Molecular and cellular origins of behavioral sex differences: a tiny little fly tells a lot
- Neuro-ICT Laboratory, Advanced ICT Research Institute, National Institute of Information and Communications Technology, Kobe, Japan
Behavioral sex differences primarily derive from the sexually dimorphic organization of neural circuits that direct the behavior. In Drosophila melanogaster, the sex-determination genes fruitless (fru) and doublesex (dsx) play pivotal roles in producing the sexual dimorphism of neural circuits for behavior. Here we examine three neural groups expressing fru and/or dsx, i.e., the P1 cluster, aSP-f and aSP-g cluster pairs and aDN cluster, in which causal relationships between the dimorphic behavior and dimorphic neural characteristics are best illustrated. aSP-f, aSP-g and aDN clusters represent examples where fru or dsx switches cell-autonomously their neurite structures between the female-type and male-type. Processed sensory inputs impinging on these neurons may result in outputs that encode different valences, which culminate in the execution of distinct behavior according to the sex. In contrast, the P1 cluster is male-specific as its female counterpart undergoes dsx-driven cell death, which lowers the threshold for the induction of male-specific behaviors. We propose that the products of fru and dsx genes, as terminal selectors in sexually dimorphic neuronal wiring, induce and maintain the sex-typical chromatin state at postembryonic stages, orchestrating the transcription of effector genes that shape single neuron structures and govern cell survival and death.
Introduction: studying fly mating unveils the logic of brain circuit rearrangements
Behavioral sex difference is widespread in the animal kingdom. The most conspicuous cases are often found in mating behavior, where a female and a male are driven by conflicting interests for their own reproductive success, yet cooperation between the two individuals is indispensable for successful reproduction. Faced with such conflicting demands, selective pressure will act to exaggerate the dimorphic traits in both morphology and behavior (Price et al., 2023; Tosto et al., 2023). Our understanding of the genetic and cellular mechanisms for morphological sexual differences has been fueled by the rapid grow of the evo-devo field, but the mechanistic basis of behavioral sexual dimorphisms remains enigmatic, primarily because the complex architecture of neural circuits underlying behaviors has hampered the cell-by-cell analysis of dimorphic connectivity.
Drosophila melanogaster provides a suitable experimental system for unraveling the neural substrates of dimorphic behaviors, because sophisticated genetic techniques for labeling and manipulation of neurons are available, in conjunction with applicability of electrophysiology and Ca2+-imaging for neural activity recordings in live, behaving animals, allowing one to identify the neuron that plays a key role in the expression of dimorphic behaviors (Yamamoto, 2022). Since D. melanogaster is one of only a few species in which the whole brain connectome has been completed (Scheffer et al., 2020; Dorkenwald et al., 2021), the neurons found to be important for a particular behavior can immediately be mapped in the deduced connectomic circuits.
Indeed, the circuits for sociosexual behaviors such as courtship and aggression have been particularly well characterized in D. melanogaster (Koganezawa et al., 2016; Asahina, 2017; Chiu et al., 2021; Jiang and Pan, 2022), since two sex determination genes fruitless (fru) and doublesex (dsx) play instructing roles for the configuration of the sex-specific circuit, often referred to as the fru/dsx-circuit (Cachero et al., 2010; Yu et al., 2010), and consequently, the molecular and genetic resources derived from these two genes (e.g., a large array of transgenic and mutant flies and specific antibodies) offer an unparalleled opportunity to selectively manipulate the sex-specific circuit with a minimal disturbance of other neural networks in the brain (Demir and Dickson, 2005; Rideout et al., 2010; Neville et al., 2021; Sato and Yamamoto, 2022).
The organization and function of the fru/dsx-circuit has been reviewed several times (Yamamoto and Koganezawa, 2013; Coen and Murthy, 2016; Ellendersen and von Philipsborn, 2017) and we do not intend to repeat a general overview of this circuit. Instead, this article focuses on a few select studies that successfully unveiled how a specific cellular sex difference in an identified neuron could lead to a clear sex difference in certain behavior. We also attempt to construct a coherent framework in which fru/dsx gene products reconfigure subcellular structures of a neuron so that the neuron is incorporated into a female-typical or male-typical circuit that produces a sex-specific behavior.
Male-specific P1 neurons: are they essential for executing male courtship?
The P1 neuron cluster is composed of (per hemibrain) 20 male-specific neurons that express both the male-specific fru product FruM and the male-specific dsx product DsxM (Kimura et al., 2008). It is a prevalent view that P1 neurons are essential for males in initiating courtship behavior toward a female. In fact, P1 neurons were discovered by an unbiased screen of mosaic females that carry tens of masculinized fru expressing neurons for the ability to court another female with a male-typical posture, i.e., unilateral wing vibration: the P1 cluster was the sole masculinized neuron group that was found significantly more often in courtship-positive females than courtship-negative females (p < 0.0001 by Fisher’s exact probability test; Kimura et al., 2008). In other words, a few mosaic females did not have a masculinized P1 cluster, but they nonetheless displayed male-typical courtship behavior. This initial observation already indicated that the presence of P1 neurons is not essential for the initiation of male courtship (even in mosaic females). This important point was thoroughly discussed in a review article by Siwicki and Kravitz (2009) that appeared soon after the publication of the original article. As a matter of fact, 5 months prior to the report of Kimura et al. (2008) and Clyne and Miesenböck (2008) reported that decapitated females were able to generate a quasi-normal courtship (pulse) song when the neurons in the ventral nerve cord were artificially activated via P2X2, although the temporal structure of the song was distorted. An even more striking observation was made by Demir and Dickson (2005), who demonstrated that females that transgenically express the male-specific FruM display male-typical courtship toward other female flies. Notably, these FruM-overexpressing females do not have P1 neurons, because they are females and FruM has nothing to do with the presence or absence of P1 neurons (see below; Kimura et al., 2008). However, we found that the courting activity of these females that lack P1 neurons was very low. In male flies, thermogenetic or optogenetic activation of P1 neurons readily induced courtship pursuit particularly when such males were, at the same time, pheromonally stimulated, or visually stimulated by not only a moving female but also a moving dummy or even moving stripes displayed on a computer screen (Kohatsu et al., 2011; Pan et al., 2012; Kohatsu and Yamamoto, 2015; see also Agrawal et al., 2014 for the role of vision). P1 neurons were shown to respond to these chemical and visual stimuli with Ca2+ rises, which were sustained beyond the stimulation period under constrained conditions (Inagaki et al., 2014) or recurrently occurred while the male pursued a courtship target (Kohatsu and Yamamoto, 2015). These observations collectively indicate that P1 neurons are dispensable for generating a series of courtship actions. Rather, P1 neurons seem to boost up male courtship activity and increase the coherence among distinct courtship actions and time organizations. Therefore, the presence or absence of P1 neurons does not produce a qualitative difference between the female and male courtship behavior, but P1 neuron activities increase the level and fidelity of male courtship behavior.
