- Microbiology Program, Department of Biological Sciences, Faculty of Science, Kuwait University, Safat, Kuwait
The aim of this study was to explore the heavy-metal resistance and hydrocarbonoclastic potential of microorganisms in a hypersaline soil. For this, hydrocarbonoclastic microorganisms were counted on a mineral medium with oil vapor as a sole carbon source in the presence of increasing concentrations of ZnSO4, HgCl2, CdSO4, PbNO3, CuSO4, and Na2HAsO4. The colony-forming units counted decreased in number from about 150 g-1 on the heavy-metal-free medium to zero units on media with 40–100 mg l-1 of HgCl2, CdSO4, PbNO3, or Na2HAsO4. On media with CuSO4 or ZnSO4 on the other hand, numbers increased first reaching maxima on media with 50 mg l-1 CuSO4 and 90 mg l-1 ZnSO4. Higher concentrations reduced the numbers, which however, still remained considerable. Pure microbial isolates in cultures tolerated 200–1600 mg l-1 of HgCl2, CdSO4, PbNO3, CuSO4, and Na2HAsO4 in the absence of crude oil. In the presence of oil vapor, the isolates tolerated much lower concentrations of the heavy metals, only 10–80 mg l-1. The addition of 10 Fe2(SO4)3 and 200 mg l-1 proline (by up to two- to threefold) enhanced the tolerance of several isolates to heavy metals, and consequently their potential for oil biodegradation in their presence. The results are useful in designing bioremediation technologies for oil spilled in hypersaline areas.
Introduction
Oil started to be extensively produced and used as energy and raw-material sources since about 80 years. This was associated with increasing rates of pollution of terrestrial, aquatic, and atmospheric environments with spilled oil, oil vapor, and processed oil products. It has been estimated that alone the marine environment is charged yearly with 10 Mt of hydrocarbon pollutants (Banerjee et al., 2006). As expected, pollution rates are particularly high in oil-producing countries such as the Arabian Gulf states. The Gulf water-body is used by vessels that carry about 50% of the marine-transported oil worldwide (Hunter, 1982). Those states belong geographically to the semiarid region and are characterized by harsh climates. For example, the temperature in the small state of Kuwait during the long, dry summer frequently exceeds 50°C. Under such harsh conditions, oil bioremediation and self-cleaning via activities of nonextremophilic microorganisms are minimal (Margesin and Schinner, 2001; Oren, 2002). At such high temperatures, coastal seawaters trapped during tidal movement lose water by excessive evaporation, and the respective areas become thus hypersaline with NaCl concentrations reaching 4 M and higher. Like elsewhere (Lefebvre and Moletta, 2006), the hypersaline coastal areas in Kuwait are subjected to contamination with spilled oil. Bioremediation of such polluted areas cannot be achieved by nonextremophilic microorganisms (Pieper and Reineke, 2000; Oren, 2002); only halophilic/halotolerant microorganisms could be effective.
Halophilic/halotolerant microorganisms with hydrocarbon-utilization potential were recorded and isolated from hypersaline areas all over the globe (Oren et al., 1992; Emerson et al., 1994; Margesin and Schinner, 2001; Garcia et al., 2004; Nicholson and Fathepure, 2005; Zhao et al., 2009; Bonfa et al., 2011; Fathepure, 2014). Those hydrocarbonoclastic halophiles include prokaryotes (bacteria and archaea) and eukaryotes (yeasts and molds). Our group in Kuwait contributed to such studies and published extensive information on numbers, identities, and hydrocarbonoclastic potential of hydrocarbonoclastic microorganisms from hypersaline coastal areas in this region (Al-Mailem et al., 2012, 2014a). Emphasis was put on the feasibility of using these indigenous microorganisms in bioremediation and self-cleaning of local oil spills. The Gulf microorganisms naturally are stressed, not only by high salinity, harsh climatic conditions, and oil spills, but also by toxic heavy metals that may be associated with crude oil (Osuji and Onojake, 2004; Basumatary et al., 2012). Within this context, toxicity and resistance of heavy metals have been studied using predominantly nonextremophilic microorganisms (for review, see Nies, 1999). Those metals have a density above 5 g cm-3 (atomic weights > 50 amu) (Weast, 1984). They potentially form unspecific complex compounds in the cell which leads to toxicity. Yet, some heavy metals are essential trace elements. Two systems for heavy-metal uptake are known. The fast, unspecific system which is constitutively expressed; it depends solely on concentration gradient force. The second is the slow uptake system, with high substrate specificity in which ATP is consumed. Heavy-metal toxicity arises when the heavy-metal cations bind to SH groups leading to the inhibition of the activity of sensitive (especially respiratory) enzymes. Ions may also bind with O2 leading to oxidative stress. Three mechanisms for heavy-metal resistance are known. The first involves the active extrusion (efflux) of ions from the cell. The second is the segregation of cations (especially the S-lovers) into complex compounds by thiol-containing molecules. Thirdly, metal ions may be reduced to a less toxic oxidation (sometimes volatile, e.g., Hgo) state.
Within a research project on the bioremediation of oily hypersaline regions in Kuwait, we reported recently on biostimulation of pure, hydrocarbonoclastic microorganisms from those habitats by the addition of cations including Fe3+ (Al-Mailem et al., 2017). The major objective of this current study was to elaborate on the previous work by investigating the sensitivity and tolerance of the same microorganisms to common heavy metals, and to measure the effects of individual heavy metals on crude-oil removal by such microorganisms under salt stress. In other words, the main aim was jointly exploring the heavy-metal tolerance and hydrocarbon-degrading potential of novel halotolerant/halophilic isolates from hypersaline coastal soil in Kuwait. We started this study by counting the numbers of colony-forming units (CFU) of hydrocarbonoclastic, halophilic/halotolerant microorganisms for the hypersaline soil on media containing increasing concentrations of heavy metals. This part was followed by another one in which pure cultures from the hypersaline soil were used. It was also proposed to investigate whether or not, Fe3+ and proline (a compatible solute) may affect the microbial tolerance to the toxicity of individual heavy metals. Information gained from this research would help in designing biotechnologies for bioremediation of spilled oil in areas under multiple stresses. Within this context, our group recently found that calcium and dipicolinic acid (constituents of heat-tolerant endospores) biostimulated oil bioremediation under multiple stresses by heat, oil, and heavy metals (Radwan et al., 2017).
Materials and Methods
Counting Heavy-Metal-Tolerant Microorganisms in Hypersaline Soil
The aim of this experiment was to study the inhibition pattern of increasing concentrations of heavy metals on the numbers of CFU of halophilic/halotolerant, hydrocarbonoclastic microorganisms counted by the dilution-plating method. A solid (1.5% agar) mineral medium with oil vapor as sole source of carbon and energy (Sorkhoh et al., 1990) was used, it had the following composition (g l-1): 0.85 NaNO3, 0.56 KH2PO4, 0.86 Na2HPO4, 0.17 K2SO4, 0.37 MgSO4.7H2O, 0.7 CaCl2.2H2O, 2.5 ml of a trace element mixture consisting of (g l-1): 2.32 ZnSO4, 1.78 MnSO4, 0.56 H3BO3, 1.0 CuSO4, 0.39 Na2 MoO4, 0.42 CoCl2, 0.66 KI, 1.0 EDTA, 0.4 FeSO4, 0.004 NiCl2, pH 7.0. With oil vapor as a sole carbon and energy source, this medium obviously would support hydrocarbonoclastic microorganisms selectively. The medium salinity was adjusted to 2 M NaCl, and different concentrations of individual heavy-metal salts were added. Medium portions, 25 ml, were dispensed in sterile Petri dishes. After medium solidification at room temperature, inoculum aliquots, 0.25 ml [from down series of dilutions of the hypersaline soil (about 4 M NaCl) in sterile hypersaline water, about 3 M NaCl], were spread on the solid medium surfaces. Filter papers impregnated with 2 ml crude oil as source of oil vapor were fixed in dish lids, and the dishes were sealed. The heavy-metal salts tested were HgCl2, CdSO4, PbNO3, CuSO4, and Na2HAsO4 at concentrations from 0 (controls) to 300 mg l-1. Three replicates for each soil dilution and heavy-metal concentration were prepared. The plates were incubated at 30°C for 12 days (d) and the CFUs were counted and the mean numbers per gram soil were calculated.
Halophilic/Halotolerant, Hydrocarbonoclastic Microorganisms
Pure cultures of the four halophilic, hydrocarbonoclastic bacteria, Arhodomonas aquaeolei, Marinobacter lacisalsi, Halomonas axialensis, and Kocuria flava, and the two haloarchaea, Haloferax elongans and Halobacterium salinarum, that had been used in our earlier investigation (Al-Mailem et al., 2014a,b, 2017) were used in this study. These cultures had been isolated from the hypersaline coastal soil (about 4 M NaCl), about 90 km, south of Kuwait city, 10 km north of the Saudi Arabian borders, using the mineral medium with oil vapor as sole source of carbon and energy. Those isolates had been identified by comparing sequences of their 16S rRNA genes with those of type strains in the GenBank database. For this, total genomic DNA was extracted from the cells using the PrepMan Ultra Kit (Applied Biosystems, United States) and the 16S rRNA genes were amplified using the primer pairs GM5F and 907R (Santegoeds et al., 1998) for bacteria and the pairs of 0018F and 1518R for archaea (Cui et al., 2009). The PCR products were purified, sequenced, and obtained sequences were subjected to basic local alignment search tool analysis. The sequences were deposited in the GenBank database. A phylogenetic tree was constructed by neighbor joining including bootstrap analysis using PAUP∗ V.4 (Swofford, 1998).
