- 1Department of Biosciences, College of Life and Environmental Sciences, University of Exeter, Exeter, United Kingdom
- 2ISCA Diagnostics Ltd., Exeter, United Kingdom
Invasive pulmonary aspergillosis (IPA) is a life-threatening lung disease of immuno-compromised humans caused by the ubiquitous environmental mold Aspergillus. Biomarker tests for the disease lack sensitivity and specificity, and culture of the fungus from invasive lung biopsy is slow, insensitive, and undesirable in critically ill patients. A computed tomogram (CT) of the chest offers a simple non-intrusive diagnostic procedure for rapid decision making, and so is used in many hematology units to drive antifungal treatment. However, radiological indicators that raise the suspicion of IPA are either transient signs in the early stages of the disease or not specific for Aspergillus infection, with other angio-invasive molds or bacterial pathogens producing comparable radiological manifestations in a chest CT. Improvements to the specificity of radiographic imaging of IPA have been attempted by coupling CT and positron emission tomography (PET) with [18F]fluorodeoxyglucose ([18F]FDG), a marker of metabolic activity well suited to cancer imaging, but with limited use in invasive fungal disease diagnostics due to its inability to differentiate between infectious etiologies, cancer, and inflammation. Bioluminescence imaging using single genetically modified strains of Aspergillus fumigatus has enabled in vivo monitoring of IPA in animal models of disease. For in vivo detection of Aspergillus lung infections in humans, radiolabeled Aspergillus-specific monoclonal antibodies, and iron siderophores, hold enormous potential for clinical diagnosis. This review examines the different experimental technologies used to image IPA, and recent advances in state-of-the-art molecular imaging of IPA using antibody-guided PET/magnetic resonance imaging (immunoPET/MRI).
Introduction
Invasive pulmonary aspergillosis is a frequently fatal lung disease of immuno-compromised individuals caused by inhalation of spores of the air-borne fungus Aspergillus. While a number of species of Aspergillus can cause IPA, the principal agent of the disease is Aspergillus fumigatus, responsible for >80% of recorded cases. As an opportunistic pathogen, it causes more than 200,000 life-threatening infections in humans every year, mainly in high-risk patient groups such as those with hematological malignancies, and in hematopoietic stem cell (HSC) and solid organ transplant recipients, with mortality rates of between 30 and 90% (Segal, 2009). The disease is typically seen during periods of prolonged neutropenia, but non-neutropenic patients with underlying lung diseases such as steroid-treated chronic obstructive pulmonary disease (COPD), asthma, lung cancer, or autoimmune diseases with pulmonary involvement can develop IPA (Prattes et al., 2014). Furthermore, other Aspergillus lung diseases such as chronic pulmonary aspergillosis (CPA) and allergic bronchopulmonary aspergillosis (ABPA) are thought to affect >5 million people globally each year (Brown et al., 2012). Taken together, Aspergillus diseases represent a significant burden to human health, contributing to patient morbidities and prolonging hospitalization. Much of this burden is caused by the lack of diagnostic tests with sufficient accuracy to allow early identification and timely intervention with effective antifungal drugs.
Early detection of IPA and treatment with mold-active drugs is vital for patient survival. However, clinical symptoms of the disease (fevers and chills, hemoptysis, shortness of breath, chest pains, and headaches) are not specific for Aspergillus infections. The gold standard test for IPA is culture of Aspergillus from a sterile biopsy, but this is limited by poor sensitivity, lengthy turnaround time, and requires invasive recovery of lung tissue. Assays that detect circulating biomarkers of infection such as the Platelia galactomannan enzyme-linked immunosorbent assay (ELISA) and “pan-fungal” β-D-glucan tests lack either sensitivity or specificity (Prattes et al., 2014). The Aspergillus lateral-flow assay (LFA; Thornton, 2008) will be available commercially as a CE-marked in vitro device (IVD) for IPA diagnosis in March 2018. When used with BAL samples, it has the ability to be used as a point-of-care test, and so has the potential to improve the speed and accuracy of disease detection (Hoenigl et al., 2017). Despite this, the current inadequacies of IPA diagnostics have led to the empiric or “fever-driven” use of antifungals. This contributes to the erroneous treatment of already sick patients with costly and noxious drugs and promotes the emergence in Aspergillus of resistance to mold-active triazoles and to breakthrough infections. Empiric antifungal treatments also impact the sensitivities of fungal culture and biomarker-assisted tests, which are needed for diagnosis, for establishing drug sensitivities, and for monitoring responsiveness to treatments.
Diagnostic-driven approaches to antifungal treatment have been shown to be more effective than empiric treatment with respect to both cost and patient outcome (Barnes, 2013). Diagnostic-driven approaches to IPA treatment habitually rely on radiographic imaging, coupled with frequent testing for fungal biomarkers. Radiographic imaging is an attractive means of detecting Aspergillus lung infections in vivo because it is a non-invasive procedure, but basic radiographic findings in IPA are largely non-specific (Panse et al., 2016). A computed tomogram (CT) of the chest provides a quick non-intrusive clue for rapid decision making (Prasad et al., 2016), with the earliest sign suggestive of the disease being a nodule. The “halo sign,” a transient CT finding, is also suggestive of probable disease, and initiation of antifungal treatment in patients with this indicator at baseline has been associated with improved patient outcomes for early stages compared to later stages of disease (Greene, 2005; Greene et al., 2007). However, other mold pathogens such as mucormycetes species, and angio-invasive bacterial pathogens such as Pseudomonas aeruginosa, can give similar appearances (Segal, 2009; Stanzani et al., 2015). The “halo sign” therefore provides only limited accuracy for the diagnosis of IPA.
Over recent years, alternative techniques for non-invasive imaging of IPA have been developed and tested in pre-clinical animal models of disease (Velde and Wiehr, 2017). One such method is bioluminescence, that has been used to track Candida albicans and A. fumigatus infections and to monitor their responsiveness to antifungal treatments (Doyle et al., 2006; d’Enfert et al., 2010; Brock, 2012; Jacobsen et al., 2014). Bioluminescent strains of A. fumigatus have been generated through constitutive expression of the firefly luciferase gene under the fungal promoter gpdA (Brock et al., 2008). Transformed strains of the pathogen have been used to monitor antifungal drug efficacies in vitro and in vivo (Brock et al., 2008; Galiger et al., 2013) and to investigate the roles of resident and recruited immune effector cells in defense against invasive A. fumigatus infections (Ibrahim-Granet et al., 2010). The limitation of this technique is the requirement for genetically modified strains, which restricts studies to single mutants of the pathogen expressing luciferase. Different approaches for imaging IPA have therefore been explored using, for example, small molecules such as peptides (Yang et al., 2009), and the antifungal drug fluconazole coupled to 18F or 99mTc (Lupetti et al., 2002), for scintigraphic imaging of infections. For instance, using a 111In-labeled peptide c(CGGRLGPFC)-NH2 selected from a bacteriophage phage library, γ-imaging is able to delineate experimental IPA in mice (Yang et al., 2009). However, because the peptide corresponds to extracellular matrix proteins of the lung parenchyma, it is probable that the peptide binds to other fungi that are able to interact with extracellular matrix components of the lungs. Further specificity tests would therefore need to be conducted in vivo to determine the spectrum of IFDs detectable with this probe. While 99mTc-fluconazole proved to be superior to 18F-fluconazole for imaging of C. albicans infections in mice, it was found to be unsuitable for imaging of A. fumigatus infections (Lupetti et al., 2002).
The limitations of bioluminescence and small molecule imaging have led to efforts to improve the specificity of radiographic imaging of IPA by combining well-established hospital imaging technologies [high-resolution computed tomography (HRCT), MRI, and PET] with specific markers of infection. The aim of this mini-review is to examine recent advances in molecular imaging of IPA using radiolabeled Aspergillus-specific monoclonal antibodies (mAbs), and iron siderophores, and their potential for translation to the clinical setting.
Aspergillosis Imaging With Computed Tomography and Magnetic Resonance Imaging
According to current EORTC/MSG guidelines (De Pauw et al., 2008), a CT examination of the chest that reveals “dense, well-delineated, nodular infiltrates in the lung with or without ground glass attenuation (‘halo sign’) in a patient with an ongoing or recent history of prolonged neutropenia, or hematopoietic stem cell transplant (HSCT),” is defined as having a “possible” mold infection. Importantly, while 88–96% of neutropenic patients exhibit this sign in the first day of IPA, it disappears in one-third of patients within 72 h and in the remaining two-thirds of patients within 2 weeks (Caillot et al., 1997; Brodoefel et al., 2006). Furthermore, this diagnosis is not specific for IPA, as other infections, and neoplastic and inflammatory processes, can produce similar opacities with ground glass attenuation (Georgiadou et al., 2011), and the disease can also manifest as atypical presentations in liver transplant patients (Mucha et al., 2013) and during invasive bronchial-pulmonary aspergillosis in critically ill patients with COPD (Huang et al., 2016). Radiological indications are rare in the initial stages of IPA in non-neutropenic patients (Prattes et al., 2014), and differ between children and adults with IFDs (Ankrah et al., 2016).
