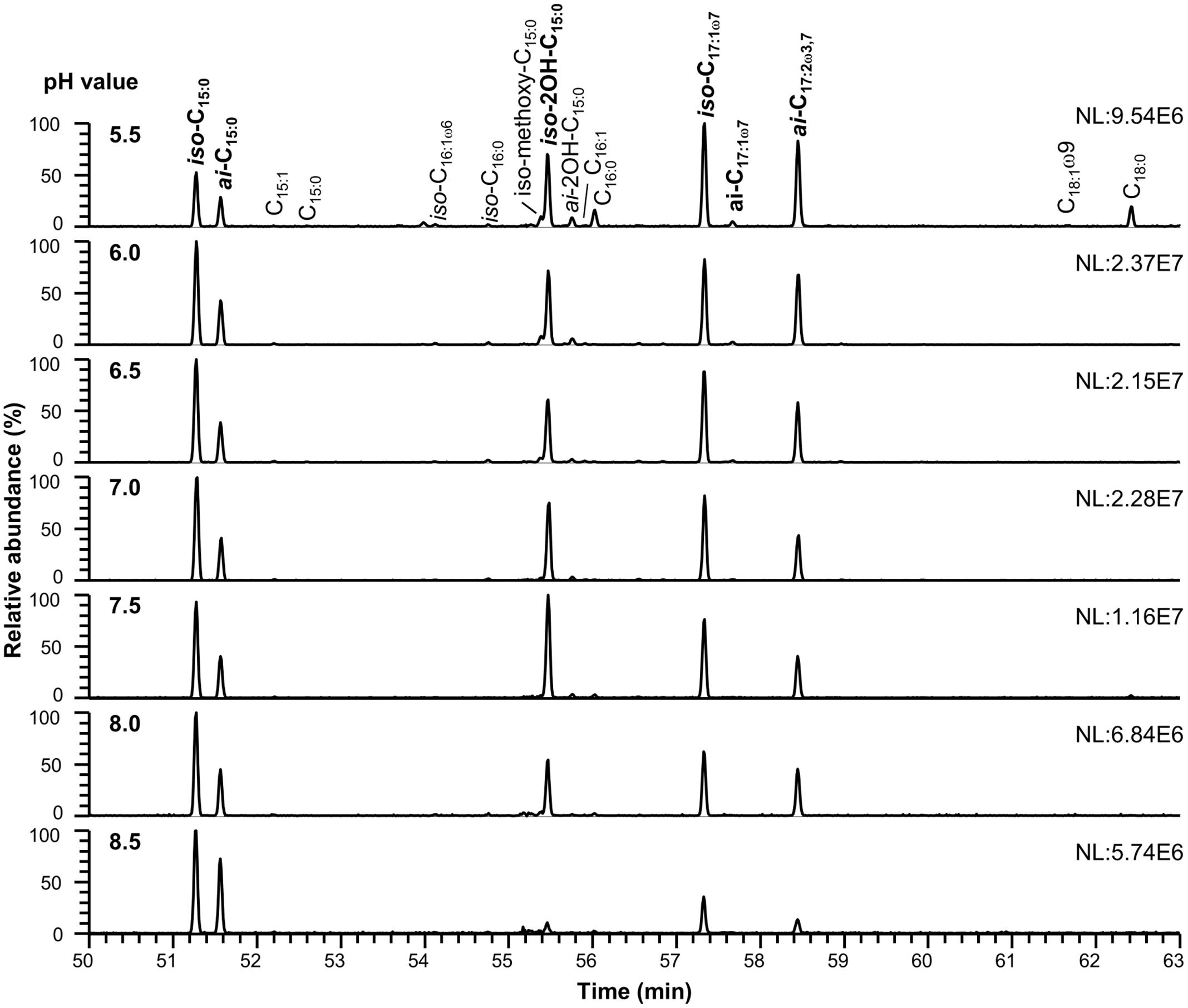
FIGURE 3. Cell membrane derived fatty acid of C. frigidisoli PB4T at different pH conditions. Growth in dependence of pH was detected at pH 5.5, 6.0, 6.5, 7.0, 7.5, 8.0, and 8.5 after incubation in R2A medium at 18°C (in the range of the temperature optimum). The major fatty acids (>10%) are shown in bold. For a detailed list of all membrane fatty acids see Table 2.
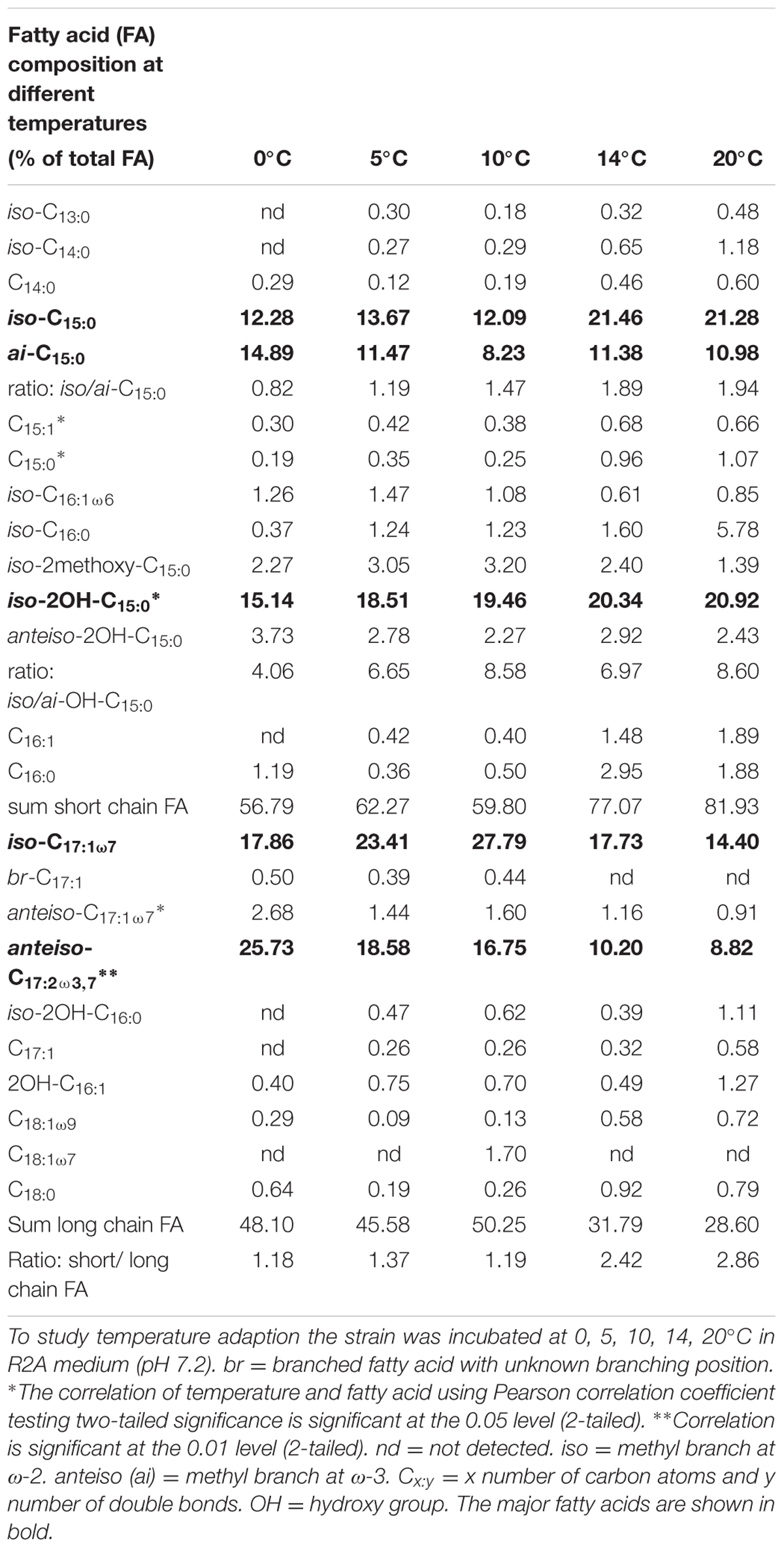
TABLE 1. Adaption of the cell membrane derived fatty acid inventory of Chryseobacterium frigidisoli PB4T with respect to different temperature conditions.