Effects of Crude Oil, Fe2(SO4)3, and Proline on Heavy-Metal Tolerance by Pure Halophilic/Halotolerant Isolates
Heavy-metal tolerance is measured either as “maximum tolerated concentrations” (MTCs) or as “minimum inhibitory concentrations” (MIC). The MTC measurement was chosen here because it was more related to the objectives of this study than the MIC measurement.
For this experiment, saline (2 M NaCl) nutrient agar was used, once as such, and once with oil vapor in the dish head spaces. Obviously, the mineral medium with oil vapor as a sole carbon source is not suitable because in the absence of oil vapor, the sole carbon source, no microbial activity would occur at all. Aliquots of the media were provided with individual heavy-metal salts at concentrations of 0–1600 mg l-1. To study the effects of Fe2(SO4)3 (10 mg l-1) and proline (200 mg l-1) (those concentrations were based on the results of preliminary experiments and the concentrations used by earlier researchers), they were added, separately. Common inocula (one loopful of biomass in 5 ml of sterile hypersaline water) were prepared, and the medium aliquots were inoculated by streaking one loopful of the inoculum on medium surfaces. Triplicates were prepared throughout. The cultures were incubated at 30°C for 12 d, and examined for the highest heavy-metal concentrations, above which the tested organisms failed to grow MTC.
Effects of Fe2(SO4)3, Proline, and Heavy Metals on Crude-Oil Consumption by Halophilic/Halotolerant Isolates
In this experiment, the liquid mineral medium was provided with 2 M NaCl and used. Aliquots, 50 ml, dispensed in screw-caped flasks were provided with 0.3%, w/v, crude oil alone and together with the individual heavy-metal salts: HgCl2 (10 mg l-1), PbNO3 (10 mg l-1), CuSO4 (20 mg l-1), CdSO4 (10 mg l-1), and Na2HAsO4 (20 mg l-1). In addition, Fe2(SO4)3 (10 mg l-1) and proline (200 mg l-1) were amended, separately. Triplicates were prepared throughout. The media were inoculated with 1 ml portions of common inocula, sealed, and incubated at 30°C on a reciprocal shaker, 110 rpm, for 12 d. The residual oil in each flask was recovered with three 10 ml successive portions of pentane. The combined extract was raised to 35 ml using pure pentane, and 1 μl aliquots were analyzed by gas–liquid chromatography (GLC) using a Chrompack (Chrompack, Middelburg, Netherlands) CP-9000 instrument equipped with a FID, a WCOT-fused silica CP-SIL-5CB capillary column, and a temperature program, which raised the temperature from 45 to 310°C, 10°C min-1. The oil consumption was calculated as percent decreases of the total peak areas in the GLC profiles at the end of incubation based on the total peak areas of the abiotic controls (similarly treated, but using previously autoclaved inocula). Mean readings of the triplicates ± standard deviation values were calculated.
Effects of Heavy Metals on Growth of Halophilic/Halotolerant Isolates at Different Salinities
In this experiment, nutrient broth was used. Medium aliquots in test tubes were provided with NaCl at concentrations from 0.0 to 4.5 M. To study the effects of heavy metals on microbial growth, the medium aliquots were provided in addition with individual heavy-metal salts ranging in concentration from 0.0 to 80 mg l-1 of HgCl2 or PbNO3, and from 0.0 to 160 mg l-1 of CdSO4, CuSO4, or Na2HAsO4. Selection of those concentrations was based on results of preliminary experiments. Triplicates were prepared throughout. The test-tube cultures were inoculated with common inocula, and incubated at 30°C for 5 d. Growth was measured as optical density values at the commonly used wavelength of 660nm, using a spectrophotometer (Spectronic 21D, Milton Roy, United States). The use of oil-containing medium was avoided because the water-immiscible oil would have interfered with optical density measurement.
Effects of Salinity on Heavy-Metal Uptake by Halophilic/Halotolerant Isolates
The cells were first allowed to propagate in a heavy-metal-free medium, and were subsequently suspended in solutions of the metals whose uptake was to be measured.
Nutrient broth as a medium and the heavy-metal salts, HgCl2 and PbNO3, were used in this experiment. The oil-containing medium was not used because oil would interfere with heavy-metal uptake. Medium salinities were adjusted to 0, 1, 2, and 3 M NaCl. Heavy-metal-free medium aliquots were inoculated as described above, and incubated at 30°C for 24 h to allow for cell growth without heavy metals. Cells were harvested by centrifugation, 10,000 × g, for 10 min at 4°C and washed twice for a few minutes with sterile deionized water. The results of a preliminary experiment had confirmed that cells of H. salinarum (known to lyse in pure water) remained intact in deionized water for up to 3 h (duration of OD determination), but after 24 h complete cell lysis occurred. To recall, cell washing with water in this experiment took only minutes. The cells were subsequently suspended in 50 ml portions of deionized water containing 0, 1, 2, or 3 M NaCl, 0.2%, w/v, glucose and either HgCl2 (10 mg l-1) or PbNO3 (10 mg l-1). The suspensions were incubated for 40 min at 30°C. Longer incubation could have led to cell lysis after 3 h (at 0 M NaCl) as the preliminary experiments revealed. At time 0 and in 5 min intervals, 5 ml samples were harvested and centrifuged (10,000 × g) for 2 min at 4°C. Cells were digested with conc. HNO3 and HCl, 2:1 on a hot plate. The digestion solutions were filtered, diluted with 1% HNO3, and the heavy-metal-salt concentrations therein were determined by the USEPA method 6010B using Inductively Coupled Plasma Optical Emission Spectrometry (ICP-OES; PerkinElmer Optima 7300DV, United States) and Certified Reference Materials (multi-elements) (Oyetibo et al., 2010). As blank, the uninoculated liquid medium containing all the reagents was used. Reportedly, this method produces an average heavy-metal recovery of 100% with only 0.92% relative error (Han et al., 2006). The differences from the start concentrations were considered as the heavy-metal uptake values.
Statistical Analysis
As already mentioned, three parallel replicates for each analysis were prepared throughout, and the mean values ± standard deviation values were calculated using Microsoft Excel 2003. Statistical Package for Social Sciences, version 12 was used to assess the degree of significance, where the analysis of variance (ANOVA) was used to differentiate between the means of the tested parameters.
Results and Discussion
Numbers of Cultivable Heavy-Metal-Resistant CFU in Hypersaline Soil
To recall, the culture-dependent analysis captures the cultivable, hydrocarbonoclastic microorganisms only. Heavy metals are grouped into toxic and less toxic ones (Massadeh et al., 2005; Salgaonkar et al., 2013). This study focused on the first group that comprises mercury, cadmium, lead, and arsenic. For comparison, two representatives of the much less toxic heavy metals, copper and zinc, were used.
The results in Figure 1 show that the CFU numbers of hydrocarbonoclastic microorganisms (capable of growth on the mineral medium with oil vapor as a sole source of carbon and energy) as counted on the heavy-metal-free medium (control) ranged between about 170 ± 7 and 200 ± 11 CFU g-1. Addition of salts of mercury, cadmium, lead, and arsenic led to the lowest numbers of CFU counted. Increasing concentrations of those salts led to immediate decreases of the CFU numbers, until no more colonies appeared at heavy-metal-salt concentrations around 40 mg l-1. Basically, the same result was obtained with Na2HAsO4, although the maximum concentration of this heavy-metal salt above which no CFU appeared was >100 mg l-1. The results with CuSO4 and ZnSO4 were quite different. Within the concentration ranges of 0–50 and 80 mg l-1 of those salts, respectively, concentration increases were associated with immediate increases in the CFU numbers. Maximum numbers were counted in the presence of 50 mg l-1 CuSO4 (a concentration at which Hg2+, Cd2+, and Pb+ prevented CFU growth) and about 80 mg l-1 ZnSO4. Those enhancing effects are probably related to the roles of Cu2+ and Zn2+ in certain enzymatic activities (Salgaonkar et al., 2013). Furthermore, Cu2+ and Zn2+ with their low toxicity may be involved in cytoplasmic osmolarity regulation under high salinity, just like the conventional cations, Na+ and K+. Copper was more potent in its inhibitory effect than zinc, the maximum CuSO4 concentration above which no CFU appeared was 140 mg l-1, while as high as 300 mg l-1 ZnSO4 did not result in reducing the CFU numbers below those counted in the complete absence of this salt.