Notwithstanding these limitations, CT acts as a prompt for instigating antifungal treatment in numerous hematology units, with HRCT providing opportunities for improved antifungal stewardship (Stanzani et al., 2016). Reductions in the unnecessary use of antifungal drugs have been reported in centers using HRCT to drive treatment strategies in allogeneic transplant patients who have persistent febrile antibiotic-resistant neutropenia (Dignan et al., 2009). Therefore, in certain settings, refractory fever/HRCT-based approaches to diagnosis may result in significant reductions in parenteral antifungal drug usage in patients who would otherwise have received empirical treatment. However, due to the limited specificity of HRCT, such an approach cannot be used for IPA specifically, but rather IFDs as a whole (Dignan et al., 2009). Improvements to the specificity of HRCT for the detection of fungal lung infections have been attempted by combining it with pulmonary angiography (CTPA), but the performance of CTPA relative to other signs (halo sign, hypo-dense sign, pleural effusion, and reversed halo sign) is not known. Nevertheless, vessel occlusion detected by CTPA may be a more sensitive and possibly more specific radiographic sign in patients with hematological malignancies (Stanzani et al., 2015).
Pre-clinical studies using MORF oligomers that target fungal ribosomal RNA have shown that CT specificity can be dramatically improved when 99mTc-labeled Aspergillus-specific probes are combined with SPECT (Wang et al., 2013). Two probes, AGEN and AFUM, have been investigated that are genus-specific and A. fumigatus-specific, respectively. Single photon emission tomography (SPECT)/CT imaging of mice with experimental IPA showed a twofold increased accumulation of both 99mTc-labeled probes in A. fumigatus infected lungs compared to uninfected controls. While the AGEN oligomer was found to cross-react with C. albicans, and the AFUM oligomer would preclude detection of infectious Aspergillus species other than A. fumigatus, the work nevertheless elegantly demonstrates that CT imaging can be rendered disease-specific by using pathogen-specific probes.
Magnetic resonance imaging is now the non-invasive imaging tool of choice, with high spatial resolution and the highest soft tissue contrast. However, unlike HRCT, MRI has received far less attention as a diagnostic imaging modality for Aspergillus lung infections, but has been studied extensively as a detection aid for Aspergillus cerebral and central nervous system infections (Starkey et al., 2014; Marzolf et al., 2016). While CT is highly suitable for lung applications because it produces high-resolution 3D images with an excellent air–tissue contrast, MRI of the lung is challenging owing to the lack of detectable protons in air-filled spaces and potential artifacts between air–tissue interfaces. Despite these shortcomings, a longitudinal in vivo study in mice showed that A. fumigatus lung lesions could be visualized and quantified using MRI (Poelmans et al., 2016). By using an advanced MR pulse sequence with ultra-short echo times, pathological changes within the infected lung were visually and quantitatively detectable and with a high degree of sensitivity. In humans, dynamic contrast-enhanced MRI (DCE-MRI) might also be useful for imaging IPA in immunosuppressed acute myeloid leukemia patients (Araz et al., 2014).
Aspergillosis Imaging With [18F]FDG Positron Emission Tomography
In contrast to HRCT and MRI, PET can give information about the physiological status of the particular target organ. It has emerged as an immensely powerful imaging technique in the field of oncology, but its use in infectious disease imaging is very much in its infancy (Glaudemans et al., 2012, 2015; Signore et al., 2015). Compared to SPECT of IPA using 67Ga scintigraphy (Tzen et al., 1999; Goméz et al., 2000), PET provides increased sensitivity and resolution through coincidence detection of photons emitted from radionuclei resulting from positron annihilation, with its success in oncology resulting from the use of [18F]fluorodeoxyglucose ([18F]FDG), a diagnostic tracer that specifically accumulates in metabolically active inflammatory cells (neutrophils, macrophages, and lymphocytes), cancer cells, and during infectious processes (Imperiale et al., 2010; Bassetti et al., 2017).
Several studies have indicated that [18F]FDG might be useful for imaging fungal infections (Sharma et al., 2014), for differentiating between non-invasive and invasive aspergillosis (Kim et al., 2013), for identification of extra-pulmonary sites of infection (Chamilos et al., 2008), and for therapy monitoring (Ho et al., 1998; Ozsahin et al., 1998; Franzius et al., 2001; Stanzani et al., 2016). However, a recent study by Rolle et al. (2016), which employed PET and MRI (PET/MRI) to detect lung infections by A. fumigatus in vivo, showed that the increase in [18F]FDG uptake during Aspergillus lung infection could not be distinguished from that seen during sterile inflammation or during bacterial lung infections caused by Streptococcus pneumonia or Yersinia enterocolitica, further demonstrating the lack of specificity of this tracer which has been reported elsewhere (Petrik et al., 2014). While these studies are pre-clinical investigations using mouse models of IPA, numerous clinical studies have also reported the lack of specificity of FDG-PET for diagnosing the disease in humans, with Aspergillus lung diseases mimicking lung cancer (Wilkinson et al., 2003; Baxter et al., 2011; Garcia-Olivé et al., 2011), metastatic thyroid cancer (Ahn et al., 2011), lymphoma (Sonet et al., 2007), and tuberculoma (Nishikawa et al., 2011). Pulmonary IFIs caused by fungi other than Aspergillus (e.g., Candida, Blastomyces, Cryptococcus, Coccidioides, Histoplasma, mucormycetes, and Pneumocystis) are also detected by FDG-PET in humans (Ankrah et al., 2016). Consequently, while FDG-PET may confirm IPA lesions observed using HRCT and other imaging modalities (Chamilos et al., 2008; Hot et al., 2011), and to guide needle aspirations of lung tissues for fungal culture (Casal et al., 2009), it cannot be used for definitive in vivo diagnosis of IPA, or for its differentiation from ABPA (Nakajima et al., 2009) or aspergillomas (Franzius et al., 2001; Ahn et al., 2011).
Aspergillosis Imaging With 68Ga-Siderophores
Other than the Aspergillus-reactive 99mTc-labeled MORF probes (Wang et al., 2013), few imaging tracers have been developed that specifically target Aspergillus infections, but substantial success has been achieved by combining microPET/CT with iron-scavenging siderophores labeled with 68Ga or 89Zr. Iron is essential for fungal growth and, in iron-poor environments such as serum, bacteria and fungi produce low molecular weight ferric iron-specific chelators to scavenge iron from the host (Haas, 2003). A. fumigatus lacks specific uptake systems for host iron sequestered in heme, ferritin or transferrin, and instead uses two high-affinity iron uptake mechanisms, reductive iron assimilation and siderophore-assisted iron mobilization, both of which are induced under conditions of iron starvation. The pathogen produces three hydroxamate-type siderophores, extracellular fusarinine C (FSC) and triacetylfusarinine C (TAFC), and intracellular ferricrocin (FC; Schrettl et al., 2004; Haas et al., 2015). TAFC is secreted soon after spore germination in iron-limited media (Hissen et al., 2004), and is detectable in serum from patients with proven/probable IPA (Carroll et al., 2016). Its biosynthesis is an essential requirement for spore germination, and for virulence in a mouse model of disease (Schrettl et al., 2004; Hissen et al., 2005). Using 68Ga, a positron emitter with complexing properties similar to those of Fe(III), Petrik et al. (2010) evaluated the potential of [68Ga]TAFC and [68Ga]FC as radiopharmaceuticals for imaging of IPA. They showed that uptake by A. fumigatus was highly dependent on iron load, but that [68Ga]TAFC displayed excellent in vitro stability, and highly selective accumulation in iron-starved cells. Uptake of [68Ga]TAFC in the lungs of immunosuppressed rats correlated with severity of Aspergillus infection, while the bio-distribution of [68Ga]FC was inferior to [68Ga]TAFC, and showed poor stability both in vitro and in vivo. [68Ga]TAFC was again shown in a subsequent pre-clinical study to selectively accumulate in infected lung tissues in a rat infection model, and that another siderophore ferrioxamine (FOXE), when coupled to 68Ga ([68Ga]FOXE), also displayed excellent pharmacokinetics, highly selective accumulation in Aspergillus infected lung tissues, and similarly good correlation with disease severity (Petrik et al., 2012a,b). A downside of using the radionuclide 68Ga in PET imaging, compared to longer-lived positron emitters such as 64Cu (t1/2 = 12.7 h), 124I, 86Y, 90Nb, or 89Zr (t1/2 = 78.41 h), is its relatively short half-life (t1/2 = 67.7 min), which limits its use in longitudinal studies. For this reason, alternative siderophores have been investigated for radiolabeling with 89Zr and for use as imaging agent for Aspergillus infections (Petrik et al., 2016). Small animal imaging studies of all 68Ga- and 89Zr-labeled siderophores injected in mice displayed similar pharmacokinetics and minimal accumulation of radioactivity in blood and other organs and tissues, with the exception of [89Zr]FOXE which caused significant retention in the gastrointestinal tract. [89Zr]TAFC showed favorable properties for potential longitudinal Aspergillus infection imaging.