aSP-f and aSP-g: changeover switch of behavioral sex types in a pheromone pathway
cis-vaccenyl acetate (cVA) is synthesized in the male ejaculatory bulb and transferred to the female uterus with seminal fluid (Brieger and Butterworth, 1970; Guiraudie-Capraz et al., 2007), attenuating attractiveness of the female as a mating partner for males; it thus acts as a mating inhibitory pheromone for males (Siegel and Hall, 1979). However, for females, and even for males under certain conditions, cVA is attractive and induces aggregation (Bartelt et al., 1985; Cortot et al., 2022). The neural basis for the sexually dimorphic actions of cVA has been successfully tracked back to two identified clusters of neurons, aSP-f (also known as DC1; Figure 1A) and aSP-g (Figure 1B), which are third-order interneurons in the olfactory center lateral horn (Kohl et al., 2013). Patch clamp recordings from MARCM clone neurons revealed that male aSP-f and female aSP-g receive monosynaptic inputs from DA1 projection neurons, which relay cVA signals originating from antennal sensory neurons that express Odorant receptor 67d (Or67d) (Datta et al., 2008) to the lateral horn via the sexually dimorphic DA1 glomerulus of the antennal lobe (Kondoh et al., 2003; Stockinger et al., 2005). aSP-f/DC1 and aSP-g neurons each send output to different postsynaptic neurons, so that the behavioral outcome of sensing cVA becomes distinctly different between the sexes—i.e., attraction in females and repulsion in males (Kohl et al., 2013). Loss of FruM in mutant MARCM clones in male flies transforms the dendritic field of these two neural types: aSP-f/DC1 loses, while aSP-g acquires, morphological and electrophysiological connections with DA1 projection neurons. Thus, FruM masculinizes the dendritic patterns of aSP-g and aSP-f/DC1, allowing otherwise non-sex-specific cVA sensory input drives either female-specific or male-specific behavior depending on the fly’s sex (Kohl et al., 2013). In this case, a single transcription factor (FruM) switches the information flow in the brain through its action on neurite patterning according to the sex.
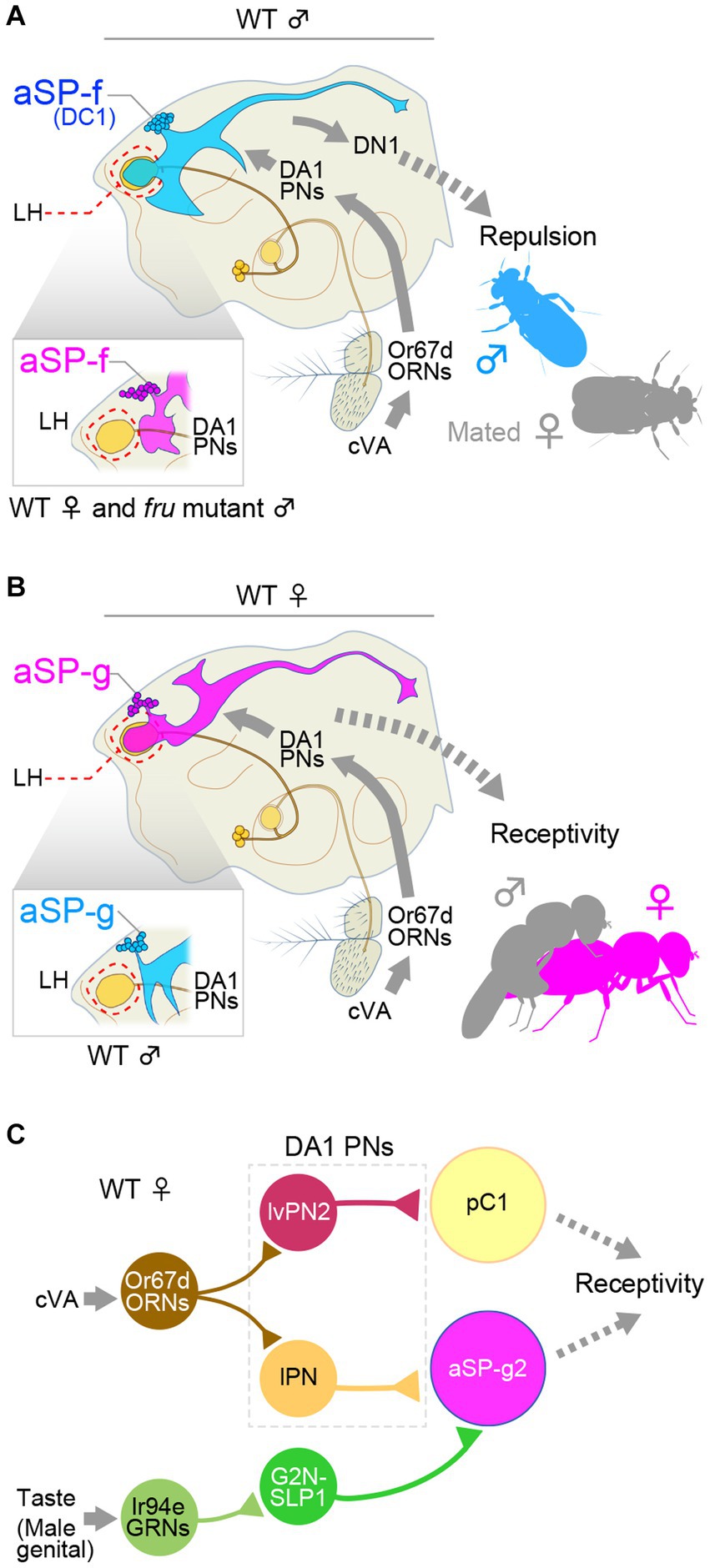
Figure 1. fru-expressing aSP-f and aSP-g interneurons form a changeover switch in the pheromone cis-vaccenyl acetate (cVA)-processing pathways. (A) cVA activates the Odorant receptor 67d (Or67d)-expressing sensory neurons (ORNs) in the antenna, which project to the DA1 glomerulus. DA1 projection neurons (PNs) send their axon to the lateral horn (LH), where they synapse onto aSP-f (DC1) interneurons, which, in turn, connect with a descending interneuron, DN1, in males. (B) In females, DA1 projection neurons synapse onto aSP-g interneurons without forming synapses onto aSP-f interneurons. This bidirectional circuit switch reroutes the cVA signals to different descending neurons, resulting in the distinct behavioral outputs in males and females. (C) A diagram of the circuit integrating olfactory and gustatory information, which controls female receptivity. lPN, lateral projection neuron; lvPN, lateroventral projection neuron; GRNs, gustatory receptor neurons; G2N-SLP1, gustatory second order neuron-superior lateral protocerebrum. Panel (C) was drawn based primarily on the results reported by Kohl et al. (2013) and Taisz et al. (2023).