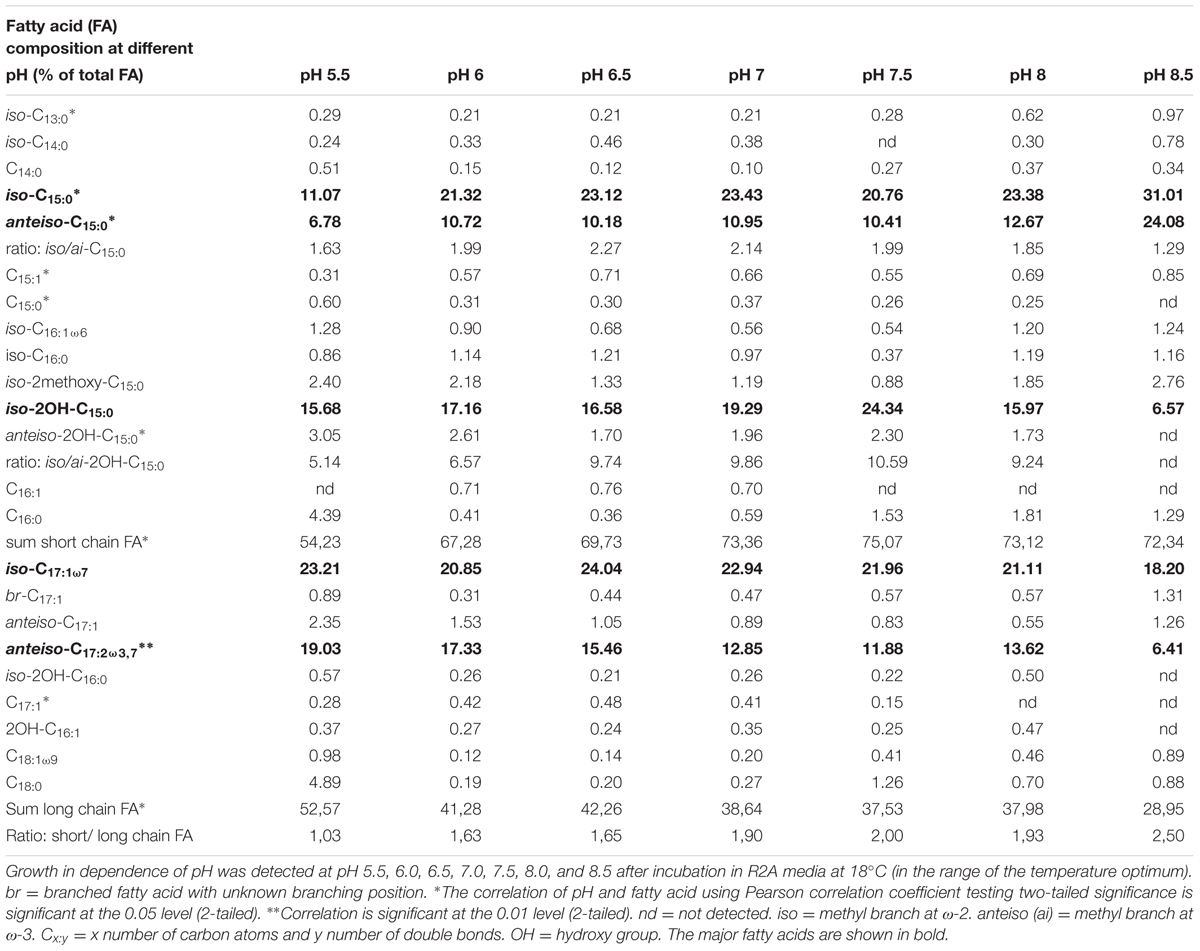
TABLE 2. Adaption of the cell membrane derived fatty acid inventory of C. frigidisoli PB4T to different pH conditions.