Using the radiolabeled siderophores as diagnostic tracers should allow for highly specific detection of IPA since TAFC and FC have no function in human physiology and uptake is not detectable in human lung cancer cells (Petrik et al., 2014). However, while the energy-dependent siderophore transporter system might appear advantageous compared to cell wall-labeling approaches using, for example, Aspergillus-specific mAbs, the use of radiolabeled siderophores does have its limitations. The first is specificity. While pathogenic bacteria have been shown not to use TAFC- or FOXE-mediated uptake of iron (Petrik et al., 2014), Fe-TAFC uptake under iron depletion has been demonstrated in the human pathogenic fungi C. albicans (Lesuisse et al., 2002) and Fusarium oxysporum (Leal et al., 2013), and [68Ga]TAFC and [68Ga]FOXE uptake under iron deficiency has been shown in the human pathogens Fusarium solani and Rhizopus oryzae (Petrik et al., 2014). Consequently, further work is needed to determine whether [68Ga]TAFC (or [68Ga]FOXE) uptake is able to discriminate between Aspergillus infections in vivo and commensal C. albicans colonization of the gastrointestinal tract, invasive candidiasis, mucormycosis, and disseminated Fusarium infections (fusariosis). Furthermore, while A. fumigatus is the principal agent of IPA, other Aspergillus species such as Aspergillus flavus, Aspergillus nidulans, Aspergillus niger, and Aspergillus terreus are able to cause the disease (Willinger et al., 2014). While uptake of Fe-TAFC has been shown in A. nidulans (Oberegger et al., 2001) and [68Ga]TAFC uptake has been shown in A. flavus and A. terreus (albeit at significantly lower levels than A. fumigatus), it is unclear whether all infectious Aspergillus species are detectable in vivo using this system. The second consideration is the important role of iron overload in the development of invasive fungal diseases. Many patients at high risk for developing IPA (heavily transfused AML patients, neutropenic patients, liver and allogeneic HSCT recipients, and those receiving chemotherapy) frequently have iron overload, which has been shown to contribute to the increased susceptibility of these groups to invasive fungal infections (Alexander et al., 2006; Bullen et al., 2006; Pagano et al., 2011). In these patients, freely available iron could arguably lead to decreased imaging sensitivity using [68Ga]TAFC (or [68Ga]FOXE), given the strong correlation between iron availability and uptake of radiolabeled siderophores by A. fumigatus. Despite these potential drawbacks, the possibility of accurately diagnosing IPA in humans using [68Ga]TAFC or [68Ga]FOXE imaging merits clinical evaluation.
Aspergillosis Imaging With Monoclonal Antibody JF5
Despite the abilities of mAbs to differentiate different genera and species of human pathogenic fungi, and their capacity to discriminate between inactive spores and invasive hyphae (Thornton and Wills, 2015), their use in molecular imaging of IFDs has yet to be fully realized. Imaging with antibodies has, until very recently, been the domain of cancer detection (Mestel, 2017), with limited application in infectious disease diagnostics (Rolle and Wiehr, 2017). However, recent studies have demonstrated the enormous potential of antibody-guided PET/MRI (immunoPET/MRI) technologies to dramatically improve the molecular imaging of viral (Santangelo et al., 2014), bacterial (Wiehr et al., 2016), and fungal (Rolle et al., 2016; Davies et al., 2017) infections in vivo, with the real possibility of precision medicine for infectious diseases in the near future (Jain, 2017). ImmunoPET/MRI marries functionality of PET with the specificity of mAbs and anatomical depiction of MRI. Any infectious disease can potentially be detected with this technology provided that high-integrity antibodies are available that have sufficient specificity for the target organism and which detect signature molecules of active infection. In the case of IPA, these diagnostic requirements have been met through the Aspergillus-specific mouse mAb mJF5 (Thornton, 2008) and its humanized derivative hJF5 (Davies et al., 2017). mAb mJF5, which forms the basis of the Aspergillus LFA (Thornton, 2008; Prattes et al., 2014; Hoenigl et al., 2017), binds to extracellular (galacto)mannoprotein antigens produced by all clinically relevant Aspergillus species (Thornton, 2014; Davies et al., 2017), and is able to detect IPA in humans caused by A. fumigatus, A. flavus, A. nidulans, A. niger, and A. terreus, either as single or as mixed species infections (Willinger et al., 2014). Its high-level specificity for Aspergillus species means that it is able to discriminate between IPA and lungs infections caused by other mold pathogens including F. solani (Willinger et al., 2014). Furthermore, the JF5 antigen is produced during active growth only, which means that it is able to differentiate between inactive spores present in inhaled air and invasive hyphae that infect or colonize the lung (Thornton, 2008, 2014).
Aspergillosis Imaging With Antibody-Guided Positron Emission Tomography/Magnetic Resonance Imaging
Novel probes for the non-invasive detection of A. fumigatus lung infection based on antibody-guided PET/MR imaging (immunoPET/MRI) have recently been reported (Rolle et al., 2016; Davies et al., 2017). Administration of [64Cu]DOTA-labeled mAb mJF5 to neutrophil-depleted A. fumigatus-infected mice allowed specific localization of lung infections when combined with PET, while optical imaging with a fluorophore (DyLight650)-labeled version of the mAb showed co-localization with invasive hyphae (Rolle et al., 2016). The [64Cu]DOTA-mJF5 tracer distinguished Aspergillus from bacterial lung infections and, unlike [18F]FDG-PET, differentiated Aspergillus infection from lung inflammation caused by a sterile inflammatory stimulus. The long in vivo half-life of [64Cu]DOTA-mJF5 allows repeat imaging of infections following a single injection of the radioactive tracer and, because it is hyphal-specific, may prove useful in monitoring infection in response to antifungal treatment.
Despite specific uptake of the [64Cu]DOTA-mJF5 tracer in the lungs of A. fumigatus-infected animals compared to uninfected controls, high liver uptake of the tracer was also evident. It was hypothesized that this liver uptake might be due to hepatic removal of the radiolabeled antibody circulating in the bloodstream, specific binding to antigen in liver tissues following shedding of soluble antigen from hyphae in the lungs, and transchelation of 64Cu to liver proteins due to insufficiently strong binding to the chelator DOTA. Transchelation of 64Cu to serum protein was shown not to occur, although it has been shown elsewhere that DOTA has a poor in vivo stability, which results in loss of the radio-metal and its non-specific accumulation in off-target tissues. In a subsequent study (Davies et al., 2017), the chelator DOTA was exchanged with the alternative 64Cu chelators DOTAGA and NODAGA, which have increased in vivo stability. This decreased the uptake of the radiolabeled immuno-conjugates in the liver, while preserving specific accumulation in the A. fumigatus-infected lung. In particular, a NODAGA conjugated tracer ([64Cu]NODAGA-mJF5) provided the lowest liver uptake, while enabling the greatest uptake in infected lungs.
The immunoPET/MR imaging technology based on mAb mJF5 is fundamentally translatable to human disease detection since the antibody tracks a biomarker of Aspergillus infection that has been clinically validated for human IPA diagnosis using LFA tests of serum and BAL fluids (Thornton, 2008; Held et al., 2013; White et al., 2013; Prattes et al., 2014; Willinger et al., 2014; Hoenigl et al., 2017). To allow translation of the antibody tracer to the clinical setting, a humanized version of the antibody (hJF5) has been generated by grafting of the mJF5 CDRs into a human IgG1 framework (Davies et al., 2017). Pre-clinical imaging with a [64Cu]NODAGA-hJF5 tracer in a neutropenic mouse model of IPA (Figure 1) has demonstrated improved PET/MR image resolution of A. fumigatus lung infections compared to its murine counterpart [64Cu]NODAGA-mJF5 (Davies et al., 2017), with the lowest liver uptake but highest uptake in infected lungs (Figure 2).