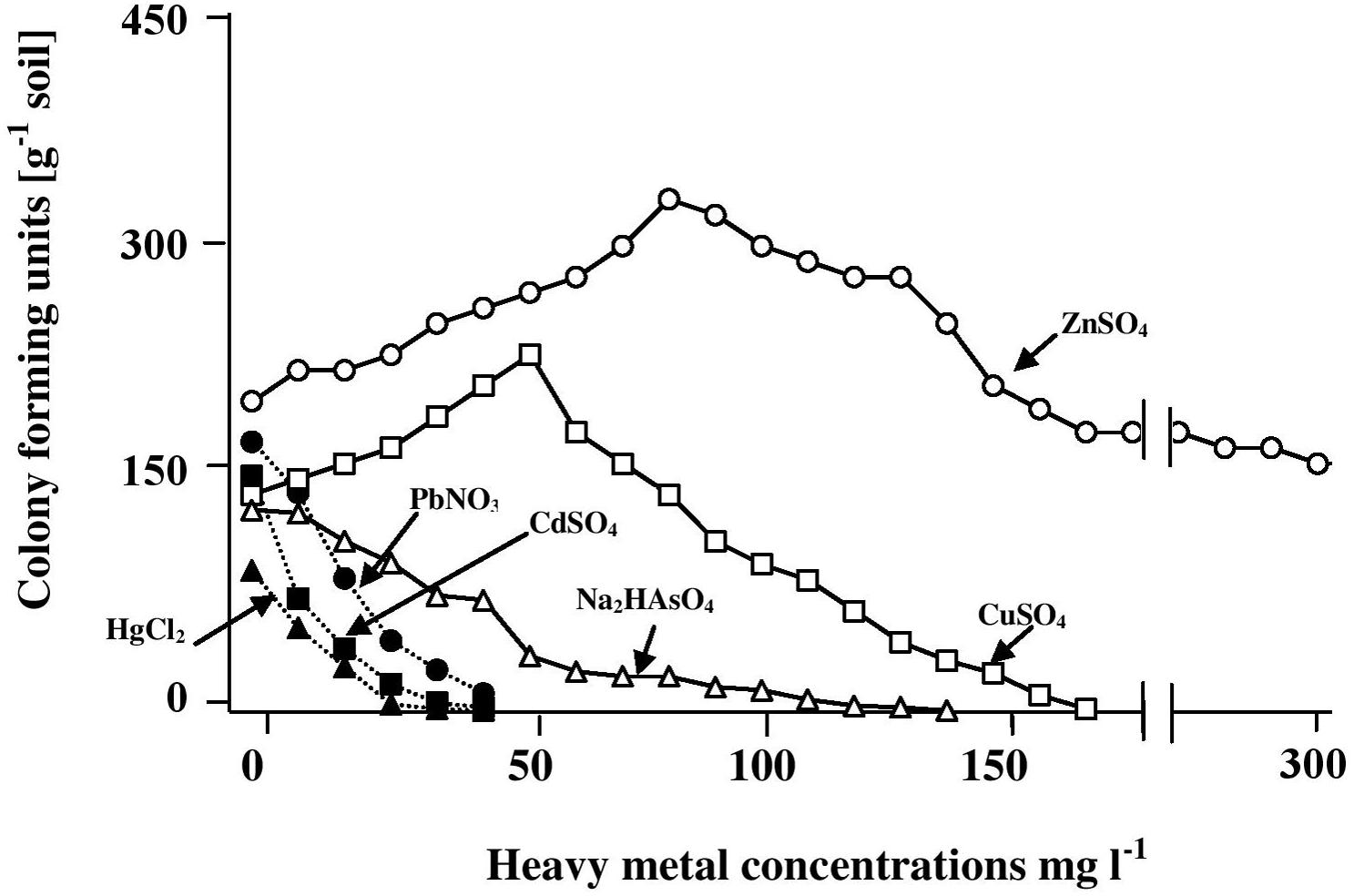
FIGURE 1. Numbers of CFU of hydrocarbonoclastic microorganisms in hypersaline soil as determined by the dilution-plating method on heavy-metal containing mineral medium with oil vapor as a sole source of carbon and energy. The medium salinity was adjusted to 2 M NaCl (section “Materials and Methods”). The CFU numbers are obviously those of cultivable microorganism only.
Pure Isolates of Hydrocarbonoclastic Microorganisms
The phylogenetic tree in Figure 2 shows the phylogenetic affiliations among the pure isolates studies and includes their accession numbers in the GenBank database.
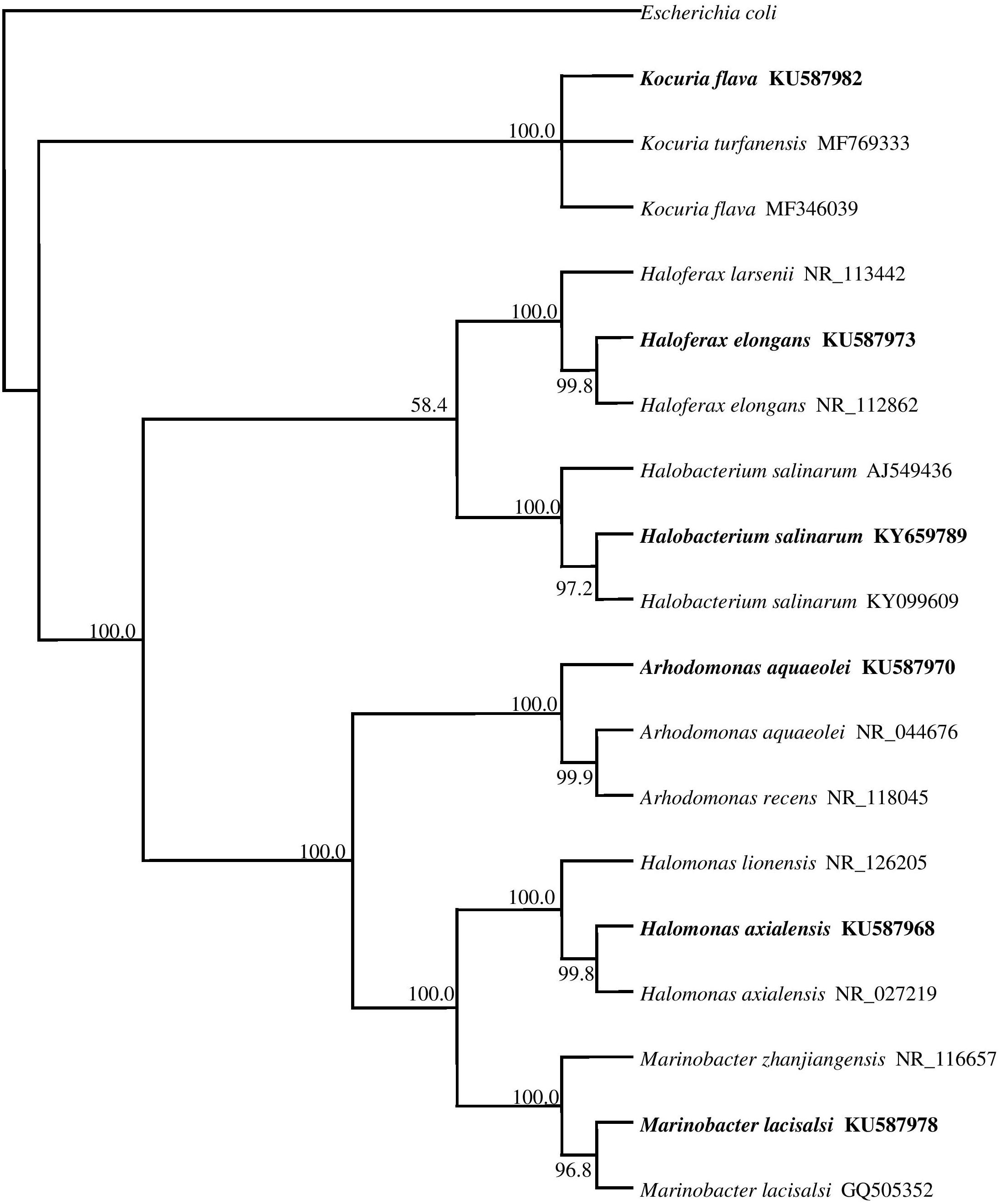
FIGURE 2. 16S rRNA gene phylogeny of hydrocarbonoclastic bacterial and archaeal isolates used in this study. Values used in each node of the tree are bootstrap values; 2000 bootstrap replicates were performed. The tree includes the accession numbers of the isolates.