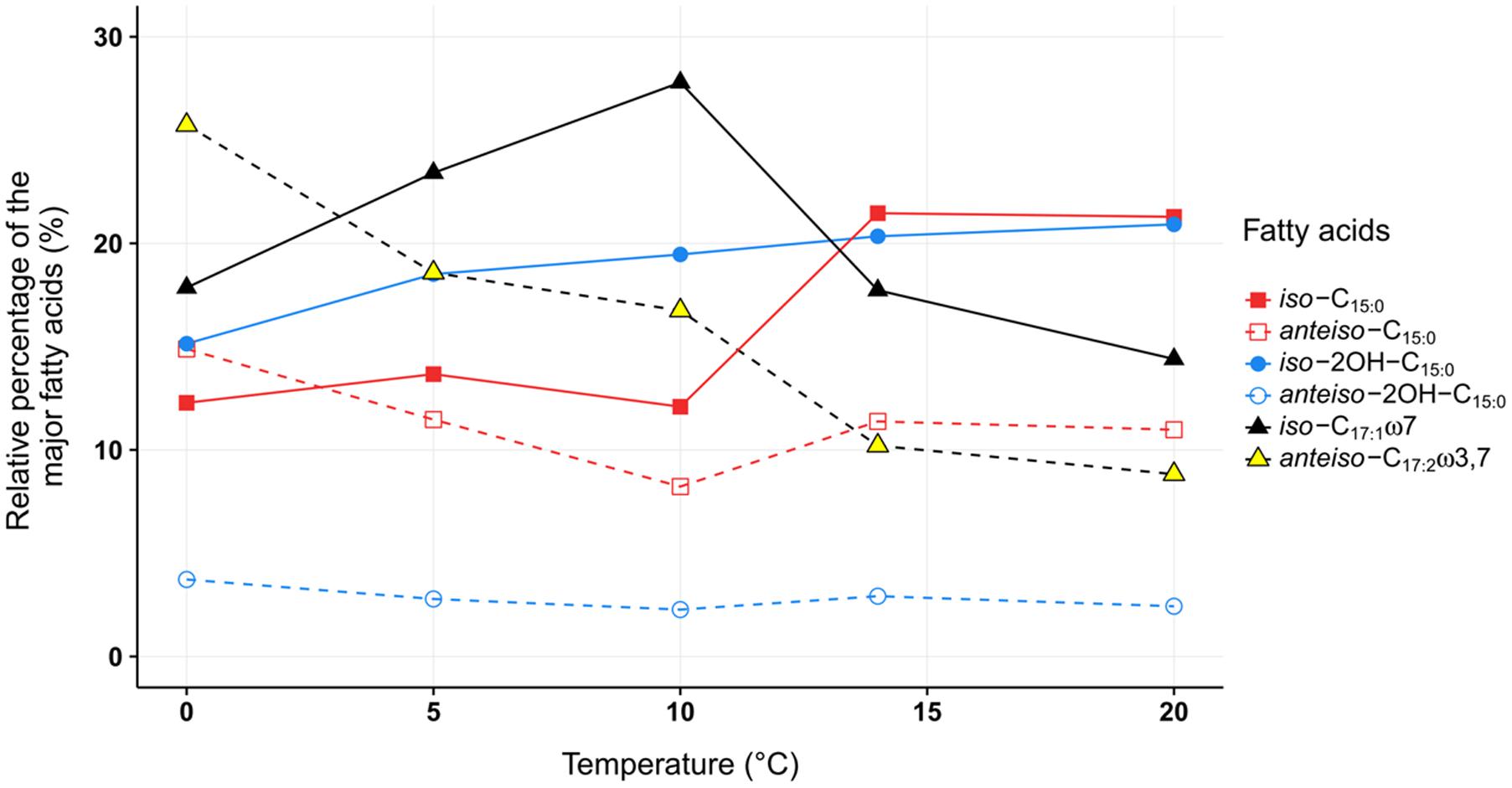
FIGURE 4. The relative proportion of the major membrane fatty acids from C. frigidisoli PB4T cultivated at different temperatures. While between 20°C and 14°C only minor variations within the relative proportions of the individual fatty acids were shown, a significant shift from saturated iso-C15:0 to unsaturated iso-C17:1ω7 between 14°C and 10°C was observed and below 10°C all iso-fatty acids decrease while the anteiso-fatty acids are increasing. iso = methyl branch at ω-2. anteiso = methyl branch at ω-3. Cx:y = x number of carbon atoms and y number of double bonds. OH = hydroxy group.
Overall, the fatty acid inventory of C. frigidisoli is characterized by branched (iso/anteiso) and unsaturated fatty acids in the shorter chain range between 15 and 17 carbon atoms. These features are used for instance by cells that are able to grow under harsh living conditions such as low temperatures or changing biogeochemical gradients to maintain membrane fluidity and functionality (Denich et al., 2003). By those means C. frigidisoli is well adapted to a psychrotolerant life style, because shorter chain fatty acids, branches and unsaturations increase the fluidity of the cell membrane at low temperatures (Russell, 1984). Furthermore, the whole genus Chryseobacterium is characterized by the presence of branched C15 and C17 fatty acids (Vandamme et al., 1994). The incorporation of anteiso-fatty acids, as it is shown in this study or for other psychrotolerant species such as C. frigidum (Kim et al., 2016) and C. haifense (Hantsis-Zacharov and Halpern, 2007) lowers the melting temperature of the cell membrane enhancing motion ability. Anteiso-C15:0 (25.5°C) for instance has a critical lower main phase transition temperature than iso-C15:0 (52.2°C; Suutari and Laakso, 1994). The incorporation of an unsaturation leads to an even stronger decrease in the melting temperature, while for instance a C16:0 fatty acid has a solid-liquid phase transition temperature of 63°C, whereas C16:1ω7 has a melting temperature of –1°C (Knothe and Dunn, 2009). In line with this, C. antarcticum incorporates iso-C15:1 as the major fatty acid and C. frigidisoli and its closest relatives C. humi (Pires et al., 2010) and C. marinum (Lee et al., 2007) contain various unsaturated C17 fatty acids in their cell membranes. In contrast, saturated and longer chain fatty acids (>C17), representing adaptation to warmer conditions, play only a minor role in the fatty acid inventory of C. frigidisoli. In general, the genus Chryseobacterium comprises several psychrotolerant species isolated from cold-affected habitats indicating that Chryseobacterium have developed suitable genetic, biochemical, and physiological adaptations for a life at low temperature (Yi et al., 2005; Zhao et al., 2011; Bajerski et al., 2013).
Fatty Acid Adaption to Variable Temperatures
As discussed in the previous paragraph the cell membrane of C. frigidisoli predominately contains branched shorter chained fatty acids partly with unsaturation as an adaptation to a psychrotolerant lifestyle. In the current study, we conducted temperature cultivation experiments at 0, 5, 10, 14, and 20°C. Between 20°C and 14°C the relative proportions of the fatty acids are not much different, however, below 14°C drastic changes are visible (Figure 4). This pattern is in accordance with the growth rate curve (Figure 1A) showing a significant change in the growth rate at 10°C. Generally, the fatty acid inventories at different cultivation temperatures are composed of the same fatty acids (Figure 2). However, there are differences in the relative distributions of the membrane fatty acids. While the anteiso-C15:0 is more abundant than the iso-congener in the 0°C culture, the ratio of iso- to anteiso-C15:0 changes drastically with increasing cultivation temperature with a clear dominance of the iso-C15:0 in the 20°C culture (Figure 4). This change is clearly resembled in the iso/anteiso-C15:0 ratio in Table 1. The relative proportion of iso-2OH-C15:0 constantly increases from the 0°C to the 20°C culture (Figure 4), while the anteiso-2OH-C15:0 shows an overall slight decrease between 0°C and 10°C and a more or less constant trend between 10°C and 20°C (Figure 4). The ratio of iso/anteiso-2OH-C15:0 (Table 1) shows overall an increasing trend from 0°C to 20°C, however, the ratio value of the 10°C culture falls out of this trend due to the lower amount of anteiso-2OH-C15:0. The change of the iso/anteiso-ratio allows the organism to adapt the cell membrane melting temperature in response to different ambient temperatures. The importance of anteiso-branched fatty acids at low temperatures was also reported in the temperature adaptation of Bacillus subtilis by Klein et al. (1999). They observed a relative dominance of anteiso-C15:0 and anteiso-C17:0 in the fatty acid inventory in cold shock experiments with B. subtilis.