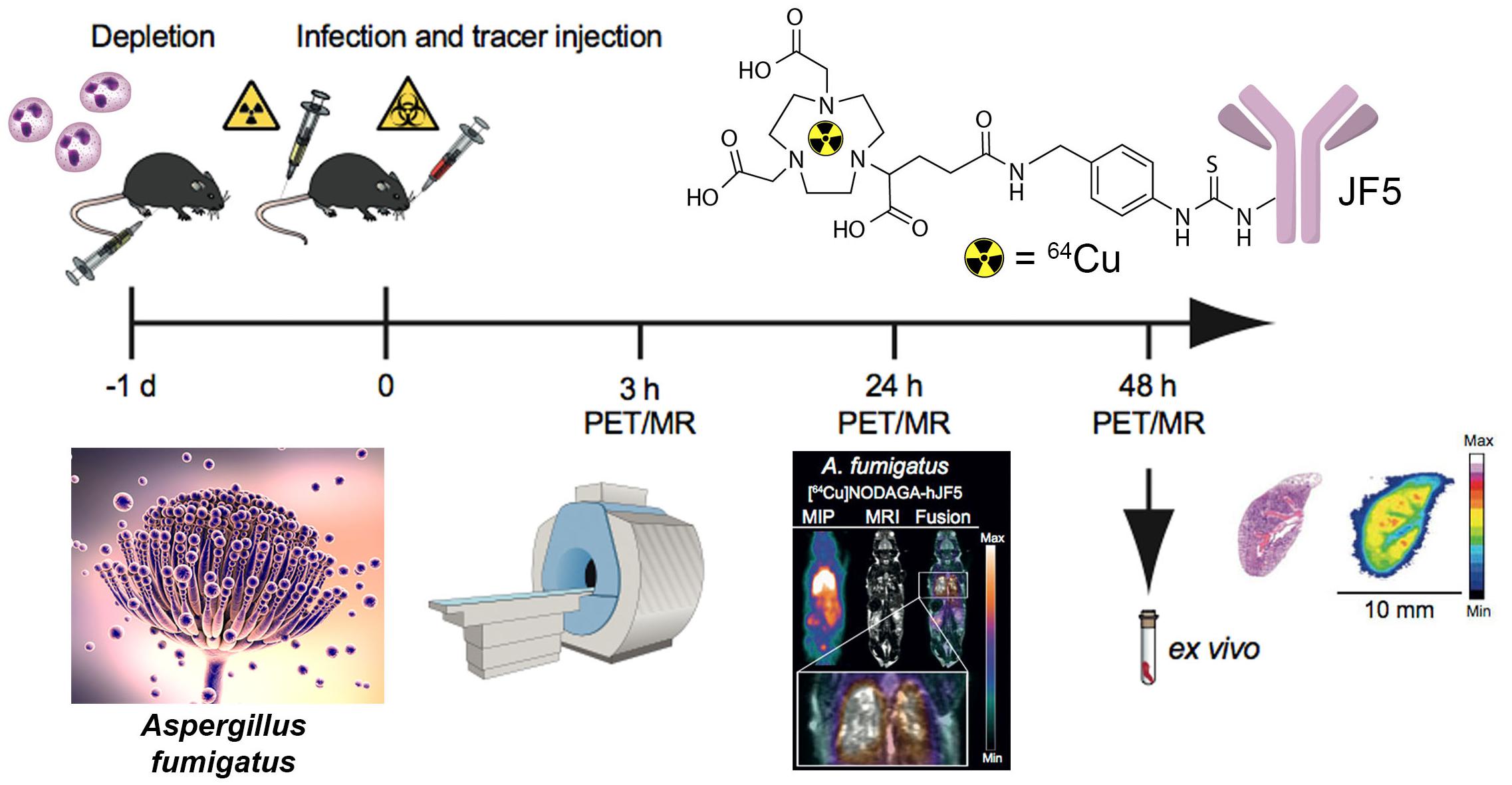
FIGURE 1. Schematic representation of antibody-guided immunoPET/MR imaging of IPA in a neutropenic mouse model of disease. Twenty-four hours (–1 day) prior to infection with A. fumigatus, and administration of the PET tracer, neutropenia is induced in mice by the injection of 100 μg of the anti-Ly-6G/anti-Ly6C antibody RB6-8C5. At time 0, mice are infected by intratracheal injection with an A. fumigatus spore suspension and with simultaneous tail vein injection with a JF5-based PET tracer. The tracer shown is mAb JF5 conjugated to the positron emitter 64Cu using the chelator NODAGA. Simultaneous PET/MR imaging of animals is then performed 3, 24, and 48 h after infection/tracer injection. Ex vivo bio-distribution and autoradiography are conducted after the last PET scan at 48 h. Adapted from Davies et al. (2017).
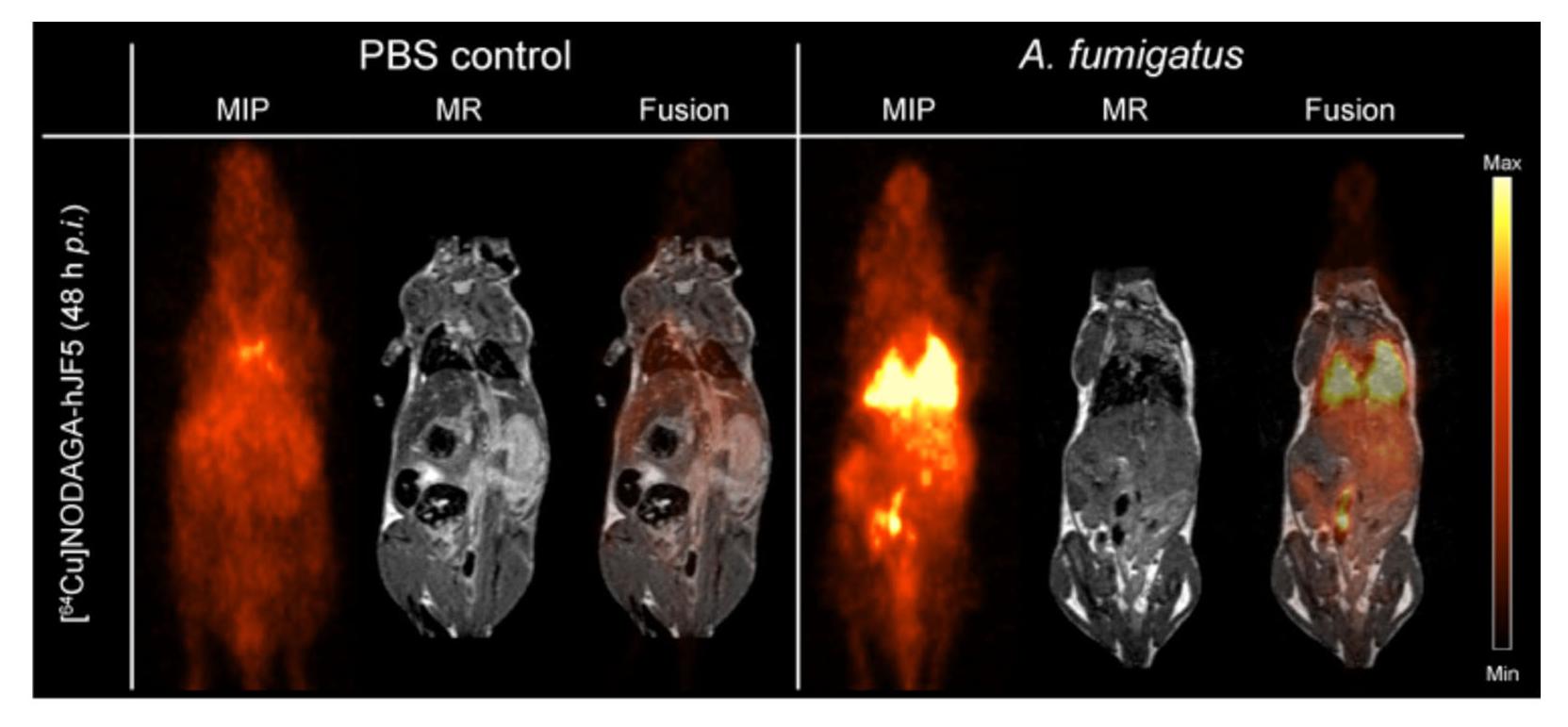
FIGURE 2. ImmunoPET/MR imaging of IPA using the humanized Aspergillus-specific tracer [64Cu]NODAGA-hJF5. The images show coronal maximum intensity projections (MIP), magnetic resonance (MR) imaging, and fused positron emission tomography (PET)/MR images of uninfected mice (PBS control) and mice infected with A. fumigatus. In order to render animals neutropenic, they received an intraperitoneal injection of the antibody RB6-8C5 and, 24 h later, were injected with the pathogen and [64Cu]NODAGA-hJF5 tracer, according to the experimental procedure shown in Figure 1. Forty-eight hours after injection with the pathogen and humanized tracer, PET/MR imaging shows specific accumulation of the tracer in A. fumigatus-infected lung tissues. Image courtesy of MATHIAS Consortium.