aSP-f/DC1 in males, then, activates the male-specific descending neuron DN1, although the behavioral outcome of this activation has not been elucidated (Ruta et al., 2010). Additionally, a tachykinin-expressing subpopulation of aSP-f in males was reported to promote same-sex aggression (Asahina et al., 2014). On the other hand, a recent work gave a new twist on the behavioral roles of aSP-g in females (Figure 1C). Taisz et al. (2023) distinguished three morphological subtypes of aSP-g, and showed that only the aSP-g2 population possesses a dendritic field that overlaps with the DA1 projection neuron terminals. Connectome data suggested that aSP-g2 is likely to be postsynaptic to a gustatory interneuron named G2N-SLP1 (an abbreviation of gustatory second order neuron-superior lateral protocerebrum). Taisz et al. (2023) reported that G2N-SLP1 conveys, to aSP-g2, input from Ir94e-expressing labellar gustatory receptor neurons (Ir94e-GRNs), which they found to respond strongly to male genitals, in addition to their known ligands, water and NaCl (Jaeger et al., 2018). Intriguingly, optogenetic activation of both Ir94e-GRNs and c-VA-sensitive olfactory projection neurons, but not either one of the two, promoted female acceptance, and optogenetic activation of aSP-g2 alone recapitulated the effect of gustatory and olfactory dual stimulations (Taisz et al., 2023). These observations elegantly documented that a dendritic sexual dimorphism of a specific neural node offers a decisive mechanism whereby the same sensory input triggers radically different behavioral outputs between the two sexes.
The aDN cluster: crossing the border between visual and olfactory perception
The aDN cluster composed of two pairs of neurons that express dsx but not fru exhibits a sexual dimorphism in neurite distributions and plays important roles in both sexes but in different behavioral contexts: in females, aDN contributes to oviposition site selection (Figure 2A), whereas, in males, it mediates visually guided courtship orientation (Figure 2B; Nojima et al., 2021). This functional diversification of aDN between the sexes was found to stem primarily from a difference in its presynaptic partners (Nojima et al., 2021): male aDN receives input from LC10a visual interneurons (Ribeiro et al., 2018) in the anterior optic tubercle (AOTu), and female aDN receives multimodal sensory input in the posterior part of the superior lateral protocerebrum (pSLP), superior clamp (SCL) and ventrolateral protocerebrum (VLP) with predominating olfactory input from the lateral horn (LH). Indeed, inactivation of aDN induced misdirected orientation in courting males and loss of preference for the medium deposited with male pheromone as an oviposition site in mated females (Nojima et al., 2021). In contrast to the diverged dendritic fields, the output site of aDN remained nearly the same in the two sexes, i.e., the superior medial protocerebrum (SMP). Connectome data indicated that the predominating output neuron for female aDN is the uncharacterized SMP156 neuron, which forms an “efferent” path from the SMP to the lobula in the contralateral optic lobe (Nojima et al., 2021). It remains to be determined whether male aDN similarly connect with a SMP homolog, forming a recurrent path to the lobula. If this is the case, the feedback loop will presumably be used by the male to correct errors in orienting a visual target (Shadmehr et al., 2010). By analogy, olfaction-based navigation of a female fly to a possible oviposition site might be fine-tuned by the SMP efferent path. Thus, this study appears to unveil a deep homology in the logic underlying circuit architecture for two types of navigation, one relying on visual input operating for male-specific behavior and the other relying on olfactory input operating for female-specific behavior. This also represents an economical solution for switching behavioral machineries between the sexes, which can be attained by a simple change in dendritic positioning within just two pairs of neurons as instructed by one sex-determination gene, dsx.