Another adaptation to ambient temperature can be the chain length of the membrane fatty acids, which can be reduced with decreasing temperatures, since the melting temperature of the respective membrane fatty acids decreases with shorter chain length (Russell, 1989; Suutari and Laakso, 1994). However, in our study on C. frigidisoli the chain length does not seem to be a major regulation mechanism (Table 1) in response to changing temperature regimes. The overall chain length (short vs. long chain length) does not change significantly with temperature. Nevertheless, the fatty acid inventory of C. frigidisoli generally contains a high amount of shorter-chained fatty acids and the species uses other mechanisms (iso/ai ratios, unsaturation) to maintain the cell membrane fluidity at low temperature. In contrast to the chain length, temperature adaptation via the relative abundance of unsaturated fatty acids seems to play a significant role. The highly abundant monounsaturated iso-C17:1ω7 fatty acid shows a strong increase from 20°C to 10°C, which can be interpreted as a temperature adaptation, since a higher relative proportion of unsaturated fatty acids lowers the membrane solid-liquid phase transition temperature (Russell, 1989), in strain PB4T leading to an increased fluidity of the cell membrane (Figures 2, 4). At the same time the iso-C15:0 strongly decreases. Thus, between 20 and 10°C there is a trend from iso-C15:0 to iso-C17:1ω7 (Figure 4). The iso-C17:1ω7 has indeed a longer chain length (increase of melting temperature) but the unsaturation (strong decrease in melting temperature) more than compensates the chain length effect and seems to be an adaptation towards lower ambient temperatures. Unsaturation of fatty acids is a very effective temperature adaptation, because it has a higher effect on lipid fluidity and can be either realized by de novo synthesis or modification (desaturation) of already existing fatty acids (Russell, 1984). The longer chain length of iso-C17:1ω7 might attenuate the adaptation effect introduced by the unsaturation. Below 10°C the relative amount of iso-C17:1ω7 decreases again. The same is observed for other iso-fatty acids. In contrast, the anteiso-fatty acids generally increase between 10°C and 0°C (Figure 4). Therefore, below 10°C the data indicate that there is an interchange from iso-fatty acids to more anteiso-fatty acids, which represents a clear adaptation to cooler conditions (Kaneda, 1991). This interchange point in the fatty acid composition is also shown in the growth curve (Figure 1A) indicating that C. frigidisoli experiences stress at temperatures ≤10°C. Additionally, the anteiso-C17:2ω3,7 continuously increases (Figures 2, 4) from 20°C to 0°C indicating a strong temperature adaptation of C. frigidisoli to lower ambient temperatures. The statistical analysis shows that this anteiso and bis-unsaturated fatty acid strongly correlates with temperature (p < 0.01, R2 = 0.93). Its relative proportion increases with decreasing temperature and accounts for the highest amount of all fatty acids (25.73%) at 0°C (Table 1 and Figures 2, 4). Thus, the anteiso-C17:3ω3,7 fatty acid seems to be an important and efficient regulation component for temperature adaption in C. frigidisoli. Detailed studies on changes in the PFLA profiles of microorganisms especially from extreme habitats are still rare and the discovery of the new fatty acid shows that the microorganisms develop various ways of stress response. There are several studies reporting the critical role of reducing the fatty acid chain length and altering the branching from iso to anteiso in reaction to low ambient temperatures (Paton et al., 1978; Annous et al., 1997). Russell (1984) stated that unsaturation is more effective than chain length reduction in low temperature adaptation. However, Mangelsdorf et al. (2009) showed that in microbial communities from Siberian permafrost the temperature regulation was mainly realized by chain length and the trend in the relative proportion of saturated and unsaturated fatty acids did not change significantly. Chain length and desaturation played also a minor role in the cold shock response of Bacillus subtilis and evidence for the dominance of anteiso branched fatty acids in the adaptation to low temperature was given (Klein et al., 1999). In our study, we observe a high importance of iso- and anteiso-branched fatty acids in cold adaption. Unsaturated fatty acids such as the iso-C17:1ω7 show a concerted interplay between these structural species at different temperatures most likely induced by different temperature stress levels. Additionally, the newly discovered bis-unsaturated fatty acid anteiso-heptadeca-9,13-dienoic acid plays a major role in the cell membrane temperature adaption of C. frigidisoli.
Fatty Acid Adaption to Shifting pH Values
For most of the major fatty acids (iso-C17:1ω7, iso-C15:0, anteiso-C15:0, anteiso-2OH-C15:0) of C. frigidisoli the relative abundance is rather constant at neutral pH with a plateau phase between pH 6.0 and 8 (Figure 5). An exception forms iso-2OH-C15:0, which shows a maximum at pH 7.5. At the same time membrane fatty acids at pH minima and maxima (Figure 1B) show different compositions and variable adaptation mechanisms (Figure 5). At pH 8.5 the saturated iso- and anteiso-C15:0 show a significant increase, while the unsaturated iso-C17:1ω7, anteiso-C17:3ω3,7 as well as the hydroxy fatty acids iso-2OH-C15:0 and anteiso-2OH-C15:0 are all decreasing. In contrast, at pH 5.5 iso-C17:1ω7, anteiso-C17:3ω3,7 and anteiso-2OH-C15:0 increase, while iso- and anteiso-C15:0 decrease. The iso-2OH-C15:0 also decrease at lower pH. Thus, some opposite compositional trends can be observed at the different pH extremes outlined by the growth range of C. frigidisoli (Figure 1B).