Using targeted deletion of the gene encoding UDP-galactopyranose mutase, an enzyme involved in the production of galactofuranose-containing glyco-conjugates in Aspergillus species, mAb JF5 has been shown to bind to β1,5-galactofuranose (Galf), an immuno-dominant epitope present in its (galacto)mannoprotein target (Davies et al., 2017). The absence of the epitope Galf in mammalian carbohydrates (Tefsen et al., 2012), in addition to the enhanced imaging capabilities of the hJF5 antibody, reduces the likelihood of the [64Cu]NODAGA-hJF5 tracer binding to human structures non-specifically, while providing a highly sensitive, non-invasive, procedure for visualizing real-time Aspergillus infections of the human lung.
While the pre-clinical imaging studies in the mouse model of disease have shown the enormous potential of the humanized antibody tracer to detect IPA in the context of neutropenia, a number of issues have yet to be resolved. The ability of the tracer to detect chronic semi-invasive aspergillosis syndromes in the setting of underlying lung diseases such as COPD and cystic fibrosis has yet to be established, as has its ability to detect IPA in immunocompetent patients presenting with diffuse bilateral chest infiltrates, with or without cavity (Pathak et al., 2011). In addition, while pre-clinical studies have demonstrated excellent performance of the JF5 tracer in detecting Aspergillus lung infections under high inoculum load, its capability in detecting extra-pulmonary infections involving, for example, the brain, spleen, and bone (Davoudi et al., 2014), and its ability to penetrate necrotic tissues, also needs to be determined. However, given the very low background uptake of the tracer in these organs (Rolle et al., 2016; Davies et al., 2017; Figure 2), it is likely that the [64Cu]NODAGA-hJF5 tracer will be able to detect deep-seated infections in tissues other than the lung.
Despite these caveats, the humanized JF5 antibody represents an ideal candidate for molecular imaging of IPA in humans and translation of the antibody-guided imaging technology to the clinical setting. To this end, the NODAGA-labeled hJF5 antibody has entered mammalian toxicity testing and will enter first-in-human clinical trials in 2018.
Conclusion
Clinical diagnosis of IPA remains extremely challenging, with non-specific patient symptoms, and insufficient specificity and sensitivity of diagnostic biomarker tests. Radiological imaging of the lung is an attractive means of diagnosing invasive fungal infections since it is a non-invasive procedure, but abnormalities seen in a chest CT which are suggestive of IPA are not sufficiently specific for definitive diagnosis of the disease. Attempts have been to improve radiological detection of IPA using [18F]FDG-PET, but uptake of the tracer during IPA is indistinguishable from that seen during cancer, inflammatory reactions, or during bacterial infections. The specificity of PET has been dramatically improved through the use Aspergillus siderophores, and mAbs conjugated to radionuclides, but all studies to date have been conducted in animal models of IPA.
For translation to the clinical setting, the Aspergillus-specific mAb JF5 has been humanized using CDR grafting of the mouse IgG3 heavy and light chain variable fragments into a human IgG1 framework. The humanized antibody (hJF5) is currently undergoing toxicity testing prior to clinical trials to allow immunoPET/MR imaging of IPA in humans with the Aspergillus-specific PET tracer [64Cu]NODAGA-hJF5. Once the accuracy of the tracer has been established in human clinical trials, its cost effectiveness as a hospital diagnostic procedure for IPA, and its usefulness in monitoring disease in humans in response to antifungal treatment, will then need to be established. These are lengthy and costly investigations but, if successful, may herald a new age in molecular imaging of IPA, and could act as a paradigm for antibody-guided imaging of other invasive fungal diseases of humans. As a platform technology, immuno-PET/MR can be used to image any invasive fungal disease providing that well-characterized disease-specific antibodies are available. Highly specific mAbs have been reported for a number of the most serious mold pathogens of humans such as Fusarium, Scedosporium, and Lomentospora (Thornton, 2009, Thornton et al., 2015; Al-Maqtoofi and Thornton, 2016), enabling molecular imaging of invasive diseases (fusariosis and scedosporiosis) caused by these pathogens. However, it is important to note that despite the unparalleled sensitivity and specificity of immuno-PET/MRI, the high cost of its development, clinical evaluation, and implementation in healthcare systems means that it will likely only be accessible in specialist centers, and will not replace but rather complement less sophisticated diagnostic tests such as ELISA, PCR, and LFA.
Author Contributions
The author confirms being the sole contributor of this work and approved it for publication.
Funding
This work was supported by the European Union Seventh Framework Program FP7/2007-2013 under grant 602820.
Conflict of Interest Statement
The author is the Director of the ISCA Diagnostics Ltd. and is the inventor of the patented Aspergillus-specific monoclonal antibody JF5 and the Aspergillus lateral-flow device.
Abbreviations
CT, computed tomography; IFD, invasive fungal disease; IPA, invasive pulmonary aspergillosis; MRI, magnetic resonance imaging, PET, positron emission tomography.
References
Ahn, B.-C., Lee, S.-W., Lee, J., and Kim, C. (2011). Pulmonary aspergilloma mimicking metastasis from papillary thyroid cancer. Thyroid 21, 555–558. doi: 10.1089/thy.2010.0448
Alexander, J., Limaye, A. P., Ko, C. W., Bronner, M. P., and Kowdley, K. V. (2006). Association of hepatic iron overload with invasive fungal infection in liver transplant recipients. Liver Transpl. 12, 1799–1804. doi: 10.1002/lt.20827
Al-Maqtoofi, M., and Thornton, C. R. (2016). Detection of human pathogenic Fusarium species in hospital and communal sink biofilms by using a highly specific monoclonal antibody. Environ. Microbiol. 18, 3620–3634. doi: 10.1111/1462-2920.13233
Ankrah, A. O., Sathekge, M. M., Dierckx, R. A. J. O., and Glaudemans, A. W. J. M. (2016). Imaging fungal infections in humans. Clin. Transl. Imaging 4, 57–72. doi: 10.1007/s40336-015-0159-2
Araz,Ö., Karaman, A., Ucar, E. Y., Bilen, Y., and Subasi, I. D. (2014). DCE-MRI findings of invasive aspergillosis in patient with acute myeloid leukemia. Clin. Respir. J. 8, 248–250. doi: 10.1111/crj.12061
Barnes, R. A. (2013). Directed therapy for fungal infections: focus on aspergillosis. J. Antimicrob. Chemother. 68, 2431–2434. doi: 10.1093/jac/dkt227
Bassetti, M., Carnelutti, A., Muser, D., Righi, E., Petrosillo, N., Di Gregorio, F., et al. (2017). 18F-Fluorodeoxyglucose positron emission tomography and infectious diseases: current applications and future perspectives. Curr. Opin. Infect. Dis. 30, 192–200. doi: 10.1097/QCO.0000000000000354
Baxter, C. G., Bishop, P., Low, S. E., Baiden-Amissah, K., and Denning, D. W. (2011). Pulmonary aspergillosis: an alternative diagnosis to lung cancer after positive [18F]FDG positron emission tomography. Thorax 66, 638–640. doi: 10.1136/thx.2010.155515
Brock, M. (2012). Applications of bioluminescence imaging for in vivo monitoring of fungal infections. Int. J. Microbiol. 2012:956794. doi: 10.1155/2012/956794