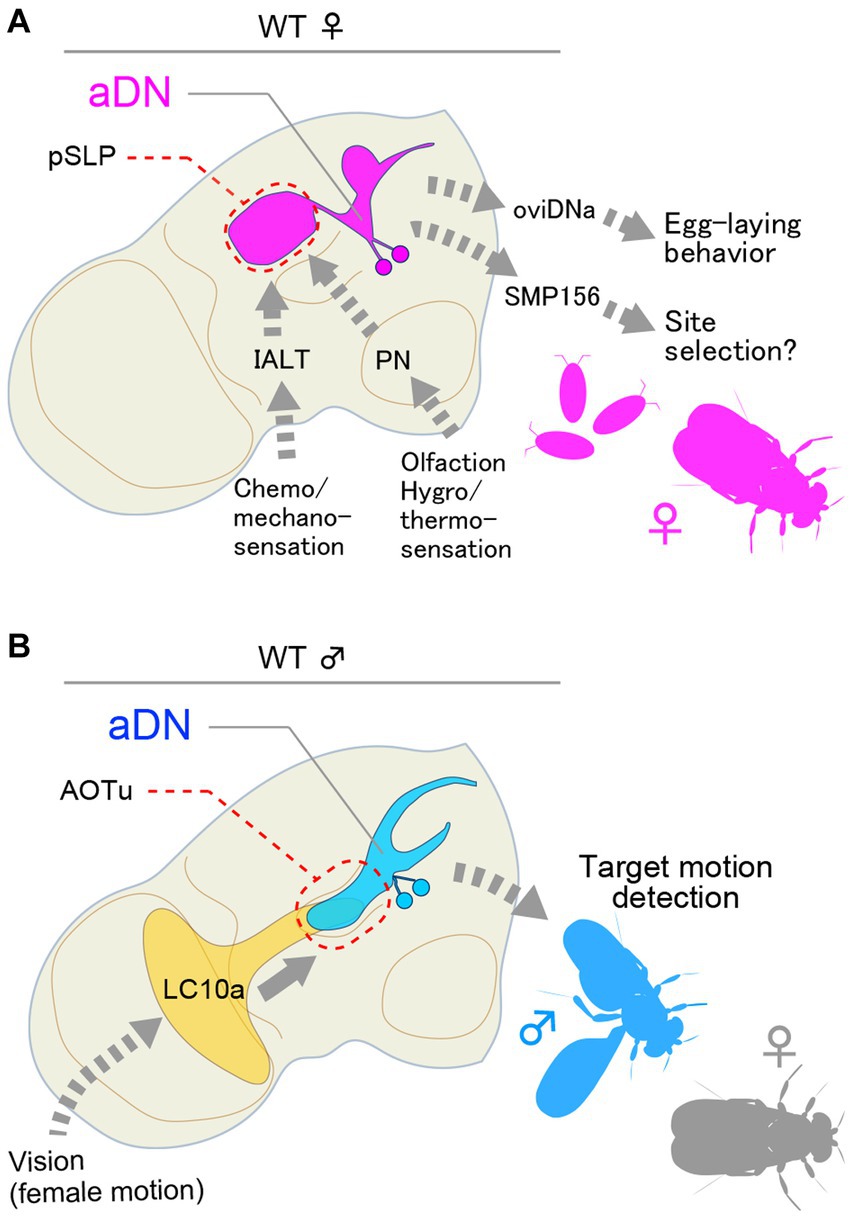
Figure 2. dsx-expressing aDN neurons receive sexually dimorphic input and generate distinct behavior in females and males. (A) Female aDN neurons receive multimodal sensory input in the posterior part of the superior lateral protocerebrum (pSLP), superior clamp (SCP), and ventrolateral protocerebrum (VLP) with predominating olfactory input from the lateral horn for the control of communal egg-laying behavior. (B) Male aDN neurons extend their neurite in the anterior optic tubercle (AOTu), where they receive visual input from LC10a visual projection neurons. These neurons regulate visual courtship pursuit toward a female. IALT, lateral antennal lobe tract.
Closing remarks
In the above sections, we examined three remarkable findings that illuminate specific cellular changes in the neural circuit that are causally related to sex differences in behavior. All cellular changes observed in these studies occurred cell autonomously within single neurons that express fru and/or dsx. Then, how do fru and dsx orchestrate such a major set of changes? Because the products of fru and dsx genes are transcription factors, the diversity in their functions likely derives from the diversity of their transcriptional targets (Luo et al., 2011; Ito et al., 2012; Clough et al., 2014; Neville et al., 2014; Ghosh et al., 2019; Li et al., 2022; Palmateer et al., 2023).
P1 neurons express both fru and dsx and are male-specific because female P1 homologs are fated to die as directed by the female-type dsx product DsxF (Kimura et al., 2008). However, females have a large set of P1-like neurons, PC1, which play central roles in aggression and receptivity control (Zhou et al., 2014; Deutsch et al., 2020; Chiu et al., 2021; Wang et al., 2021).
Some fru-single positive neuron clusters contain different numbers of neurons depending on the sex. For example, the mAL cluster is composed of 5 and 30 cells in the female and male brain, respectively (Kimura et al., 2005). This difference in cell number between the sexes arises from both sexually dimorphic proliferation and cell death, suggesting that dsx and possibly fru gene products regulate these fundamental cellular processes (Kimura et al., 2005; Ren et al., 2016; Ghosh et al., 2019).