Heavy-Metal Tolerance by Halophilic/Halotolerant Isolates on Oil-Free and Oil-Containing Media
The histograms in Figure 3 illustrate the dramatic inhibitory effects of crude oil on the heavy-metal tolerance by the four hydrocarbonoclastic bacterial and the two hydrocarbonoclastic haloarchaeal species from the hypersaline soil. Interestingly, the two haloarchaeal species seemed to be more sensitive to the tested heavy metals (showing weaker growth) than the four halophilic bacterial species, particularly on the crude-oil-free nutrient agar. Earlier researchers noted that numerous halophilic bacteria but only a few haloarchaea possess heavy-metal resistance (for review, see Voica et al., 2016). The highest tolerance measured in our study was that of A. aquaeolei which tolerated up to 1600 mg l-1 Na2HAsO4, and the lowest was that of M. lacisalci which tolerated up to only 200 mg l-1 HgCl2. High tolerance values were also measured for H. axialensis, which tolerated up to 1200 mg l-1 of CdSO4, CuSO4, and Na2HAsO4. K. flava tolerated up to 800 mg l-1 of CuSO4 and Na2HAsO4 and up to 600 mg l-1 of HgCl2, CdSO4, and PbNO3. In contrast, the highest tolerance of the haloarchaeon, H. elongans was at 200 mg l-1 Na2HAsO4, and that of H. salinarum was at 320 mg l-1 CdSO4. The heavy-metal-tolerance values on the oil-containing medium were lower than on the oil-free medium. A simple calculation showed that the highest concentrations of heavy metals tolerated by bacteria in the presence of oil were between only about 3 and 10% (in one case only reaching 13%) of the corresponding values in the absence of oil (on nutrient agar). Corresponding tolerance values for the archaeal species were considerably higher, between 20 and 25% (with 13% in three cases only).
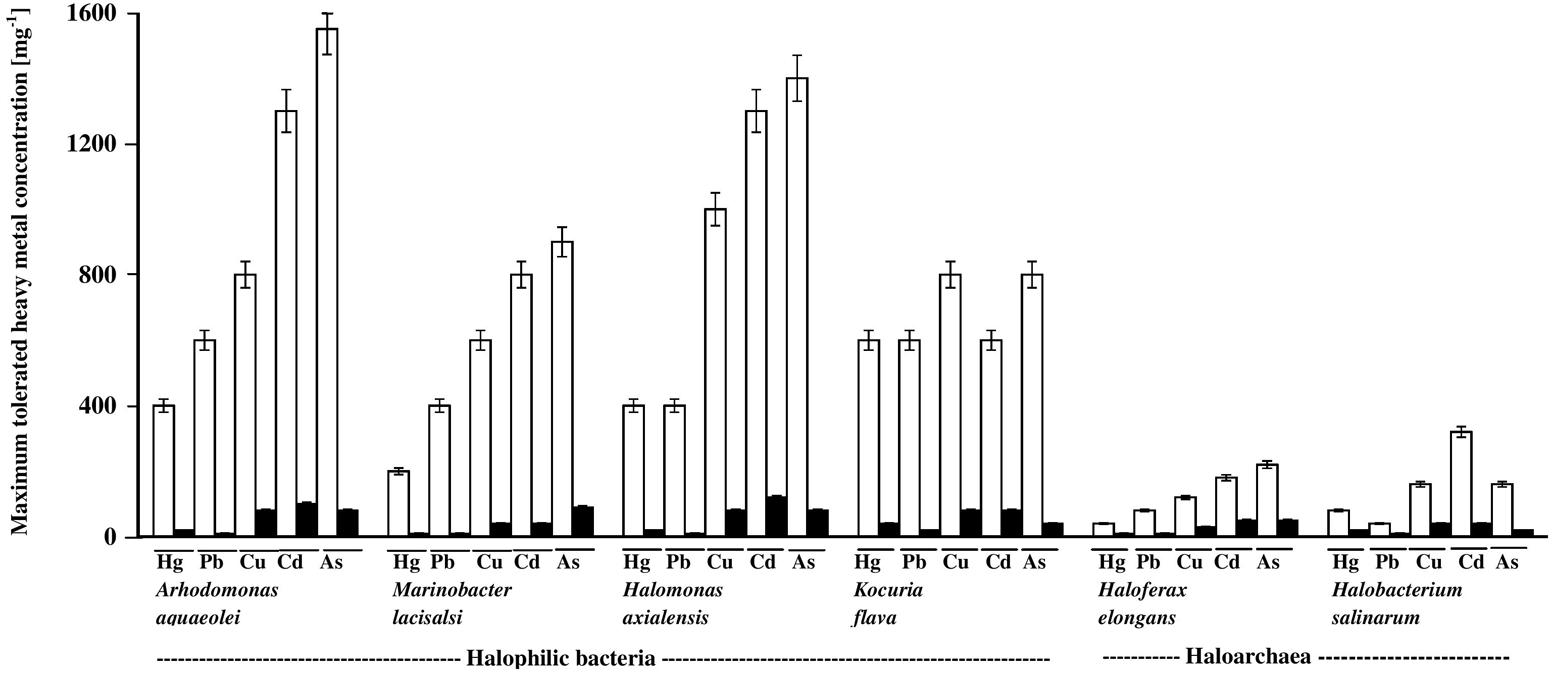
FIGURE 3. Heavy-metal tolerance by halophilic/halotolerant bacterial and archaeal cultures in the presence (black columns) and absence (white columns) of crude oil. Hg, HgCl2; Pb, PbNO3; Cu, CuSO4; Cd, CdSO4; As, Na2HAsO4. Each reading was the mean of three parallel replicates, whose maximum tolerated values were identical, i.e., no deviation. The medium salinity was adjusted to 2 M NaCl (section “Materials and Methods”).
There are no relevant result in the available literature to compare them with the above results. However, the weaker heavy-metal tolerance in the presence of oil than in its absence may be due to additional stress by aromatic hydrocarbons in the oil used (Shukla and Singh, 2012).
Effects of Fe2(SO4)3 and Proline on the “Maximum Heavy-Metal Concentrations” Tolerated by Halophilic/Halotolerant Isolates
The histograms in Figure 4 confirm that bacterial cultures commonly tolerated higher concentrations of heavy metals than archaeal cultures. The data also show that the tested microorganisms tolerated higher heavy-metal concentrations on the oil-free than in the oil-containing media. In many cases (highlighted by arrows in Figure 4), the addition of Fe3+ and, albeit to a less extent, proline enhanced this tolerance. The highest tolerated heavy-metal concentrations were double to triple as high as the concentrations tolerated in the absence of Fe2(SO4)3 and proline. This result was more frequent among the tested haloarchaea than among the halophilic bacteria. In several other cases, Fe2(SO4)3 and proline did not affect the heavy-metal tolerance at all. There are no relevant results in the available literature to compare them with those results. However, Fe3+ and proline might have contributed to osmoregulation of the cytoplasm leading to less salt stress and thus to enhanced heavy-metal tolerance. Why this did not happen in several other cases as Figure 4 shows, this could be due to the fact that concerned microorganisms accumulate other compatible solutes for osmoregulation.
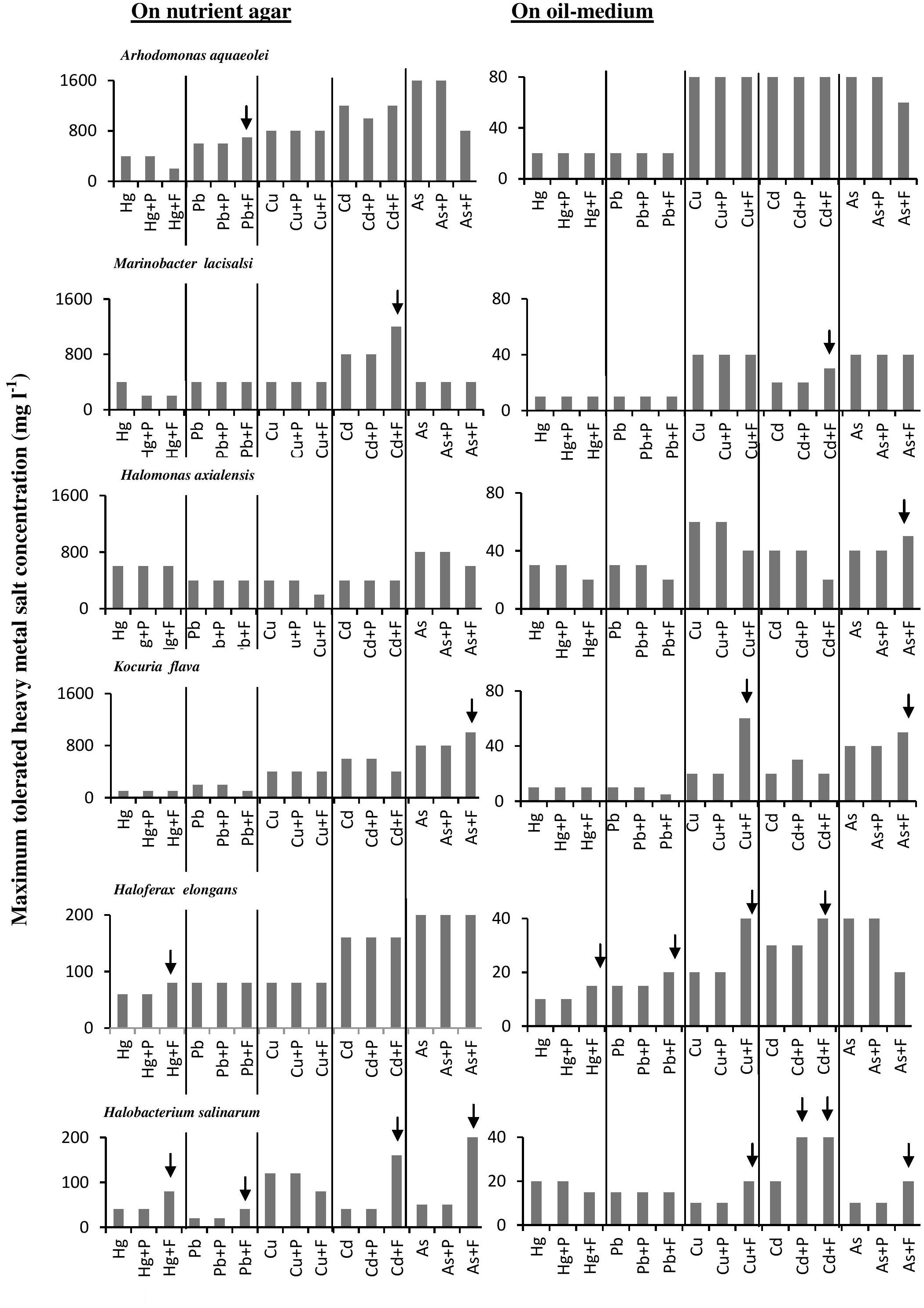
FIGURE 4. Effects of Fe3+ and proline on maximum-heavy-metal concentrations tolerated by halophilic/ halotolerant microorganisms. Arrows highlight positive effects of added Fe3+ and proline. Hg, HgCl2; Pb, PbNO3; Cu, CuSO4; Cd, CdSO4; As, Na2HAsO4. Each reading was the mean of three parallel replicates, whose maximum tolerated values were identical, i.e., there were no deviations. The medium salinity was adjusted to 2 M NaCl (section “Materials and Methods”).