Brock, M., Jouvion, G., Droin-Bergère, S., Dussurget, O., Nicola, M.-A., and Ibrahim-Granet, O. (2008). Bioluminescent Aspergillus fumigatus, a new tool for drug efficiency testing and in vivo monitoring of invasive aspergillosis. Appl. Environ. Microbiol. 74, 7023–7035. doi: 10.1128/AEM.01288-08
Brodoefel, H., Vogel, M., Hebart, H., Einsele, H., Vonthein, R., Claussen, C., et al. (2006). Long-term CT follow-up in 40 non-HIV immunocompromised patients with invasive pulmonary aspergillosis: kinetics of CT morphology and correlation with clinical findings and outcome. Am. J. Roentgenol. 187, 404–413. doi: 10.2214/AJR.05.0513
Brown, G. D., Denning, D. W., Gow, N. A. R., Levitz, S. M., Netea, M. G., and White, T. C. (2012). Hidden killers: human fungal infections. Sci. Transl. Med. 4:165rv13. doi: 10.1126/scitranslmed.3004404
Bullen, J. J., Rogers, H. J., Spalding, P. B., and Ward, C. G. (2006). Natural resistance, iron and infections: a challenge for clinical medicine. J. Med. Microbiol. 55, 251–258. doi: 10.1099/jmm.0.46386-0
Caillot, D., Casasnovas, O., Bernard, A., Couaillier, J. F., Durand, C., Cuisenier, B., et al. (1997). Improved management of invasive pulmonary aspergillosis in neutropenic patients using early thoracic computed tomographic scan and surgery. J. Clin. Oncol. 15, 139–147. doi: 10.1200/JCO.1997.15.1.139
Carroll, C. S., Amankwa, K. N., Pinto, L. J., Fuller, J. D., and Moore, M. M. (2016). Detection of a serum siderophore by LC-MS/MS as a potential biomarker of invasive aspergillosis. PLoS One 11:e0151260. doi: 10.1371/journal.pone.0151260
Casal, R. F., Adachi, R., Jimenez, C. A., Sarkiss, M., Morice, R. C., and Eapen, G. A. (2009). Diagnosis of invasive Aspergillus tracheobronchitis facilitated by endobronchial ultrasound-guided transbronchial needle aspiration: a case report. J. Med. Case Rep. 3:9290. doi: 10.1186/1752-1947-3-9290
Chamilos, G., Macapinlac, H. A., and Kontoyiannis, D. P. (2008). The use of 18F-fluorodeoxyglucose positron emission tomography for the diagnosis and management of of invasive mould infections. Med. Mycol. 46, 23–29. doi: 10.1080/13693780701639546
Davies, G., Rolle, A.-M., Maurer, A., Spycher, P. R., Schillinger, C., Solouk-Saran, D., et al. (2017). Towards translational ImmunoPET/MR imaging of invasive pulmonary aspergillosis: the humanised monoclonal antibody JF5 detects Aspergillus lung infections in vivo. Theranostics 7, 3398–3414. doi: 10.7150/thno.20919
Davoudi, S., Anderlini, P., Fuller, G. N., and Kontoyiannis, D. P. (2014). A long-term survivor of disseminated Aspergillus and Mucorales infection: an instructive case. Mycopathologia 178, 465–470. doi: 10.1007/s11046-014-9785-x
De Pauw, B., Walsh, T. J., Donnelly, J. P., Stevens, D. A., Edwards, J. E., Calandra, T., et al. (2008). Revised definitions of invasive fungal disease from the European Organisation for Research and Treatment of Cancer/invasive fungal infections cooperative group and the National Institute of Allergy and Infectious Diseases Mycoses Study Group (EORTC/MSG) consensus group. Clin. Infect. Dis. 46, 1813–1821. doi: 10.1086/588660
d’Enfert, C., Vecchiarella, A., and Brown, A. J. P. (2010). Bioluminescent fungi for real-time monitoring of fungal infections. Virulence 1, 174–176. doi: 10.4161/viru.1.3.11119
Dignan, F. L., Evans, S. O., Ethell, M. E., Shaw, B. E., Davies, F. E., Dearden, C. E., et al. (2009). An early CT-diagnosis based treatment strategy for invasive fungal infection in allogeneic transplant recipients using caspofungin first line: an effective strategy with low mortality. Bone Marrow Transpl. 44, 51–56. doi: 10.1038/bmt.2008.427
Doyle, T. C., Nawotka, K. A., Kawahara, C. B., Francis, K. P., and Contag, P. R. (2006). Visualising fungal infections in living mice using bioluminescent pathogenic Candida albicans strains transformed with the firefly luciferase gene. Microb. Pathog. 40, 82–90. doi: 10.1016/j.micpath.2005.11.003
Franzius, C., Biermann, M., Hülskamp, G., Frosch, M., Roth, J., Sciuk, J., et al. (2001). Therapy monitoring in aspergillosis using F-18 FDG positron emission tomography. Clin. Nucl. Med. 26, 232–233. doi: 10.1097/00003072-200103000-00011
Galiger, C., Brock, M., Jouvion, G., Savers, A., Parlato, M., and Ibrahim-Granet, O. (2013). Assessment of efficacy of antifungals against Aspergillus fumigatus: value of real-time bioluminescence imaging. Antimicrob. Agents Chemother. 57, 3046–3059. doi: 10.1128/AAC.01660-12
Garcia-Olivé, I., Andreo, P., Rosiñol, O., Sanz-Santos, J., Font, A., and Monsó, R. (2011). Bronchial stump aspergillosis after lobectomy for lung cancer as an unusual cause of false positive fluorodeoxyglucose positron emission tomography and computed tomography: a case report. J. Med. Case Rep. 5:72. doi: 10.1186/1752-1947-5-72
Georgiadou, S. P., Sipsas, N. V., Marom, E. M., and Kontoyiannis, D. P. (2011). The diagnostic value of halo and reversed halo signs for invasive mold infections in compromised hosts. Clin. Infect. Dis. 52, 1144–1155. doi: 10.1093/cid/cir122
Glaudemans, A. W., Slart, R. H., van Dijl, J. M., van Oosten, M., and van Dam, G. M. (2015). Molecular imaging of infectious and inflammatory diseases: a terra incognita. J. Nucl. Med. 56, 659–661. doi: 10.2967/jnumed.115.155119
Glaudemans, A. W. J. M., Quintero, A. M., and Signore, A. (2012). PET/MRI in infectious and inflammatory disease: will it be a useful improvement? Eur. J. Nucl. Med. Mol. Imaging 39, 745–749. doi: 10.1007/s00259-012-2060-9
Goméz, M. D. V., Gallardo, F. G., Babe, J., and Cobo, J. (2000). Ga-67 scan in invasive aspergillosis: a case report. Clin. Nucl. Med. 25, 1035–1036. doi: 10.1097/00003072-200012000-00020
Greene, R. (2005). The radiological spectrum of pulmonary aspergillosis. Med. Mycol. 43(Suppl.), S147–S154. doi: 10.1080/13693780500064771
Greene, R. E., Schlamm, H. T., Oestmann, J.-W., Stark, P., Durand, C., Lortholary, O., et al. (2007). Imaging findings in acute invasive pulmonary aspergillosis: clinical significance of the halo sign. Clin. Infect. Dis. 44, 373–379. doi: 10.1086/509917
Haas, H. (2003). Molecular genetics of fungal siderophore biosynthesis and uptake: the role of siderophores in iron uptake and storage. Appl. Microbiol. Biotechnol. 62, 316–330. doi: 10.1007/s00253-003-1335-2
Haas, H., Petrik, M., and Descristoforo, C. (2015). An iron-mimicking, Trojan horse-entering fungi - has the time come for molecular imaging of fungal infections? PLoS Pathog. 11:e1004568. doi: 10.1371/journal.ppat.1004568
Held, J., Schmidt, T., Thornton, C. R., Kotter, E., and Bertz, H. (2013). Comparison of a novel Aspergillus lateral-flow device and the Platelia galactomannan assay for diagnosis of invasive aspergillosis following haematopoietic stem cell transplantation. Infection 41, 1163–1169. doi: 10.1007/s15010-013-0472-5
Hissen, A. H. T., Chow, J. M. T., Pinto, L. J., and Moore, M. M. (2004). Survival of Aspergillus fumigatus in serum involves removal of iron from transferrin: the role of siderophores. Infect. Immun. 72, 1402–1408. doi: 10.1128/IAI.72.3.1402-1408.2004
Hissen, A. H. T., Wan, A. N. C., Warwas, M. L., Pinto, L. J., and Moore, M. M. (2005). The Aspergillus fumigatus siderophore biosynthetic gene sidA, encoding L-ornithine N5-oxygenase, is required for virulence. Infect. Immun. 73, 5493–5503. doi: 10.1128/IAI.73.9.5493-5503.2005
Ho, A. Y., Pagliuca, A., Maisey, M. N., and Mufti, G. J. (1998). Positron emission scanning with 18-FDG in the diagnosis of deep fungal infections. Br. J. Haematol. 100, 392–393. doi: 10.1046/j.1365-2141.1998.0738e.x