We saw above that third-order processing of cVA information involves aSP-f in males and aSP-g in females, and the switching of synaptic partners is accomplished by the extension and retraction of dendritic branches by these neurons, which in turn depend on whether the neurons contain FruM (Kohl et al., 2013). In a similar manner, aDN switches its synaptic partners from/to olfactory vs. visual interneurons dependent on the presence or absence of DsxM (Nojima et al., 2021). In addition, P1 neurons extend a neurite in unusual directions in the male brain when FruM is lost from the P1 neurons themselves in the otherwise fru+ wild-type mosaic brain (Kimura et al., 2008). These observations collectively suggest that transforming dendritic branches at a hub in information flow is one of the major mechanisms for generating dimorphic circuits for sex-specific behavior by FruM and Dsx proteins. In fact, two firmly established transcriptional targets for FruM are two guidance molecules, i.e., robo1 and tei (Ito et al., 2016; Sato et al., 2020). Õzel et al. (2022) proposed that the identity of individual neurons determined early in life is implemented in mature neurons by a neuron-specific code (the terminal selector code; Hobert and Kratsios, 2019) made up of ~10 transcriptional factors, whose sustained expression maintains the identity throughout the postembryonic stage. They showed in the Drosophila optic lobe that gain or loss of just one transcription factor alters the code so that the manipulated neuron transforms into another type of neuron by changing, for example, its dendritic pattern. The terminal selector transcription factors directly regulate the transcription of genes for effector proteins, such as Dscam4 and the Netrin receptor frazzled (Õzel et al., 2022). Expression of FruM and Dsx is sustained throughout the adult stage (Lee et al., 2000; Usui-Aoki et al., 2000) and directly regulates the transcription of effector proteins including robo1 and tei in the case of FruM (Ito et al., 2016; Sato et al., 2020). It is thus tempting to hypothesize that FruM and Dsx contribute to terminal selector codes for sex-specific neuronal characteristics required for generating sex-typical behaviors. FruM and probably also Dsx form a large complex with several other transcription factors to remodel chromatin structures for orchestrating transcription of a large number of target genes to shape sex-specific neuronal structure and function (Ito et al., 2012, 2016; Neville et al., 2014; Vernes, 2014; Chowdhury et al., 2017; Zhang et al., 2018; Sato et al., 2019, 2020). A recent snRNA-seq analysis in the sexually dimorphic bed nucleus of stria terminalis of the mouse brain unraveled a similar genome-wide chromatin rearrangement triggered by estrogen receptor activation for sex-specific network patterning in the perinatal stage, which represents the organizational effect of sex steroids (Gegenhuber et al., 2022). Mammalian sex steroids also mediate the manifestation of secondary sex characteristics and sexual drive after adolescence, demonstrating the activational effect of sex steroids. A deep homology between mammalian and insect neural sex differentiation processes implies the existence of adult-specific effects of FruM and Dsx, in addition to their roles in development. Indeed, Chen et al. (2021) proposed that FruM expressed in adult neurons inhibits male-to-male courtship, while pupal FruM instructs the circuit formation for male-to-female courtship behavior, based on the distinctly different effects of fru knockdown at the adult vs. pupal stages. Notably, pheromone-sensitive Or47b-expressing olfactory neurons exhibit experience-dependent FruM expression and functional modulation at the adult stage (Hueston et al., 2016; Sethi et al., 2019). There exists evidence that the P1a subset of P1 neurons, which plays a critical role in male-to-female courtship, may be dispensable for inducing male–male courtship (Bonheur et al., 2023).
This review article primarily focused on sexually dimorphic shaping of synaptic connectivity and survival of specific neuron types via cell autonomous actions of putative terminal selector transcription factors. Attention must be paid, however, to the fact that non-cell autonomous actions of a terminal selector transcription factor also play pivotal roles in organizing sexually dimorphic circuits. Indeed, an array of neuropeptides have been shown to modulate outputs of the fru circuit depending on the behavioral context as paracrine or endocrine signals (Lee et al., 2006; Kim et al., 2013; Asahina et al., 2014; Chen et al., 2017). Of note, some of fru developmental roles may be mediated by cell non-autonomous actions via cell-to-cell interactions, which affect survival and morphogenesis of synaptic partners (Brovero et al., 2021). Although the fru- and/or dsx-expressing neurons that process the pheromone inputs were the major subject of this article, these transcription factors are vital for sex-specification of neurons in the motor pathways (e.g., premotor neurons involved in copulation control; Crickmore and Vosshall, 2013; Kim et al., 2013).
The principle of circuit rearrangements by a sex-specific terminal selector in development may apply to sex-type specification in the mammalian brain, in which sex steroids initiate the entire process.
Further studies are needed to validate the hypothesis that the sex-specific terminal selector code acts throughout life to accomplish innate and experience-dependent wiring for gendered behavior across the animal kingdom.
Author contributions
KS: Funding acquisition, Writing – original draft. DY: Conceptualization, Funding acquisition, Writing – original draft, Writing – review & editing.
Funding
The author(s) declare financial support was received for the research, authorship, and/or publication of this article. This work was supported, in part, by Grants-in-Aid for Scientific Research from the Ministry of Education, Culture, Sports, Science and Technology (MEXT) of Japan to DY (grant no. 21H04790) and KS (grant no. 22H02726), and a Life Science Grant from the Takeda Science Foundation to DY and KS.
Acknowledgments
We thank current and past members of the Yamamoto laboratory for contributions to the original researches and M. Adachi for secretarial assistance.
Conflict of interest
The authors declare that the research was conducted in the absence of any commercial or financial relationships that could be construed as a potential conflict of interest.
Publisher’s note
All claims expressed in this article are solely those of the authors and do not necessarily represent those of their affiliated organizations, or those of the publisher, the editors and the reviewers. Any product that may be evaluated in this article, or claim that may be made by its manufacturer, is not guaranteed or endorsed by the publisher.