Effects of Fe2(SO4)3 and Proline on Heavy-Metal-Mediated Inhibition of Crude-Oil Consumption by Halophilic/Halotolerant Isolates
In view of the extensive setup, this experiment was done on one bacterial and one haloarchaeal species only. The histograms in Figure 5 show that, in most of the cases, Fe3+ and proline significantly (P < 0.05) enhanced the oil consumption by the studied microorganisms in the presence of heavy metals. In many cases, the Fe3+-amendments led to consumption values much higher even than those in the absence of heavy metals. Still in some other cases, these amendments had even negative effects on oil consumption. Again the enhancement of oil consumption could have been due to the osmoregulatory effects of Fe3+ and proline, thus reducing the salt stress and supporting the heavy-metal tolerance of the respective oil-utilizing microorganisms. Furthermore, it has been reported that Fe3+ may serve as an electron acceptor during anaerobic respiration of bacteria and archaea (Lovley et al., 1991).
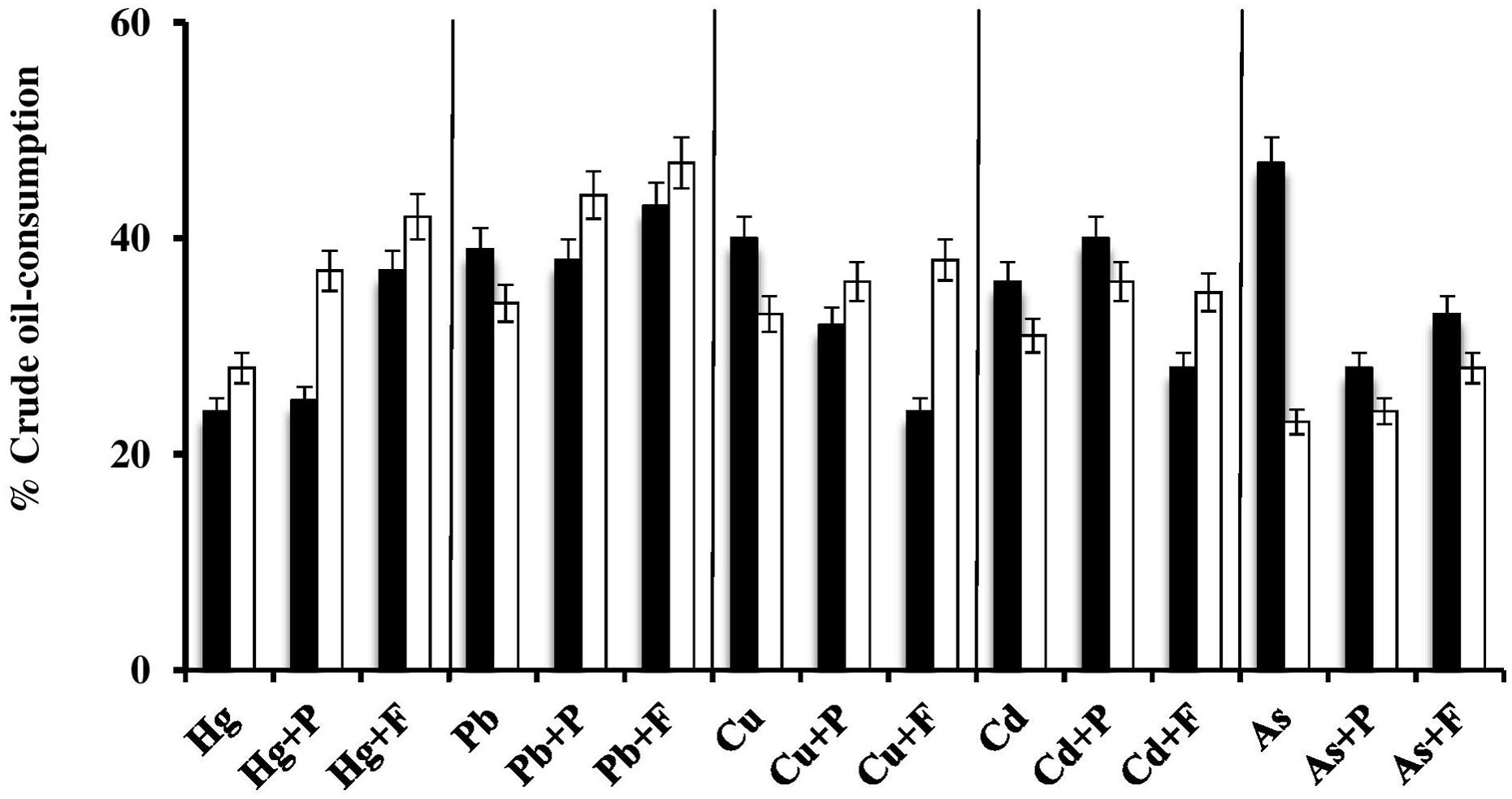
FIGURE 5. Effects of Fe3+ and proline on heavy-metal-mediated inhibition of crude oil consumption by the halophilic/halotolerant bacterium, Kocuria flava (black columns) and the haloarchaeon, Halobacterium salinarum (white columns). Hg, HgCl2; Pb, PbNO3; Cu, CuSO4; Cd, CdSO4; As, Na2HAsO4; P, proline; F, Fe2(SO4)3. The medium salinity was adjusted to 2 M NaCl (section “Materials and Methods”).
The typical GLC profiles in Figure 6 illustrate this novel result using the most toxic heavy-metal Hg2+. Irrespective of the actual mechanism(s) by which Fe3+ and proline may enhance the oil consumption by some microorganism, this result could obviously be of practical value in designing bioremediation biotechnologies for oil-contaminated, hypersaline environments.