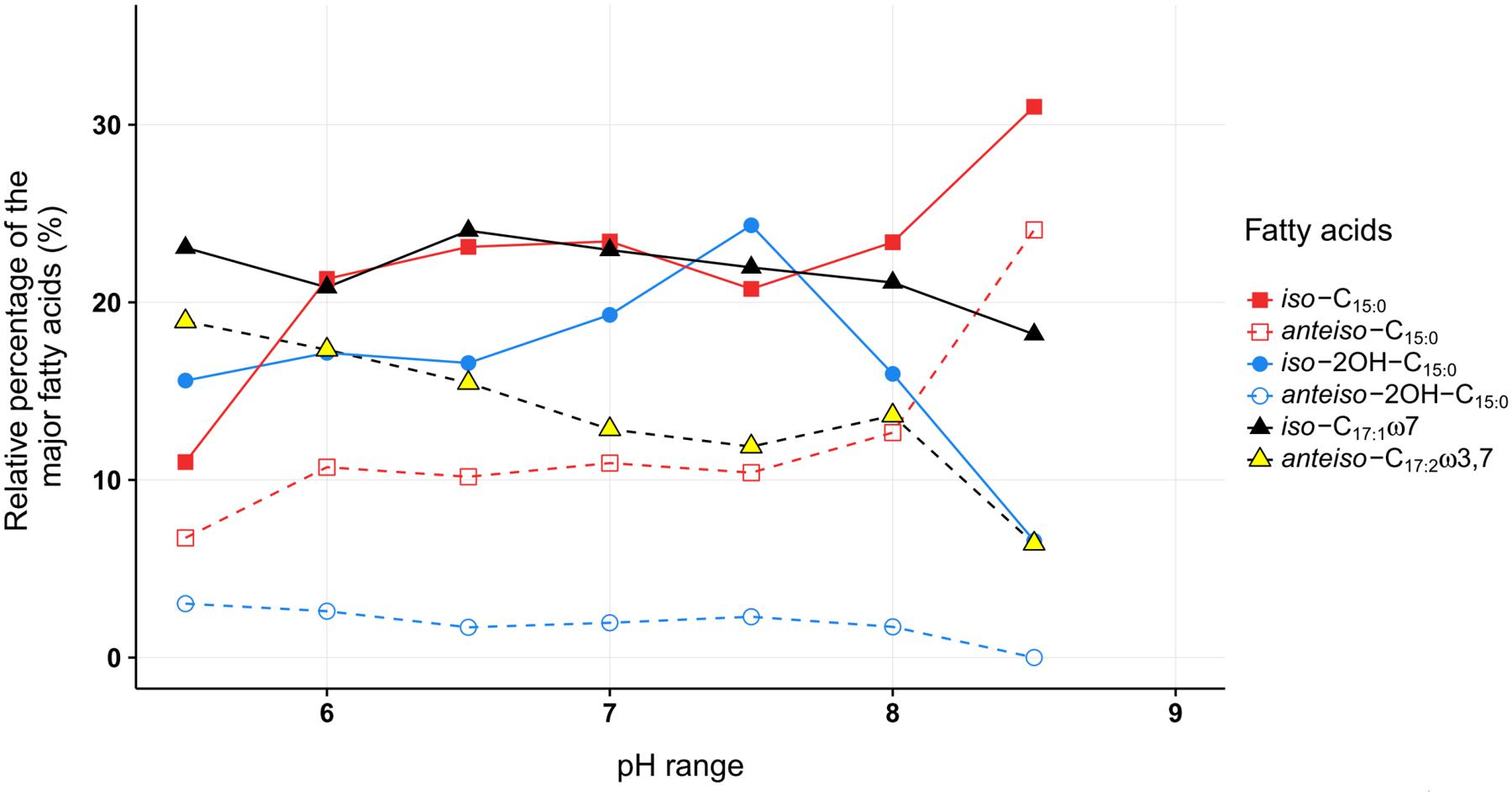
FIGURE 5. The relative proportions of the major fatty acids from C. frigidisoli PB4T cultivated at different pH conditions. The pH adaption is characterized by a relatively uniform fatty acid composition at neutral pH and significant variations mainly at the pH extremes. iso = methyl branch at ω-2. anteiso = methyl branch at ω-3. Cx:y = x number of carbon atoms and y number of double bonds. OH = hydroxy group.
Overall, the ratio of iso- to anteiso-C15:0 is high at neutral pH (dominance of iso-congener) and regardless to the increasing or decreasing trend of the iso- and anteiso-congeners the ratio becomes lower towards the pH extremes indicating an increasing relative importance of the anteiso-branched fatty acids in response to high and low pH (Table 2). The introduction of more anteiso-branched fatty acids supports a higher flexibility of the cell membrane (Russell, 1989). The main challenge for the microorganism in an acidic or alkaline environment is the change of the ion strength that influences proton motive force of the cell membrane. The maintenance of the transmembrane electrical potential (Δψ) and the transmembrane ΔpH is mainly realized using transporters including active proton transport (Krulwich et al., 2011). A higher flexibility of the cell membrane could enable the incorporation of new membrane channels generating the ion transport through the cell membrane in reaction to the changing ion strength at different pH values.
Furthermore, different branching patterns in response to changing pH were discussed in literature before, which partly disagree with the results of the current study. Bååth and Anderson (2003) reported a decrease of iso-C15:0 and iso-C16:0 at increasing pH and no significant effect of the anteiso-branched fatty acids, while Männistö et al. (2007) observed an increase of anteiso-C15:0 and no change of iso-C15:0 at higher pH in bacterial communities in Arctic Fjelds of Finnish Lapland. Furthermore, an increase of branched (iso, anteiso) fatty acids was observed in response to low pH (Russell, 1984). In our study the relative proportions of the iso- and anteiso-C15:0 increases at high pH and, interestingly, those of iso- and anteiso-2OH-C15:0 decrease (Table 2 and Figures 3, 5). Thus, the hydroxy group as the only structural difference among these branched C15:0 fatty acids seems to be less favorable for adaptation towards higher pH conditions. In contrast at pH 5.5 the iso-C15:0 and anteiso-C15:0 fatty acids significantly decrease, while the hydroxy congeners only slightly decrease (iso) or increase (anteiso). To our knowledge, this is the first study showing a pH dependent change in the relative amount of methyl and hydroxy-side chains of the fatty acids and we can only assume that an additional hydroxy group is somehow less favorable at alkaline pH in contrast to a lower pH. For acidophilic archaea it was shown that hydroxy groups on sugar moieties prevent the protons from penetrating the cell membrane at low pH (Wang et al., 2012). This shows that a hydroxylation might be a suitable adaptation mechanism in an acidic environment, which could explain why the relative proportion of the iso- and anteiso-2OH-C15:0 is only less affected at lower pH.