References
Agrawal, S., Safarik, S., and Dickinson, M. (2014). The relative roles of vision and chemosensation in mate recognition of Drosophila melanogaster. J. Exp. Biol. 217, 2796–2805. doi: 10.1242/jeb.105817
Asahina, K. (2017). Neuromodulation and strategic action choice in Drosophila aggression. Annu. Rev. Neurosci. 40, 51–75. doi: 10.1146/annurev-neuro-072116-031240
Asahina, K., Watanabe, K., Duistermars, B. J., Hoopfer, E., González, C. R., Eyjólfsdóttir, E. A., et al. (2014). Tachykinin-expressing neurons control male-specific aggressive arousal in Drosophila. Cell 156, 221–235. doi: 10.1016/j.cell.2013.11.045
Bartelt, R. J., Schaner, A. M., and Jackson, L. L. (1985). cis-Vaccenyl acetate as an aggregation pheromone in Drosophila melanogaster. J. Chem. Ecol. 11, 1747–1756. doi: 10.1007/BF01012124
Bonheur, M., Swartz, K. J., Metcalf, M. G., Wen, X., Zhukovskaya, A., Mehta, A., et al. (2023). A rapid and bidirectional reporter of neural activity reveals neural correlates of social behaviors in Drosophila. Nat. Neurosci. 26, 1295–1307. doi: 10.1038/s41593-023-01357-w
Brieger, G., and Butterworth, F. M. (1970). Drosophila melanogaster: identity of male lipid in reproductive system. Science 167:1262. doi: 10.1126/science.167.3922.1262
Brovero, S. G., Fortier, J. C., Hu, H., Lovejoy, P. C., Newell, N. R., Palmateer, C. M., et al. (2021). Investigation of Drosophila fruitless neurons that express Dpr/DIP cell adhesion molecules. elife 10:e63101. doi: 10.7554/eLife.63101
Cachero, S., Ostrovsky, A. D., Yu, J. Y., Dickson, B. J., and Jefferis, G. S. X. E. (2010). Sexual dimorphism in the fly brain. Curr. Biol. 20, 1589–1601. doi: 10.1016/j.cub.2010.07.045
Chen, J., Jin, S., Chen, D., Cao, J., Ji, X., Peng, Q., et al. (2021). Fruitless tunes functional flexibility of courtship circuitry during development. elife 10:e59224. doi: 10.7554/eLife.59224
Chen, D., Sitaraman, D., Chen, N., Jin, X., Han, C., Chen, J., et al. (2017). Genetic and neuronal mechanisms governing the sex-specific interaction between sleep and sexual behaviors in Drosophila. Nat. Commun. 8:154. doi: 10.1038/s41467-017-00087-5
Chiu, H., Hoopfer, E. D., Coughlan, M. L., Pavlou, H. J., Goodwin, S. F., and Anderson, D. J. (2021). A circuit logic for sexually shared and dimorphic aggressive behaviors in Drosophila. Cell 184, 507–520.e16. doi: 10.1016/j.cell.2020.11.048
Chowdhury, Z. S., Sato, K., and Yamamoto, D. (2017). The core-promoter factor TRF2 mediates a fruitless action to masculinize neurobehavioral traits in Drosophila. Nat. Commun. 8:1480. doi: 10.1038/s41467-017-01623-z
Clough, E., Jimenez, E., Kim, Y. A., Whitworth, C., Neville, M. C., Hempel, L. U., et al. (2014). Sex- and tissue-specific functions of Drosophila Doublesex transcription factor target genes. Dev. Cell 31, 761–773. doi: 10.1016/j.devcel.2014.11.021
Clyne, J. D., and Miesenböck, G. (2008). Sex-specific control and tuning of the pattern generator for courtship song in Drosophila. Cell 133, 354–363. doi: 10.1016/j.cell.2008.01.050
Coen, P., and Murthy, M. (2016). Singing on the fly: sensorimotor integration and acoustic communication in Drosophila. Curr. Opin. Neurobiol. 38, 38–45. doi: 10.1016/j.conb.2016.01.013
Cortot, J., Farine, J. P., Cobb, M., Everaerts, C., and Ferveur, J.-F. (2022). Factors affecting the biosynthesis and emission of a Drosophila pheromone. J. Exp. Biol. 225:jeb244422. doi: 10.1242/jeb.244422
Crickmore, M. A., and Vosshall, L. B. (2013). Opposing dopaminergic and GABAergic neurons control the duration and persistence of copulation in Drosophila. Cell 155, 881–893. doi: 10.1016/j.cell.2013.09.055
Datta, S. R., Vasconcelos, M. L., Ruta, V., Luo, S., Wong, A., Demir, E., et al. (2008). The Drosophila pheromone cVA activates a sexually dimorphic neural circuit. Nature 452, 473–477. doi: 10.1038/nature06808
Demir, E., and Dickson, B. J. (2005). Fruitless splicing specifies male courtship behavior in Drosophila. Cell 121, 785–794. doi: 10.1016/j.cell.2005.04.027
Deutsch, D., Pacheco, D., Encarnacion-Rivera, L., Pereira, T., Fathy, R., Clemens, J., et al. (2020). The neural basis for a persistent internal state in Drosophila females. elife 9:e59502. doi: 10.7554/eLife.59502
Dorkenwald, S., CE, M. K., Macrina, T., Kemnitz, N., Lee, K., Lu, R., et al. (2021). FlyWire: online community for whole-brain connectomics. Nat. Methods 19, 119–128. doi: 10.1038/s41592-021-01330-0
Ellendersen, B. E., and von Philipsborn, A. C. (2017). Neuronal modulation of D. melanogaster sexual behaviour. Curr. Opin. Insect. Sci. 24, 21–28. doi: 10.1016/j.cois.2017.08.005
Gegenhuber, B., Wu, M. V., Bronstein, R., and Tollkuhn, J. (2022). Gene regulation by gonadal hormone receptors underlies brain sex differences. Nature 606, 153–159. doi: 10.1038/s41586-022-04686-1
Ghosh, N., Bakshi, A., Khandelwal, R., Rajan, S. G., and Joshi, R. (2019). The Hox gene abdominal-B uses DoublesexF as a cofactor to promote neuroblast apoptosis in the Drosophila central nervous system. Development 146:dev175158. doi: 10.1242/dev.175158
Guiraudie-Capraz, G., Pho, D. B., and Jallon, J.-M. (2007). Role of the ejaculatory bulb in biosynthesis of the male pheromone cis-vaccenyl acetate in Drosophila melanogaster. Integr. Zool. 2, 89–99. doi: 10.1111/j.1749-4877.2007.00047.x