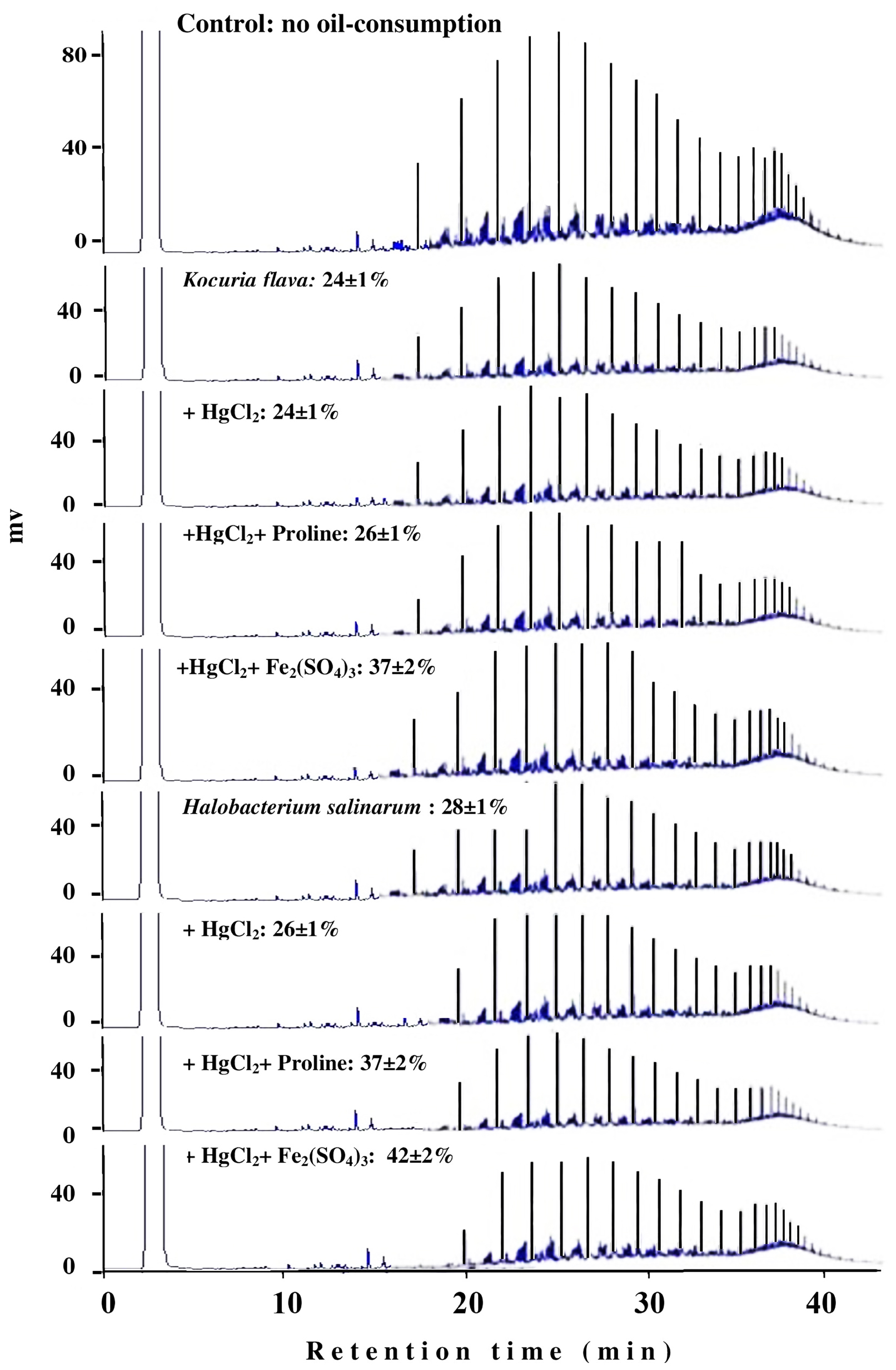
FIGURE 6. Typical GLC profiles of residual hydrocarbons in microbial cultures treated with Fe2(SO4)3 and proline. Percent values on the individual profiles are those of oil-consumption ± standard deviation. Note that small peaks mean more consumption, and that amendment with Fe2(SO4)3 and proline significantly enhanced the oil-consumption values by both organisms compared with the values in the presence of the heavy metals alone.
Effects of Heavy Metals on the Growth Magnitudes of Halophilic/Halotolerant Microorganisms Under Salt Stress
Figure 7 shows the effects of heavy metals on the growth magnitudes of tested isolates in nutrient broth containing various NaCl concentrations. The heavy metals did not only affect the growth magnitudes but also resulted in changing the optimum NaCl concentrations for growth of some isolates. As should be expected, the growth of the tested species was frequently best in the absence of heavy metals, and decreased with increasing heavy-metal concentrations. Exceptions, which are obvious in this figure, are quite interesting. Thus, with some organisms, HgCl2 and PbNO3 concentrations of 5 and 10 mg l-1 at certain salinities resulted in growth values similar to or higher than the values in the absence of those heavy metals. This was also true for CdSO4, CuSO4, and Na2HAsO4 at concentrations of 10 and 20 mg l-1. Higher heavy-metal-salt concentrations of up to 40 mg l-1 HgCl2 and up to 80 mg l-1 of all other heavy-metal salts did not arrest the growth of the tested organisms at most of the salinities. These results may imply that the “toxic” heavy-metal cations at low concentrations may be beneficial, probably by affecting cytoplasmic osmolality under environmental salt stress, like conventional cations do (K+, Mg2+, Ca2+, and Fe3+) (Al-Mailem et al., 2017). Furthermore, toxic heavy metals at trace concentrations were reported to serve as essential micronutrients in metabolic reactions and enzyme stabilization (Bruins et al., 2000).
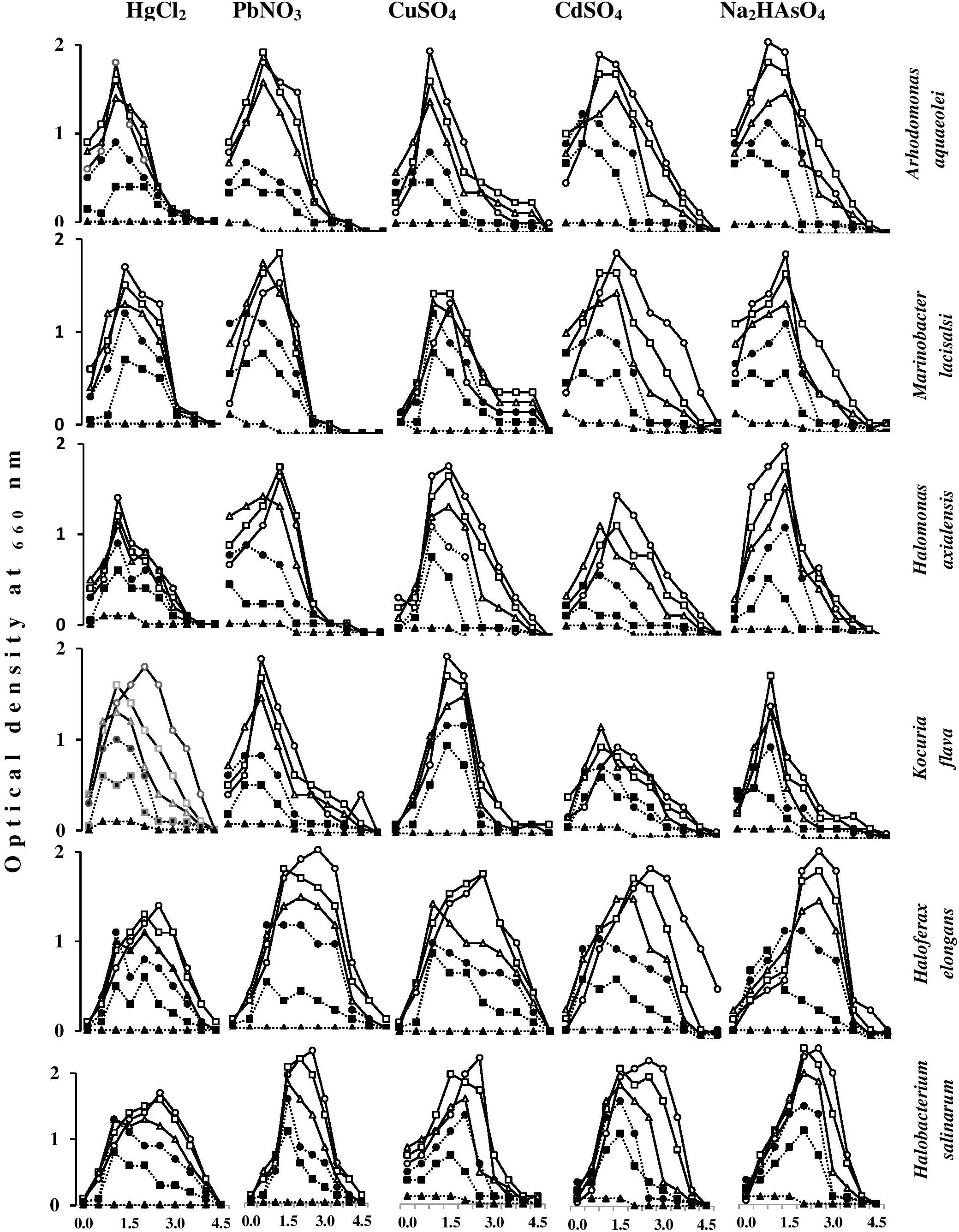
FIGURE 7. Effects of heavy-metal concentrations on the growth magnitudes of halophilic/halotolerant isolates at different salinities. HgCl2 and PbNO3 concentration (mg l-1): -o-, 0; -□-, 5; -Δ-, 10; -●-, 20; -■-, 40; -▲-, 80. CuSO4, CdSO4, Na2HAsO4 concentration (mg l-1): -o-, 0; -□-, 10; -Δ-, 20; -●-, 40; -■-, 80; -▲-, 160.
Heavy-Metal Uptake by Halophilic/Halotolerant Isolates at Various Salinities
It is important to recall that this experiment extended for only 40 min, and that it took haloarchaeal cells at least 3 h to start lysis in the absence of NaCl (see above). The results proved, however, that this short period was adequate to fulfill the objectives targeted.