Another interesting observation concerns the chain length of the respective fatty acids. C. frigidisoli incorporates significantly more short chain fatty acids, predominantly C15, at higher pH (Figures 3, 5). The unsaturated iso-C17:1ω7 and the anteiso-C17:2ω3,7 significantly decrease at higher pH (Table 2 and Figure 2). At low pH the opposite trend can be observed, while iso-C15:0, anteiso-C15:0, iso-2OH-C15:0 all decrease (anteiso-2OH-C15:0 stays rather constant), iso-C17:1ω7 and anteiso-C17:2ω3,7 increase (Figure 5). A similar shift from short-chained saturated (pH 7) to long-chained mono-unsaturated fatty acids with decreasing pH was observed in Streptococcus mutans and other oral bacteria as a survival strategy in acidic environment, however, the function of this shift is currently not understood (Quivey et al., 2000; Fozo et al., 2004).
Similar to the temperature cultivation experiments, the anteiso-C17:2ω3,7 fatty acid also seems to play a role in the cell membrane adaption at different pH conditions. With exception of the culture from pH 8, this fatty acid constantly decreases with increasing pH.
In contrast to the temperature adaptation, interpretation of the adaptation mechanisms concerning changing pH condition is rather difficult; in particular, since literature data are somehow contradicting and show no similar trends. Thus, the function of the observed fatty acid variations in the current study is not clear yet. At high pH the decrease in the long chain unsaturated fatty acids seem to support a compaction/stabilization of the cell membrane whereas the concomitantly shift to more short chain iso- and anteiso-C15:0 with a relative increase of the anteiso-congener seem to attenuate this effect. At low pH the opposite trend may increase the membrane fluidity. Thus, the pH adaptation of the cell membrane derived fatty acid inventory appears to be a balanced interplay between stabilization and enhanced flexibility of the cell membrane presumably to either prevent or enable protons or other homeostatically active substances passing the cell membrane. Further studies are needed to discover the interrelation between the structural membrane adaptation and the effect on the membrane function at changing pH conditions.
Conclusion
In its natural Antarctic habitat C. frigidisoli PB4T is exposed to extreme low temperatures and changing geochemical gradients. Temperatures fluctuate from summer to winter and especially during diurnal and nocturnal shifts. Geochemical parameters are shaped by a slow succession and severe climatic conditions such as strong winds.
The adaptation of C. frigidisoli to low temperatures shows a complex interplay of membrane derived fatty acid variations depending on different stress levels. While between 20°C and 14°C only minor variations within the relative proportions of the individual fatty acids was shown, a significant shift from saturated iso-C15:0 to unsaturated iso-C17:1ω7 between 14°C and 10°C was observed and below 10°C all iso-fatty acids decrease while the anteiso-fatty acids are increasing. All changes in the cell membrane fatty acid inventory represent a clear adaptation to highly fluctuating temperature conditions in the Antarctic environment. Another important finding is the specific role of the recently identified anteiso-heptadec-9,13-enoic fatty acid for the temperature adaptation in C. frigidisoli, since this fatty acid continuously increases with decreasing temperature and is the dominant fatty acid at 0°C.
The pH adaption is characterized by a relatively uniform fatty acid composition at neutral pH and significant variations mainly at the pH extremes. At high pH a shift from the unsaturated long chain iso- and anteiso-fatty acids to the saturated short chain iso- and anteiso-fatty acids is observed while at low pH the opposite trends are visible. These structural differences point to a strengthening of the cell membrane at high pH and a higher fluidity at low pH presumably to enable or prevent exchange processes with the surrounding environment.
Among the importance of the newly identified fatty acid for the temperature adaptation and survival of C. frigidisoli in the extreme Antarctic environment, this fatty acid is suggested to serve as a biomarker for the genus Chryseobacterium (Mangelsdorf et al., 2017).
Author Contributions
DW and FB designed the study. FB performed a part of the lab work (microbial physiology) and mainly analyzed and interpreted the data. KM performed the fatty acid analyses of the strain. DW and KM substantial contributed to the interpretation of the results and valuable discussion. FB was drafting the work and DW and KM revised it critically for important intellectual content. FB, DW, and KM finally approved the version to be published and agreed to be accountable for all aspects of the work in ensuring that questions related to the accuracy or integrity of any part of the work are appropriately investigated and resolved.
Funding
This study was supported by the Deutsche Forschungsgemeinschaft (DFG) in the framework of the priority program ‘Antarctic Research with Comparative Investigations in Arctic Ice Areas’ by a grant to DW (WA 1554/9).
Conflict of Interest Statement
The authors declare that the research was conducted in the absence of any commercial or financial relationships that could be construed as a potential conflict of interest.
Acknowledgments
The authors wish to thank the shipboard scientific party, in particular H.-W. Hubberten (Alfred Wegener Institute, Helmholtz Centre for Polar and Marine Research, AWI), of the expedition ANT-XXIII/9 in 2007 with RV Polarstern. We also thank the reviewers JSL and SV for constructive comments. Special thanks go to Lars Ganzert (Leibniz-Institute of Freshwater Ecology and Inland Fisheries, IGB) for successful field work, Lisa Padur (AWI), Oliver Burckhardt and Cornelia Karger (German Research Centre for Geosciences, GFZ) for lab assistance and Susanne Liebner (GFZ) for critical reading of the manuscript. The presented data were part of the dissertation thesis of FB at the University of Potsdam (Bajerski, 2013).
References
Abell, G. C. J., and Bowman, J. P. (2005). Ecological and biogeographic relationships of class Flavobacteria in the Southern Ocean. FEMS Microbiol. Ecol. 51, 265–277. doi: 10.1016/j.femsec.2004.09.001
Annous, B. A., Becker, L. A., Bayles, D. O., Labeda, D. P., and Wilkinson, B. J. (1997). Critical role of anteiso-C15:0 fatty acid in the growth of Listeria monocytogenes at low temperatures. Appl. Environ. Microbiol. 63, 3887–3894.
Bååth, E., and Anderson, T. H. (2003). Comparison of soil fungal/bacterial ratios in a pH gradient using physiological and PLFA-based techniques. Soil Biol. Biochem. 35, 955–963. doi: 10.1016/S0038-0717(03)00154-8
Bajerski, F. (2013). Bacterial Communities in Glacier Forefields of the Larsemann Hills, East Antarctica: Structure, Development & Adaptation; Bakterielle Gemeinschaften in Gletschervorfeldern der Larsemann Berge der Ostantarktis: Struktur, Entwicklung & Anpassung. Doctoral thesis, University of Potsdam, Potsdam.