Hobert, O., and Kratsios, P. (2019). Neuronal identity control by terminal selectors in worms, flies, and chordates. Curr. Opin. Neurobiol. 56, 97–105. doi: 10.1016/j.conb.2018.12.006
Hueston, C. E., Olsen, D., Li, Q., Okuwa, S., Peng, B., Wu, J., et al. (2016). Chromatin modulatory proteins and olfactory receptor signaling in the refinement and maintenance of fruitless expression in olfactory receptor neurons. PLoS Biol. 14:e1002443. doi: 10.1371/journal.pbio.1002443
Inagaki, H. K., Jung, Y., Hoopfer, E. D., Wong, A. M., Mishra, N., Lin, J. Y., et al. (2014). Optogenetic control of Drosophila using a red-shifted channelrhodopsin reveals experience-dependent influences on courtship. Nat. Methods 11, 325–332. doi: 10.1038/nmeth.2765
Ito, H., Sato, K., Koganezawa, M., Ote, M., Matsumoto, K., Hama, C., et al. (2012). Fruitless recruits two antagonistic chromatin factors to establish single-neuron sexual dimorphism. Cell 149, 1327–1338. doi: 10.1016/j.cell.2012.04.025
Ito, H., Sato, K., Kondo, S., Ueda, R., and Yamamoto, D. (2016). Fruitless represses robo1 transcription to shape male-specific neural morphology and behavior in Drosophila. Curr. Biol. 26, 1532–1542. doi: 10.1016/j.cub.2016.04.067
Jaeger, A. H., Stanley, M., Weiss, Z. F., Musso, P. Y., Chan, R. C., Zhang, H., et al. (2018). A complex peripheral code for salt taste in Drosophila. elife 7:e37167. doi: 10.7554/eLife.37167
Jiang, X., and Pan, Y. (2022). Neural control of action selection among innate behaviors. Neurosci. Bull. 38, 1541–1558. doi: 10.1007/s12264-022-00886-x
Kim, W. J., Jan, L. Y., and Jan, Y. N. (2013). A PDF/NPF neuropeptide signaling circuitry of male Drosophila melanogaster controls rival-induced prolonged mating. Neuron 80, 1190–1205. doi: 10.1016/j.neuron.2013.09.034
Kimura, K.-i., Hachiya, T., Koganezawa, M., Tazawa, T., and Yamamoto, D. (2008). Fruitless and Doublesex coordinate to generate male-specific neurons that can initiate courtship. Neuron 59, 759–769. doi: 10.1016/j.neuron.2008.06.007
Kimura, K.-i., Ote, M., Tazawa, T., and Yamamoto, D. (2005). Fruitless specifies sexually dimorphic neural circuitry in the Drosophila brain. Nature 438, 229–233. doi: 10.1038/nature04229
Koganezawa, M., Kimura, K.-i., and Yamamoto, D. (2016). The neural circuitry that functions as a switch for courtship versus aggression in Drosophila males. Curr. Biol. 26, 1395–1403. doi: 10.1016/j.cub.2016.04.017
Kohatsu, S., Koganezawa, M., and Yamamoto, D. (2011). Female contact activates male-specific interneurons that trigger stereotypic courtship behavior in a Drosophila male. Neuron 69, 498–508. doi: 10.1016/j.neuron.2010.12.017
Kohatsu, S., and Yamamoto, D. (2015). Visually induced initiation of Drosophila innnate courtship-like following pursuit is mediated by central excitatory state. Nat. Commun. 6:6457. doi: 10.1038/ncomms7457
Kohl, J., Ostrovsky, A. D., Frechter, S., and Jefferis, G. S. X. E. (2013). A bidirectional circuit switch reroutes pheromone signals in male and female brains. Cell 155, 1610–1623. doi: 10.1016/j.cell.2013.11.025
Kondoh, Y., Kaneshiro, K. H., Kimura, K.-i., and Yamamoto, D. (2003). Evolution of sexual dimorphism in the olfactory brain of Hawaiian Drosophila. Proc. Biol. Sci. 270, 1005–1013. doi: 10.1098/rspb.2003.2331
Lee, G., Bahn, J. H., and Park, J. H. (2006). Sex-and clock-controlled expression of the neuropeptide F gene in Drosophila. Proc. Natl. Acad. Sci. U. S. A. 103, 12580–12585. doi: 10.1073/pnas.0601171103
Lee, G., Foss, M., Goodwin, S. F., Carlo, T., Taylor, B. J., and Hall, J. C. (2000). Spatial, temporal, and sexually dimorphic expression patterns of the fruitless gene in the Drosophila central nervous system. J. Neurobiol. 43, 404–426. doi: 10.1002/1097-4695(20000615)43:4<404::aid-neu8>3.0.co;2-d
Li, H., Janssens, J., de Waegeneer, M., Kolluru, S. S., Davie, K., Gardeux, V., et al. (2022). Fly cell atlas: a single-nucleus transcriptomic atlas of the adult fruit fly. Science 375:eabk2432. doi: 10.1126/science.abk2432
Luo, S. D., Shi, G. W., and Baker, B. S. (2011). Direct targets of the D. melanogaster DSXF protein and the evolution of sexual development. Development 138, 2761–2771. doi: 10.1242/dev.065227
Neville, M. C., Eastwood, A., Allen, A. M., de Haan, A., Nojima, T., and Goodwin, S. F. (2021). Generation and characterization of fruitless P1 promoter mutant in Drosophila melanogaster. J. Neurogenet. 35, 285–294. doi: 10.1080/01677063.2021.1931179
Neville, M. C., Nojima, T., Ashley, E., Parker, D. J., Walker, J., Southall, T., et al. (2014). Male-specific fruitless isoforms target neurodevelopmental genes to specify a sexually dimorphic nervous system. Curr. Biol. 24, 229–241. doi: 10.1016/j.cub.2013.11.035
Nojima, T., Rings, A., Allen, A. M., Otto, N., Verschut, T. A., Billeter, J.-C., et al. (2021). A sex-specific switch between visual and olfactory inputs underlies adaptive sex differences in behavior. Curr. Biol. 31, 1175–1191.e6. doi: 10.1016/j.cub.2020.12.047
Õzel, M. N., Gibbs, C. S., Holguera, I., Soliman, M., Bonneau, R., and Desplan, C. (2022). Coordinated control of neuronal differentiation and wiring by sustained transcription factors. Science 378:eadd1884. doi: 10.1126/science.add1884