Hoenigl, M., Eigl, S., Heldt, S., Duettmann, W., Thornton, C., and Prattes, J. (2017). Clinical evaluation of the newly formatted lateral-flow device for invasive pulmonary aspergillosis. Mycoses 61, 40–43. doi: 10.1111/myc.12704
Hot, A., Maunoury, C., Poiree, S., Lanternier, F., Viard, J. P., Loulergue, P., et al. (2011). Diagnostic contribution of positron emission tomography with [18F]fluorodeoxyglucose for invasive fungal infections. Clin. Microbiol. Infect. 17, 409–417. doi: 10.1111/j.1469-0691.2010.03301.x
Huang, L., He, H., Ding, Y., Jin, J., and Zhan, Q. (2016). Values of radiological examinations for the diagnosis and prognosis of invasive bronchial-pulmonary aspergillosis in critically ill patients with chronic obstructive pulmonary diseases. Clin. Resp. J. 12, 499–509. doi: 10.1111/crj.12551
Ibrahim-Granet, O., Jouvion, G., Hohl, T. M., Droin-Bergère, S., Philippart, F., Kim, O. Y., et al. (2010). In vivo bioluminescence imaging and histopathologic analysis reveal distinct roles for resident and recruited immune effector cells in defense against invasive aspergillosis. BMC Microbiol. 10:105. doi: 10.1186/1471-2180-10-105
Imperiale, A., Federici, L., Lefebvre, N., Braun, J.-J., Pfumio, F., Kessler, R., et al. (2010). F-18 FDG PET/CT as a valuable imaging tool for assessing treatment efficacy in inflammatory and infectious diseases. Clin. Nucl. Med. 35, 86–90. doi: 10.1097/RLU.0b013e3181c7be8d
Jacobsen, I. D., Luttich, A., Kurzai, O., Hube, B., and Brock, M. (2014). In vivo imaging of disseminated murine Candida albicans infection reveals unexpected host sites of fungal persistence during antifungal therapy. J. Antimicrob. Chemother. 69, 2785–2796. doi: 10.1093/jac/dku198
Jain, S. (2017). The promise of molecular imaging in the study and treatment of infectious diseases. Mol. Imaging Biol. 19, 341–347. doi: 10.1007/s11307-017-1055-0
Kim, J. Y., Yoo, J.-W., Oh, M., Park, S. H., Shim, T. S., Choi, Y. Y., et al. (2013). 18F-fluoro-2-deoxy-D-glucose positron emission tomography/computed tomography findings are different between invasive and noninvasive pulmonary aspergillosis. J. Comput. Assist. Tomogr. 37, 596–601. doi: 10.1097/RCT.0b013e318289aa31
Leal, S. M., Roy, S., Vareechon, C., de Jesus Carrion, S., Clark, H., Lopez-Berges, M. S., et al. (2013). Targeting iron acquisition blocks infection with the fungal pathogens Aspergillus fumigatus and Fusarium oxysporum. PLoS Pathog. 9:e1003436. doi: 10.1371/journal.ppat.1003436
Lesuisse, E., Knight, S. A. B., Camadro, J.-M., and Dancis, A. (2002). Siderophore upake by Candida albicans: effect of serum treatment and comparison with Saccharomyces cerevisiae. Yeast 19, 329–340. doi: 10.1002/yea.840
Lupetti, A., Welling, M. K., Mazzi, U., Nibbering, P. H., and Pauwels, E. K. J. (2002). Technetium-99m labeled fluconazole and antimicrobial peptides for imaging of Candida albicans and Aspergillus fumigatus infections. Eur. J. Nucl. Med. 29, 674–679. doi: 10.3109/13693786.2010.508188
Marzolf, G., Sabou, M., Lannes, B., Cotton, F., Meyronet, D., Galanaud, D., et al. (2016). Magnetic resonance imaging of cerebral aspergillosis: imaging and pathological correlations. PLoS One 11:e0152475. doi: 10.1371/journal.pone.0152475
Mucha, K., Foroncewicz, B., Orlowski, T., Religioni, J., Bobek-Billewicz, B., Jarzab, B., et al. (2013). Atypical presentation of invasive aspergillosis in a liver transplant recipient. Ann. Transplant. 18, 238–242. doi: 10.12659/AOT.883921
Nakajima, H., Sawaguchi, H., Hoshi, S., Nakajima, S., and Tohda, Y. (2009). Intense 18F-fluorodeoxyglucose uptake due to allergic bronchopulmonary aspergillosis. Arerugi 58, 1426–1432.
Nishikawa, T., Kagawa, T., Matsumi, Y., Fujiwara, T., Kataoka, K., and Matsuura, M. (2011). Lung cancer associated with pulmonary aspergillosis in the nodule of old mycobacterial infection. Kyobu Geka 64, 231–234.
Oberegger, H., Schoeser, M., Zadra, I., Abt, B., and Haas, H. (2001). SREA is involved in regulation of siderophore biosynthesis, utilization and uptake in Aspergillus nidulans. Mol. Microbiol. 41, 1077–1089. doi: 10.1046/j.1365-2958.2001.02586.x
Ozsahin, H., von Planta, M., Müller, I., Steinert, H. C., Nadal, D., Lauener, R., et al. (1998). Successful treatment of invasive aspergillosis in chronic granulomatous disease by bone marrow transplantation, granulocyte colony-stimulating factor-mediated granulocytes, and liposomal amphotericin-B. Blood 92, 2719–2724.
Pagano, L., Akova, M., Dimopoulos, G., Herbrecht, R., Drgona, L., and Blijlevens, N. (2011). Risk assessment and prognostic factors for mould-related diseases in immunocompromised patients. J. Antimicrob. Chemother. 66, i5–i14. doi: 10.1093/jac/dkq437
Panse, P., Smith, M., Cummings, K., Jensen, E., Gotway, M., and Jokerst, C. (2016). The many faces of pulmonary aspergillosis: imaging findings with pathologic correlation. Radiol. Infect. Dis. 3, 192–200. doi: 10.1016/j.jrid.2016.10.002
Pathak, V., Rendon, I. S. H., and Ciubotaru, R. L. (2011). Invasive pulmonary aspergillosis in an immunocompetent patient. Respir. Med. CME 4, 105–106. doi: 10.1016/j.rmedc.2010.12.004
Petrik, M., Fransenn, G. M., Haas, H., Laverman, P., Hörtnagl, C., Schretti, M., et al. (2012a). Preclinical evaluation of two 68Ga-siderophores as potential radiopharmaceuticals for Aspergillus fumigatus infection imaging. Eur. J. Nucl. Med. Mol. Imaging 39, 1175–1183. doi: 10.1007/s00259-012-2110-3
Petrik, M., Haas, H., Schrettl, M., Helbok, A., Blatzer, M., and Decristiforo, C. (2012b). In vitro and in vivo evaluation of selected (68)Ga-siderophores for infection imaging. Nucl. Med. Biol. 39, 361–369. doi: 10.1016/j.nucmedbio.2011.09.012
Petrik, M., Haas, H., Dobrozemsky, G., Lass-Flörl, C., Helbok, A., Blatzer, M., et al. (2010). 68Ga-siderophores for PET imaging of invasive pulmonary aspergillosis: proof of principle. J. Nucl. Med. 51, 639–645. doi: 10.2967/jnumed.109.072462
Petrik, M., Haas, H., Laverman, P., Schrettl, M., Franssen, G. M., Blatzer, M., et al. (2014). 68Ga-triacetylfusarinine C and 68Ga-ferrioxamine E for Aspergillus infection imaging: uptake specificity in various microorganisms. Mol. Imaging Biol. 16, 102–108. doi: 10.1007/s11307-013-0654-7
Petrik, M., Zhai, C., Novy, Z., Urbanek, L., Haas, H., and Descristoforo, C. (2016). In vitro and in vivo comparison of selected Ga-68 and Zr-89 labelled siderophores. Mol. Imaging Biol. 18, 344–352. doi: 10.1007/s11307-015-0897-6
Poelmans, J., Hillen, A., Vanherp, L., Govaerts, K., Maertans, J., Dresselaers, T., et al. (2016). Longitudinal, in vivo assessment of invasive pulmonary aspergillosis in mice by computed tomography and magnetic resonance imaging. Lab. Invest. 96, 692–704. doi: 10.1038/labinvest.2016.45
Prasad, A., Agarwal, K., Deepak, D., and Atwal, S. S. (2016). Pulmonary aspergillosis: what CT can offer before it is too late! J. Clin. Diagn. Res. 10, TE01–TE05. doi: 10.7860/JCDR/2016/17141.7684
Prattes, J., Prueller, F., Koidl, C., Raggam, R. B., Palfner, M., Eigl, S., et al. (2014). Novel tests for diagnosis of invasive pulmonary aspergillosis in patients with underlying respiratory diseases. Am. J. Respir. Crit. Care Med. 190, 922–929. doi: 10.1164/rccm.201407-1275OC
Rolle, A.-M., Hasenberg, M., Thornton, C. R., Solouk-Saran, D., Männ, L., Weski, J., et al. (2016). ImmunoPET/MR imaging allows specific detection of Aspergillus fumigatus lung infection in vivo. Proc. Natl. Acad. Sci. U.S.A. 113, E1026–E1033. doi: 10.1073/pnas.1518836113
Rolle, A.-M., and Wiehr, S. (2017). “Molecular imaging of infectious diseases,” in Small Animal Imaging, eds F. Kiessling, B. J. Pichler, and P. Hauff (Cham: Springer International Publishing), 845–856.