Bajerski, F., Ganzert, L., Mangelsdorf, K., Padur, L., Lipski, A., and Wagner, D. (2013). Chryseobacterium frigidisoli sp. nov., a psychrotolerant species of the family Flavobacteriaceae isolated from sandy permafrost from a glacier forefield. Int. J. Syst. Evol. Microbiol. 63, 2666–2671. doi: 10.1099/ijs.0.046904-0
Bajerski, F., and Wagner, D. (2013). Bacterial succession in Antarctic soils of two glacier forefields on Larsemann Hills. East Antarctica. FEMS Microbiol. Ecol. 85, 128–142. doi: 10.1111/1574-6941.12105
Bakermans, C., Bergholz, P. W., Rodrigues, D. F., Vishnivetskaya, T. A., Ayala-del-Rio, H. L., and Tiedje, J. M. (2012). “Genomic and expression analyses of cold-adapted microorganisms,” in Polar Microbiology - Life in a Deep Freeze, eds R. V. Miller and L. G. Whyte (Washington, DC: ASM Press), 126–155.
Bligh, E. G., and Dyer, W. J. (1959). A rapid method of total lipid extraction and purification. Can. J. Biochem. Physiol. 37, 911–917. doi: 10.1139/o59-099
Boggs, J. M. (1986). Effect of lipid structural modifications on their intermolecular hydrogen bonding interactions and membrane functions. Biochem. Cell Biol. 64, 50–57. doi: 10.1139/o86-008
Brown, J. L., Ross, T., McMeekin, T. A., and Nichols, P. D. (1997). Acid habituation of Escherichia coli and the potential role of cyclopropane fatty acids in low pH tolerance. Int. J. Food Microbiol. 37, 163–173. doi: 10.1016/S0168-1605(97)00068-8
Cannone, N., Wagner, D., Hubberten, H. W., and Guglielmin, M. (2008). Biotic and abiotic factors influencing soil properties across a latitudinal gradient in Victoria Land. Antarctica. Geoderma 144, 50–65. doi: 10.1016/j.geoderma.2007.10.008
Cary, S. C., McDonald, I. R., Barrett, J. E., and Cowan, D. A. (2010). On the rocks: the microbiology of Antarctic Dry Valley soils. Nat. Rev. Microbiol. 8, 129–138. doi: 10.1038/nrmicro2281
Denich, T. J., Beaudette, L. A., Lee, H., and Trevors, J. T. (2003). Effect of selected environmental and physico-chemical factors on bacterial cytoplasmic membranes. J. Microbiol. Methods 52, 149–182. doi: 10.1016/S0167-7012(02)00155-0
Fozo, E. M., Kajfasz, J. K., and Quivey, R. G. (2004). Low pH-induced membrane fatty acid alterations in oral bacteria. FEMS Microbiol. Lett. 238, 291–295. doi: 10.1111/j.1574-6968.2004.tb09769.x
Ganzert, L., Lipski, A., Hubberten, H.-W., and Wagner, D. (2011). The impact of different soil parameters on the community structure of dominant bacteria from nine different soils located on Livingston Island, South Shetland Archipelago, Antarctica. FEMS Microbiol. Ecol. 76, 476–491. doi: 10.1111/j.1574-6941.2011.01068.x
Georlette, D., Blaise, V., Collins, T., D’Amico, S., Gratia, E., Hoyoux, A., et al. (2004). Some like it cold: biocatalysis at low temperatures. FEMS Microbiol. Rev. 28, 25–42. doi: 10.1016/j.femsre.2003.07.003
Gugliandolo, C., Michaud, L., Lo Giudice, A., Lentini, V., Rochera, C., Camacho, A., et al. (2016). Prokaryotic community in lacustrine sediments of byers Peninsula (Livingston Island, Maritime Antarctica). Microb. Ecol. 71, 387–400. doi: 10.1007/s00248-015-0666-8
Hantsis-Zacharov, E., and Halpern, M. (2007). Chryseobacterium haifense sp. nov., a psychrotolerant bacterium isolated from raw milk. Int. J. Syst. Evol. Microbiol. 57, 2344–2348. doi: 10.1099/ijs.0.65115-0
Hoaki, T., Nishijima, M., Kato, M., Adachi, K., Mizobuchi, S., Hanzawa, N., et al. (1994). Growth requirements of hyperthermophilic sulfur-dependent heterotrophic archaea isolated from a shallow submarine geothermal system with reference to their essential amino acids. Appl. Environ. Microbiol. 60, 2898–2904.
Hodgson, D. A., Noon, P. E., Vyverman, W., Bryant, C. L., Gore, D. B., Appleby, P., et al. (2001). Were the Larsemann Hills ice-free through the Last Glacial Maximum? Antarct. Sci. 13, 440–454. doi: 10.1017/S0954102001000608
Kaneda, T. (1991). Iso- and anteiso-fatty acids in bacteria: biosynthesis, function, and taxonomic significance. Microbiol. Rev. 55, 288–302.
Kim, T., Kim, M., Kang, O., Jiang, F., Chang, X., Liu, P., et al. (2016). Chryseobacterium frigidum sp. nov., isolated from high-Arctic tundra soil, and emended descriptions of Chryseobacterium bernardetii and Chryseobacterium taklimakanense. Int. J. Syst. Evol. Microbiol. 66, 609–615. doi: 10.1099/ijsem.0.000761
Klein, W., Weber, M. H. W., and Marahiel, M. A. (1999). Cold shock response of Bacillus subtilis: isoleucine-dependent switch in the fatty acid branching pattern for membrane adaptation to low temperatures. J. Bacteriol. 181, 5341–5349.
Knothe, G., and Dunn, R. O. (2009). A comprehensive evaluation of the melting points of fatty acids and esters determined by differential scanning calorimetry. J. Am. Oil Chem. Soc. 86, 843–856. doi: 10.1007/s11746-009-1423-2
Krulwich, T. A., Sachs, G., and Padan, E. (2011). Molecular aspects of bacterial pH sensing and homeostasis. Nat. Rev. Microbiol. 9, 330–343. doi: 10.1038/nrmicro2549
Lee, K., Lee, H. K., Choi, T.-H., and Cho, J.-C. (2007). Sejongia marina sp. nov., isolated from Antarctic seawater. Int. J. Syst. Evol. Microbiol. 57, 2917–2921. doi: 10.1099/ijs.0.65279-0
Mangelsdorf, K., Bajerski, F., Karger, C., and Wagner, D. (2017). Identification of a novel fatty acid in the cell membrane of Chryseobacterium frigidisoli PB4T isolated from an East Antarctic glacier forefield. Org. Geochem. 106, 68–75. doi: 10.1016/j.orggeochem.2017.01.003
Mangelsdorf, K., Finsel, E., Liebner, S., and Wagner, D. (2009). Temperature adaptation of microbial communities in different horizons of Siberian permafrost-affected soils from the Lena Delta. Chem. Erde Geochem. 69, 169–182. doi: 10.1016/j.chemer.2009.02.001
Männistö, M. K., Tiirola, M., and Häggblom, M. M. (2007). Bacterial communities in Arctic fjelds of Finnish Lapland are stable but highly pH-dependent. FEMS Microbiol. Ecol. 59, 452–465. doi: 10.1111/j.1574-6941.2006.00232.x
Müller, K. D., Husmann, H., and Nalik, H. P. (1990). A new and rapid method for the assay of bacterial fatty acids using high resolution capillary gas chromatography and trimethylsulfonium hydroxide. Zentralbl. Bakteriol. 274, 174–182. doi: 10.1016/S0934-8840(11)80100-3
Paton, J. C., McMurchie, E. J., May, B. K., and Elliott, W. H. (1978). Effect of growth temperature on membrane fatty acid composition and susceptibility to cold shock of Bacillus amyloliquefaciens. J. Bacteriol. 135, 754–759.