Figure 8 shows the uptake rates of HgCl2 and PbNO3 by the six tested isolates. The uptake of both salts was almost completed during the first 5 min of incubation. Their cell-associated concentrations remained nearly constant or exhibited some decreases with time. The smallest proportions of HgCl2 and PbNO3 were taken up by the bacterium, H. axialensis and the haloarchaeon, H. salinarum in the absence of NaCl, whereas the highest uptake values by both microorganisms were in the hypersaline range (2 and 3 M NaCl). Conversely, the greatest amounts of HgCl2 were taken up in the absence of NaCl by A. aqueoleoi, M. lacisalsi, K. flava, and H. elongans; the latter species also took up the greatest amount of PbNO3 in the absence of NaCl. In the remaining cases, the highest concentrations of the two studied heavy-metal salts were taken up at 1 and 2 M NaCl, but 3 M NaCl inhibited the heavy-metal uptake. The fact that there was a direct relationship between salinity and heavy-metal uptake consolidates the assumption that heavy-metal cations may play some role in affecting cytoplasmic osmolality under salt stress. It may be argued that the heavy metals were just adsorbed on the cell envelops as it happens in many microorganisms as a part of the heavy-metal tolerance strategy (Nies, 1999; Deb et al., 2013). However, the tolerance of Hg2+, one of the two studied metals, is known to follow a completely different strategy which involves the reduction of the toxic Hg2+ to the less toxic volatile Hgo form (Nies, 1999; Wiatrowski et al., 2006; De et al., 2008). The patterns of HgCl2 uptake in Figure 8, however, are more similar to the active-transport pattern than to the pattern of metal loss by volatilization.
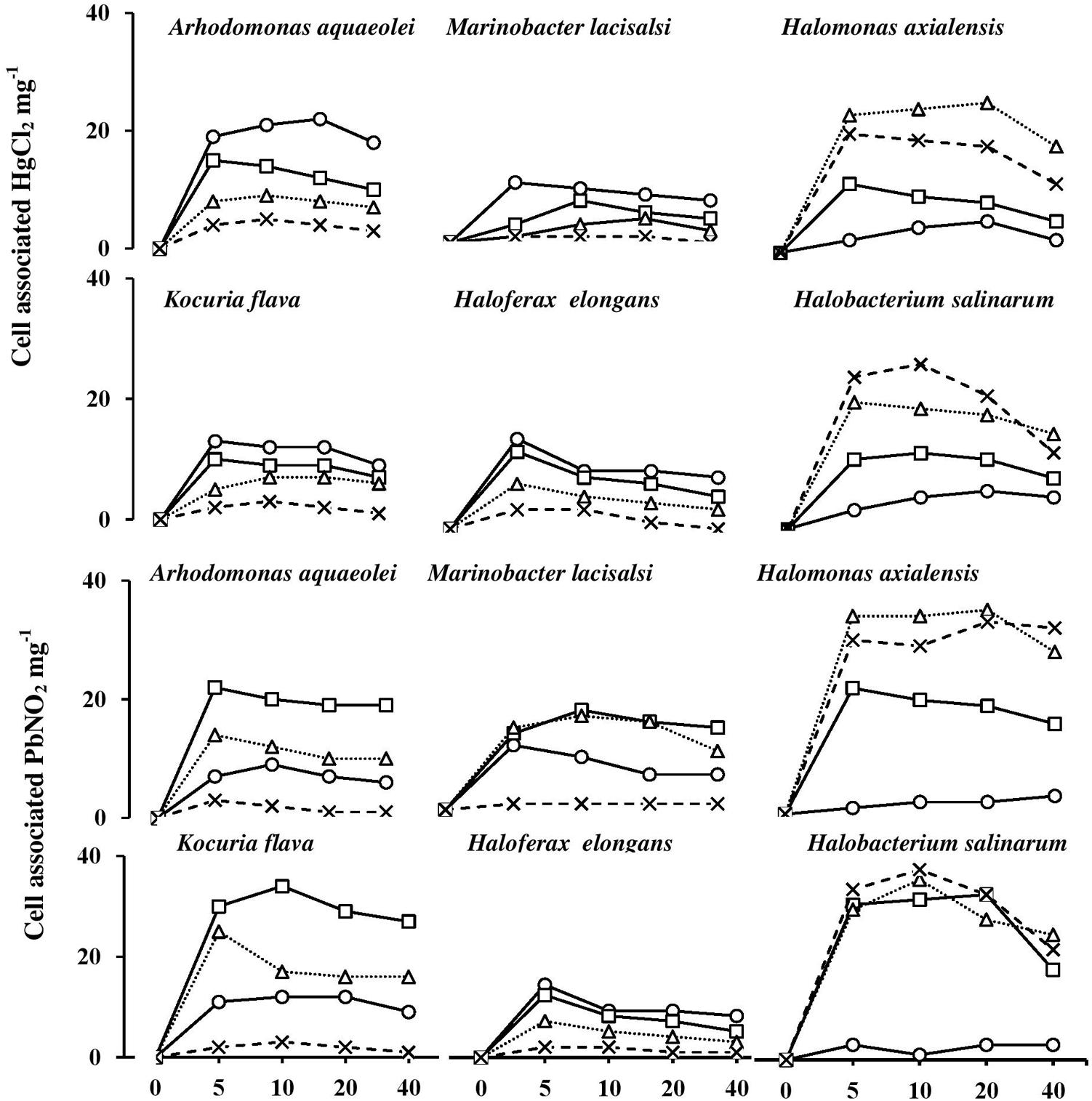
FIGURE 8. Heavy-metal uptake by halophilic microorganisms at various salinities. NaCl concentrations (M): circles, 0 M; squares, 1 M; triangles, 2 M; cross, 3 M.
Conclusion
The results of this study provide novel information complementary to our recent findings on biostimulation for oil bioremediation in salt-stressed environments. Although hydrocarbonoclastic bacteria and archaea indigenous to hypersaline soil occur permanently under multiple stresses, they still maintain the potential for active growth and biodegradation of spilled oil. In batch cultures, those organisms proved capable of growth and crude-oil removal in the presence of certain concentrations of heavy metals and high NaCl concentrations. For some isolates, those activities were significantly biostimulated by amended substances. Thus, Fe3+ and proline enhanced the tolerance of some organisms to toxic heavy metals, thus raising their potential for spilled-oil bioremediation under the prevailing multiple stresses. This study offers experimental evidence for quick uptake of heavy metals by tested microorganisms under salt stress. Although toxic, some metals enhanced salt tolerance and biodegradation of spilled oil in hypersaline environments.
Authors Contributions
DA-M, ME, and SR drafted the manuscript and performed the microbiological studies and genome sequencing analysis. DA-M and SR participated in the design of the study. All authors read and approved the final manuscript.
Funding
This work was supported by Kuwait University, Research Grant RS 01/12.
Conflict of Interest Statement
The authors declare that the research was conducted in the absence of any commercial or financial relationships that could be construed as a potential conflict of interest.
Acknowledgments
We acknowledge Kuwait University for funding (Research Grant RS 01/12). Thanks are due also to the RSPU unit, Kuwait University, for providing the Genetic Analyzer (GS 01/02) and to the NUERS unit, Kuwait University, for providing facilities for GLC and metal analysis (SRUL 01/13) and to Mrs. Majida Khanafer for technical help.
References
Al-Mailem, D. M., Al-Deieg, M., Eliyas, M., and Radwan, S. S. (2017). Biostimulation of indigenous microorganisms for bioremediation of oily hypersaline microcosms from the Arabian Gulf Kuwaiti coasts. J. Environ. Manage. 193, 576–583. doi: 10.1016/j.jenvman.2017.02.054
Al-Mailem, D. M., Eliyas, M., Khanafer, M., and Radwan, S. S. (2014a). Culture-dependent and culture-independent analysis of hydrocarbonoclastic microorganisms indigenous to hypersaline environments in Kuwait. Microb. Ecol. 67, 857–865. doi: 10.1007/s00248-014-0386-5
Al-Mailem, D. M., Eliyas, M., and Radwan, S. S. (2012). Enhanced haloarcaheal oil removal in hypersaline environments via organic nitrogen fertilization and illumination. Extremophiles 16, 751–758. doi: 10.1007/s00792-012-0471-y
Al-Mailem, D. M., Eliyas, M., and Radwan, S. S. (2014b). Enhanced bioremediation of oil-polluted, hypersaline, coastal areas in Kuwait via vitamin-fertilization. Environ. Sci. Pollut. Res. 21, 3386–3394. doi: 10.1007/s11356-013-2293-6
Banerjee, S. S., Joshi, M. V., and Jayaram, R. V. (2006). Treatment of oil spill by sorption technique using fatty acid grafted sawdust. Chemosphere 64, 1026–1031. doi: 10.1016/j.chemosphere.2006.01.065
Basumatary, B., Bordoloi, S., Sarma, H. P., and Das, H. C. (2012). A study on the physico-chemical properties and heavy metal content in crude oil contaminated soil of Duliajan, Assam, India. Int. J. Adv. Biotechnol. Res. 2, 64–66.