Santangelo, P. J., Rogers, K. A., Zurla, C., Blanchard, E. L., Gumber, S., Strait, K., et al. (2014). Whole-body immunoPET reveals active SIV dynamics in viremic and antiretroviral therapy-treated macaques. Nat. Methods 12, 427–432. doi: 10.1038/nmeth.3320
Schrettl, M., Bignell, E., Kragl, C., Joechl, C., Rogers, T., Arst, H. N., et al. (2004). Siderophore biosynthesis but not reductive iron assimilation is essential for Aspergillus fumigatus virulence. J. Exp. Med. 200, 1213–1219. doi: 10.1084/jem.20041242
Sharma, P., Mukherjee, A., Karunanithi, S., Bal, C., and Kumar, R. (2014). Potential role of 18F-FDG PET/CT in patients with fungal infections. Am. J. Radiol. 203, 180–189. doi: 10.2214/AJR.13.11712
Signore, A., Glaudemans, A. W., Galli, F., and Rouzet, F. (2015). Imaging infection and inflammation. BioMed. Res. Int. 2015:615150. doi: 10.1155/2015/615150
Sonet, A., Graux, C., Nollevaux, M. C., Krug, B., Bosly, A., and Vander Borght, T. (2007). Unsuspected FDG-PET findings in the follow-up of patients with lymphoma. Ann. Hematol. 86, 9–15. doi: 10.1007/s00277-006-0167-4
Stanzani, M., Sassi, C., Battista, G., Cavo, M., and Lewis, R. E. (2016). Improved radiographic imaging of invasive fungal disease: the cornerstone of antifungal stewardship in the hematology units? Curr. Fung. Infect. Rep. 10, 78–86. doi: 10.1007/s12281-016-0258-1
Stanzani, M., Sassi, C., Lewis, R. E., Tolomelli, G., Bazzocchi, A., Cavo, M., et al. (2015). High resolution computed tomography angiography improves the radiographic diagnosis of invasive mold disease in patients with hematological malignancies. Clin. Infect. Dis. 60, 1603–1610. doi: 10.1093/cid/civ154
Starkey, J., Moritani, T., and Kirby, P. (2014). MRI and CNS fungal infections: review of aspergillosis to histoplasmosis and everything else. Clin. Neuroradiol. 24, 217–230. doi: 10.1007/s00062-014-0305-7
Tefsen, B., Ram, A. F. J., van Die, I., and Routier, F. H. (2012). Galactofuranose in eukaryotes: aspects of biosynthesis and functional impact. Glycobiology 22, 456–469. doi: 10.1093/glycob/cwr144
Thornton, C. R. (2008). Development of an immunochromatographic lateral-flow device for rapid serodiagnosis of invasive aspergillosis. Clin. Vaccine Immunol. 15, 1095–1105. doi: 10.1128/CVI.00068-08
Thornton, C. R. (2009). Tracking the emerging human pathogen Pseudallescheria boydii using highly specific monoclonal antibodies. Clin. Vaccine Immunol. 16, 756–764. doi: 10.1128/CVI.00061-09
Thornton, C. R. (2014). Breaking the mould - novel diagnostic and therapeutic strategies for invasive pulmonary aspergillosis in the immune deficient patient. Exp. Rev. Clin. Immunol. 6, 771–780. doi: 10.1586/1744666X.2014.904747
Thornton, C. R., Ryder, L. S., Le Cocq, K., and Soanes, D. M. (2015). Identifying the emerging human pathogen Scedosporium prolificans by using a species-specific monoclonal antibody that binds to the melanin biosynthetic enzyme tetrahydroxynaphthalene reductase. Environ. Microbiol. 17, 1023–1038. doi: 10.1111/1462-2920.12470
Thornton, C. R., and Wills, O. E. (2015). Immunodetection of fungal and oomycete pathogens: established and emerging threats to human health, animal welfare and global food security. Crit. Rev. Microbiol. 41, 27–51. doi: 10.3109/1040841X.2013.788995
Tzen, K.-Y., Yen, T.-C., and Lin, K.-J. (1999). Value of Ga-67 SPECT in monitoring the effects of therapy in invasive aspergillosis of the sphenoid sinus. Clin. Nucl. Med. 24, 938–941. doi: 10.1097/00003072-199912000-00006
Velde, G. V., and Wiehr, S. (2017). “Fungal imaging,” in Imaging Infections, ed. S. J. Jain (Cham: Springer International Publishing), 173–183. doi: 10.1007/978-3-319-54592-9_7
Wang, Y., Chen, L., Liu, X., Cheng, D., Liu, G., Liu, Y., et al. (2013). Detection of Aspergillus fumigatus pulmonary fungal infections in mice with 99mTc-labeled MORF oligomers targeting ribosomal RNA. Nucl. Med. Biol. 40, 89–96. doi: 10.1016/j.nucmedbio.2012.10.001
White, P. L., Parr, C., Thornton, C. R., and Barnes, R. A. (2013). An evaluation of real-time PCR, galactomannan ELISA and a novel lateral-flow device for the diagnosis of invasive aspergillosis. J. Clin. Microbiol. 51, 1510–1516. doi: 10.1128/JCM.03189-12
Wiehr, S., Warnke, P., Rolle, A.-M., Schütz, M., Oberhettinger, P., Kohlhofer, U., et al. (2016). New pathogen-specific immunoPET/MR tracer for molecular imaging of a systemic bacterial infection. Oncotarget 7, 10990–11001. doi: 10.18632/oncotarget.7770
Wilkinson, M. D., Fulham, M. J., McCaughan, B. C., and Constable, C. J. (2003). Invasive aspergillosis mimicking stage IIIA non-small-cell lung cancer on FDG positron emission tomography. Clin. Nucl. Med. 28, 234–235. doi: 10.1097/01.RLU.0000053535.03453.D9
Willinger, B., Lackner, M., Lass-Florl, C., Prattes, J., Posch, V., Selitsch, B., et al. (2014). Bronchoalveolar lavage lateral-flow device test for invasive pulmonary aspergillosis in solid organ transplant patients: a semi-prospective multicenter study. Transplantation 98, 898–902. doi: 10.1097/TP.0000000000000153
Keywords: monoclonal antibody (mAb), invasive pulmonary aspergillosis, positron emission tomography, magnetic resonance imaging, immunoPET-MRI
Citation: Thornton CR (2018) Molecular Imaging of Invasive Pulmonary Aspergillosis Using ImmunoPET/MRI: The Future Looks Bright. Front. Microbiol. 9:691. doi: 10.3389/fmicb.2018.00691
Received: 28 November 2017; Accepted: 23 March 2018;
Published: 09 April 2018.
Edited by:
Helmut J. F. Salzer, Forschungszentrum Borstel (LG), GermanyReviewed by:
Dimitrios P. Kontoyiannis, University of Texas MD Anderson Cancer Center, United StatesGeorgios Chamilos, University of Crete, Greece
Copyright © 2018 Thornton. This is an open-access article distributed under the terms of the Creative Commons Attribution License (CC BY). The use, distribution or reproduction in other forums is permitted, provided the original author(s) and the copyright owner are credited and that the original publication in this journal is cited, in accordance with accepted academic practice. No use, distribution or reproduction is permitted which does not comply with these terms.
*Correspondence: Christopher R. Thornton, C.R.Thornton@ex.ac.uk