Pires, C., Carvalho, M. F., De Marco, P., Magan, N., and Castro, P. M. L. (2010). Chryseobacterium palustre sp. nov. and Chryseobacterium humi sp. nov., isolated from industrially contaminated sediments. Int. J. Syst. Evol. Microbiol. 60, 402–407. doi: 10.1099/ijs.0.010348-0
Quivey, R. G., Faustoferri, R., Monahan, K., and Marquis, R. (2000). Shifts in membrane fatty acid profiles associated with acid adaptation of Streptococcus mutans. FEMS Microbiol. Lett. 189, 89–92. doi: 10.1111/j.1574-6968.2000.tb09211.x
Reasoner, D. J., and Geldreich, E. E. (1985). A new medium for the enumeration and subculture of bacteria from potable water. Appl. Environ. Microbiol. 49, 1–7.
Rothschild, L. J., and Mancinelli, R. L. (2001). Life in extreme environments. Nature 409, 1092–1101. doi: 10.1038/35059215
Russell, N. J. (1984). Mechanisms of thermal adaptation in bacteria: blueprints for survival. Trends Biochem. Sci. 9, 108–112. doi: 10.1016/0968-0004(84)90106-3
Russell, N. J. (1989). “Function of lipids: structural roles and membrane functions,” in Microbial Lipids, eds C. Ratledge and S. G. Wilkinson (London: Academic Press), 279–365.
Sinensky, M. (1974). Homeoviscous adaptation—a homeostatic process that regulates the viscosity of membrane lipids in Escherichia coli. Proc. Natl. Acad. Sci. U.S.A. 71, 522–525. doi: 10.1073/pnas.71.2.522
Singer, S. J. (1972). A fluid lipid-globular protein mosaic model of membrane structure. Ann. N. Y. Acad. Sci. 195, 16–23. doi: 10.1111/j.1749-6632.1972.tb54780.x
Stüwe, K., Braun, H. M., and Peer, H. (1989). Geology and structure of the Larsemann Hills area, Prydz Bay, East Antarctica. Aust. J. Earth Sci. 36, 219–241. doi: 10.1080/08120098908729483
Suutari, M., and Laakso, S. (1994). Microbial fatty acids and thermal adaptation. Crit. Rev. Microbiol. 20, 285–328. doi: 10.3109/10408419409113560
Teixeira, L. C. R. S., Peixoto, R. S., Cury, J. C., Sul, W. J., Pellizari, V. H., Tiedje, J., et al. (2010). Bacterial diversity in rhizosphere soil from Antarctic vascular plants of Admiralty Bay, maritime Antarctica. ISME J. 4, 989–1001. doi: 10.1038/ismej.2010.35
Vandamme, P., Bernadet, J.-F., Segers, P., Kersters, K., and Holmes, B. (1994). NOTES: new perspectives in the classification of the flavobacteria: description of Chryseobacterium gen. nov., Bergeyella gen. nov., and Empedobacter nom. rev. Int. J. Syst. Bacteriol. 44, 827–831. doi: 10.1099/00207713-44-4-827
Wang, X., Lv, B. E., Cai, G., Fu, L., Wu, Y., Wang, X., et al. (2012). A proton shelter inspired by the sugar coating of acidophilic archaea. Sci. Rep. 2:892. doi: 10.1038/srep00892
Wynn-Williams, D. D. (1996). Antarctic microbial diversity: the basis of polar ecosystem processes. Biodivers. Conserv. 5, 1271–1293. doi: 10.1007/bf00051979
Yi, H., Yoon, H. I., and Chun, J. (2005). Sejongia antarctica gen. nov., sp. nov. and Sejongia jeonii sp. nov., isolated from the Antarctic. Int. J. Syst. Evol. Microbiol. 55, 409–416. doi: 10.1099/ijs.0.63273-0
Zdanowski, M. K., Żmuda-Baranowska, M. J., Borsuk, P., Świcccatecki, A., Górniak, D., Wolicka, D., et al. (2013). Culturable bacteria community development in postglacial soils of Ecology Glacier, King George Island, Antarctica. Polar Biol. 36, 511–527. doi: 10.1007/s00300-012-1278-0
Zhao, Q., Bai, Y., Zhang, G., Zhu, S., Sheng, H., Sun, Y., et al. (2011). Chryseobacterium xinjiangense sp. nov., isolated from alpine permafrost. Int. J. Syst. Evol. Microbiol. 61, 1397–1401. doi: 10.1099/ijs.0.024141-0
Keywords: bacterial cell membrane, fatty acid composition, cold temperature, physiological adaptation, Flavobacteriaceae, biogeochemical gradients, permafrost
Citation: Bajerski F, Wagner D and Mangelsdorf K (2017) Cell Membrane Fatty Acid Composition of Chryseobacterium frigidisoli PB4T, Isolated from Antarctic Glacier Forefield Soils, in Response to Changing Temperature and pH Conditions. Front. Microbiol. 8:677. doi: 10.3389/fmicb.2017.00677
Received: 21 January 2017; Accepted: 03 April 2017;
Published: 19 April 2017.
Edited by:
Axel Schippers, Federal Institute for Geosciences and Natural Resources, GermanyReviewed by:
Julius Sebastian Lipp, University of Bremen, GermanySurendra Vikram, University of Pretoria, South Africa
Copyright © 2017 Bajerski, Wagner and Mangelsdorf. This is an open-access article distributed under the terms of the Creative Commons Attribution License (CC BY). The use, distribution or reproduction in other forums is permitted, provided the original author(s) or licensor are credited and that the original publication in this journal is cited, in accordance with accepted academic practice. No use, distribution or reproduction is permitted which does not comply with these terms.
*Correspondence: Felizitas Bajerski, felizitas.bajerski@dsmz.de
†Present address: Felizitas Bajerski, Leibniz Institute DSMZ, German Collection of Microorganisms and Cell Cultures, Braunschweig, Germany