Bonfa, M. R. L., Grossman, M. J., Mellado, E., and Durrant, L. R. (2011). Biodegradation of aromatic hydrocarbons by haloarchaea and their use for the reduction of the chemical oxygen demand of hypersaline petroleum produced water. Chemosphere 84, 1671–1676. doi: 10.1016/j.chemosphere.2011.05.005
Bruins, M. R., Kapil, S., and Oehme, F. W. (2000). Microbial resistance to metals in the environment. Ecotoxicol. Environ. Saf. 45, 198–207. doi: 10.1006/eesa.1999.1860
Cui, H. L., Zhou, P. J., Oren, A., and Liu, S. J. (2009). Intraspecific polymorphism of 16S rRNA genes in two halophilic archaeal genera, Haloarcula and Halomicrobium. Extremophiles 13, 31–37. doi: 10.1007/s00792-008-0194-2
De, J., Ramaiah, N., and Vardanyan, L. (2008). Detoxification of toxic heavy metals by marine bacteria highly resistant to mercury. Mar. Biotechnol. 10, 471–477. doi: 10.1007/s10126-008-9083-z
Deb, S., Ahmed, S. F., and Basu, M. (2013). Metal accumulation in cell wall: a possible mechanism of cadmium resistance by Pseudomonas stutzeri. Bull. Environ. Contam. Toxicol. 90, 323–328. doi: 10.1007/s00128-012-0933-z
Emerson, D., Chauhanm, S., Oriel, P., and Breznak, J. A. (1994). Haloferax sp. D1227, a halophilic archaeon capable of growth on aromatic compounds. Arch. Microbiol. 61, 445–452. doi: 10.1007/BF00307764
Fathepure, B. Z. (2014). Recent studies in microbial degradation of petroleum hydrocarbons in hypersaline environments. Front. Microbiol. 5:173. doi: 10.3389/fmicb.2014.00173
Garcia, M. T., Mellado, E., Ostos, J. C., and Ventosa, A. (2004). Halomonas organivorans sp. nov., a moderate halophiles able to degrade aromatic compounds. Int. J. Syst. Evol. Microbiol. 54, 1723–1728. doi: 10.1099/ijs.0.63114-0
Han, F. X., Patterson, W. D., Xia, Y., Sridhar, B. M. M., and Su, Y. (2006). Rapid determination of mercury in plant and soil samples using inductively coupled plasma atomic emission spectroscopy, a comparative study. Water Air Soil Pollut. 170, 161–171. doi: 10.1007/s11270-006-3003-5
Hunter, J. R. (1982). “The physical oceanography of the Arabian Gulf. A review and theoretical interpretation of previous observations,” in The First Arabian Gulf Conference on Environment and Pollution, eds R. Halwagy, D. Clayton, and M. Behbehani (Kuwait City: Kuwait University), 1–23.
Lefebvre, O., and Moletta, R. (2006). Treatment of organic pollution in industrial saline wastewater: a literature review. Water Res. 40, 3671–3682. doi: 10.1016/j.watres.2006.08.027
Lovley, D. R., Phillips, E. J. P., Gorby, Y. A., and Landa, E. R. (1991). Microbial reduction of uranium. Nature 350, 413–416. doi: 10.1038/350413a0
Margesin, R., and Schinner, F. (2001). Biodegradation and bioremediation of hydrocarbons in extreme environments. Appl. Microbiol. Biotechnol. 56, 650–663. doi: 10.1007/s002530100701
Massadeh, A. M., Al-Momani, F. A., and Haddad, H. I. (2005). Removal of lead and cadmium by halophilic bacteria isolated from the Dead Sea Shore, Jordan. Biol. Trace Elem. Res. 108, 259–269. doi: 10.1385/BTER:108:1-3:259
Nicholson, C. A., and Fathepure, B. Z. (2005). Aerobic biodegradation of benzene and toluene under hypersaline conditions at the Great Salt Plains, Oklahoma. FEMS Microbiol. Lett. 245, 257–262. doi: 10.1016/j.femsle.2005.03.014
Nies, D. H. (1999). Microbial heavy-metal resistance. Appl. Microbiol. Biotechnol. 51, 730–750. doi: 10.1007/s002530051457
Oren, A. (2002). Diversity of halophilic microorganisms: environments, phylogeny, physiology, and applications. J. Indus. Microbiol. Biotechnol. 28, 56–63. doi: 10.1038/sj/jim/7000176
Oren, A., Gurevich, P., Azachi, M., and Hents, Y. (1992). Microbial degradation of pollutants at high salt concentrations. Biodegradation 3, 387–398. doi: 10.1007/BF00129095
Osuji, L. C., and Onojake, C. M. (2004). Trace heavy metals associated with crude oil: a case study of Ebocha-8 oil-spill-polluted site in Niger Delta, Nigeria. Chem. Biodivers. 1, 1708–1715. doi: 10.1002/cbdv.200490129
Oyetibo, G. O., Ilori, M. O., Adebusoyem, S. A., Oluwafemi, S., Obayori, O. S., and Amund, O. O. (2010). Bacteria with dual resistance to elevated concentrations of heavy metals and antibiotics in Nigerian contaminated systems. Environ. Monit. Assess. 168, 305–314. doi: 10.1007/s10661-009-1114-3
Pieper, D., and Reineke, W. (2000). Engineering bacteria for bioremediation. Curr. Opin. Biotechnol. 11, 262–270. doi: 10.1016/S0958-1669(00)00094-X
Radwan, S. S., Al-Mailem, D. M., and Kansour, M. K. (2017). Calcium (II)- and dipicolinic acid mediated-biostimulation of oil-bioremediation under multiple stresses by heat, oil and heavy metals. Sci. Rep. 7:9534. doi: 10.1038/s41598-017-10121-7
Salgaonkar, B. B., Mani, K., and Braganca, J. M. (2013). Characterization of polyhydroxyalkanoates accumulated by a moderately halophilic salt pan isolate Bacillus megaterium strain H16. J. Appl. Microbiol. 114, 1347–1356. doi: 10.1111/jam.12135
Santegoeds, C. M., Ferdelman, T. G., Muyzer, G., and Beer, D. (1998). Structural and functional dynamics of sulfate-reduction populations in bacterial biofilms. Appl. Environ. Microbiol. 64, 3731–3739.
Shukla, A., and Singh, C. S. (2012). “Hydrocarbon pollution: Effects on living organisms, remediation of contaminated environments, and effects of heavy metals co-contamination on bioremediation,” in Introduction to Enhanced oil Recovery (EOR) Processes and Bioremediation of Oil Contaminated Sites, ed. L. Romero-Zerón (Rijeka: In Tech).
Sorkhoh, N. A., Ghannoum, M. A., Ibrahim, A. S., Stretton, R. J., and Radwan, S. S. (1990). Crude oil and hydrocarbon-degrading strains of Rhodococcus rhodochrous isolated from soil and marine environments in Kuwait. Environ. Pollut. 65, 1–17. doi: 10.1016/0269-7491(90)90162-6
Swofford, D. L. (1998). PAUP∗. Phylogenetic Analysis Using Parasimony (∗ and Other Methods), Version 4. Sunderland, MA: Sinauer Associates.
Voica, D. M., Bartha, L., Banciu, H. L., and Oren, A. (2016). Heavy metal resistance in halophilic bacteria and Archaea. FEMS Microbiol. Lett. 363:fnw146. doi: 10.1093/femsle/fnw146
Wiatrowski, H. A., Ward, P. M., and Barkey, T. (2006). Novel reduction of mercury (II) by mercury-sensitive dissimilatory metal reducing bacteria. Environ. Sci. Technol. 40, 6690–6696. doi: 10.1021/es061046g
Keywords: bioremediation, haloarchaea, halophilic bacteria, heavy metals, hypersaline, environments, oil bioremediation
Citation: Al-Mailem DM, Eliyas M and Radwan SS (2018) Ferric Sulfate and Proline Enhance Heavy-Metal Tolerance of Halophilic/Halotolerant Soil Microorganisms and Their Bioremediation Potential for Spilled-Oil Under Multiple Stresses. Front. Microbiol. 9:394. doi: 10.3389/fmicb.2018.00394
Received: 11 January 2018; Accepted: 21 February 2018;
Published: 07 March 2018.
Edited by:
Hongchen Jiang, China University of Geosciences, ChinaReviewed by:
M. Oves, King Abdulaziz University, Saudi ArabiaKaren Trchounian, Yerevan State University, Armenia
Copyright © 2018 Al-Mailem, Eliyas and Radwan. This is an open-access article distributed under the terms of the Creative Commons Attribution License (CC BY). The use, distribution or reproduction in other forums is permitted, provided the original author(s) and the copyright owner are credited and that the original publication in this journal is cited, in accordance with accepted academic practice. No use, distribution or reproduction is permitted which does not comply with these terms.
*Correspondence: Samir S. Radwan, samir.radwan@ku.edu.kw