Palmateer, C. M., Artikis, C., Brovero, S. G., Friedman, B., Gresham, A., and Arbeitman, M. N. (2023). Single-cell transcriptome profiles of Drosophila fruitless-expressing neurons from both sexes. elife 12:e78511. doi: 10.7554/eLife.78511
Pan, Y., Meissner, G. W., and Baker, B. S. (2012). Joint control of Drosophila male courtship behavior by motion cues and activation of male-specific P1 neurons. Proc. Natl. Acad. Sci. U. S. A. 109, 10065–10070. doi: 10.1073/pnas.1207107109
Price, P. D., Parkus, S. M., and Wright, A. E. (2023). Recent progress in understanding the genomic architecture of sexual conflict. Curr. Opin. Genet. Dev. 80:102047. doi: 10.1016/j.gde.2023.102047
Ren, Q., Awasaki, T., Huang, Y. F., Liu, Z., and Lee, T. (2016). Cell class-lineage analysis reveals sexually dimorphic lineage compositions in the Drosophila brain. Curr. Biol. 26, 2583–2593. doi: 10.1016/j.cub.2016.07.086
Ribeiro, I. M. A., Drews, M., Bahl, A., Machacek, C., Borst, A., and Dickson, B. J. (2018). Visual projection neurons mediating directed courtship in Drosophila. Cell 174, 607–621.e18. doi: 10.1016/j.cell.2018.06.020
Rideout, E. J., Dornan, A. J., Neville, M. C., Eadie, S., and Goodwin, S. F. (2010). Control of sexual differentiation and behavior by the doublesex gene in Drosophila melanogaster. Nat. Neurosci. 13, 458–466. doi: 10.1038/nn.2515
Ruta, V., Datta, S. R., Vasconcelos, M. L., Freeland, J., Looger, L. L., and Axel, R. (2010). A dimorphic pheromone circuit in Drosophila from sensory input to descending output. Nature 468, 686–690. doi: 10.1038/nature09554
Sato, K., Ito, H., and Yamamoto, D. (2020). Teiresias, a fruitless target gene encoding an immunoglobulin-superfamily transmembrane protein, is required for neuronal feminization in Drosophila. Commun. Biol. 3:598. doi: 10.1038/s42003-020-01327-z
Sato, K., Ito, H., Yokoyama, A., Toba, G., and Yamamoto, D. (2019). Partial proteasomal degradation of Lola triggers the male-to-female switch of a dimorphic courtship circuit. Nat. Commun. 10:166. doi: 10.1038/s41467-018-08146-1
Sato, K., and Yamamoto, D. (2022). Mutually exclusive expression of sex-specific and non-sex-specific fruitless gene products in the Drosophila central nervous system. Gene Expr. Patterns 43:119232. doi: 10.1016/j.gep.2022.119232
Scheffer, L. K., Xu, C. S., Januszewski, M., Lu, Z., Takemura, S. Y., Hayworth, K. J., et al. (2020). A connectome and analysis of the adult Drosophila central brain. elife 9:e57443. doi: 10.7554/eLife.57443
Sethi, S., Lin, H. H., Shepherd, A. K., Volkan, P. C., Su, C. Y., and Wang, J. W. (2019). Social context enhances hormonal modulation of pheromone detection in Drosophila. Curr. Biol. 29, 3887–3898.e4. doi: 10.1016/j.cub.2019.09.045
Shadmehr, R., Smith, M. A., and Krakauer, J. W. (2010). Error correction, sensory prediction, and adaptation in motor control. Annu. Rev. Neurosci. 33, 89–108. doi: 10.1146/annurev-neuro-060909-153135
Siegel, R. W., and Hall, J. C. (1979). Conditioned responses in courtship behavior of normal and mutant Drosophila. Proc. Natl. Acad. Sci. U. S. A. 76, 3430–3434. doi: 10.1073/pnas.76.7.3430
Siwicki, K. K., and Kravitz, E. A. (2009). Fruitless, Doublesex and the genetics of social behavior in Drosophila melanogaster. Curr. Opin. Neurobiol. 19, 200–206. doi: 10.1016/j.conb.2009.04.001
Stockinger, P., Kvitsiani, D., Rotkopf, S., Tirián, L., and Dickson, B. J. (2005). Neural circuitry that governs Drosophila male courtship behavior. Cell 121, 795–807. doi: 10.1016/j.cell.2005.04.026
Taisz, I., Donà, E., Münch, D., Bailey, S. N., Morris, B. J., Meechan, K. I., et al. (2023). Generating parallel representations of position and identity in the olfactory system. Cell 186, 2556–2573.e22. doi: 10.1016/j.cell.2023.04.038
Tosto, N. M., Beasley, E. R., Wong, B. B. M., Mank, J. E., and Flanagan, S. P. (2023). The roles of sexual selection and sexual conflict in shaping patterns of genome and transcriptome variation. Nat. Ecol. Evol. 7, 981–993. doi: 10.1038/s41559-023-02019-7
Usui-Aoki, K., Ito, H., Ui-Tei, K., Takahashi, K., Lukacsovich, T., Awano, W., et al. (2000). Formation of the male-specific muscle in female Drosophila by ectopic fruitless expression. Nat. Cell Biol. 2, 500–506. doi: 10.1038/35019537
Vernes, S. C. (2014). Genome wide identification of fruitless targets suggests a role in upregulating genes important for neural circuit formation. Sci. Rep. 4:4412. doi: 10.1038/srep04412
Wang, K., Wang, F., Forknall, N., Yang, T., Patrick, C., Parekh, R., et al. (2021). Neural circuit mechanisms of sexual receptivity in Drosophila females. Nature 589, 577–581. doi: 10.1038/s41586-020-2972-7
Yamamoto, D., and Koganezawa, M. (2013). Genes and circuits of courtship behaviour in Drosophila males. Nat. Rev. Neurosci. 14, 681–692. doi: 10.1038/nrn3567
Yu, J. Y., Kanai, M. I., Demir, E., Jefferis, G. S., and Dickson, B. J. (2010). Cellular organization of the neural circuit that drives Drosophila courtship behavior. Curr. Biol. 20, 1602–1614. doi: 10.1016/j.cub.2010.08.025
Zhang, B., Sato, K., and Yamamoto, D. (2018). Ecdysone signaling regulates specification of neurons with a male-specific neurite in Drosophila. Biol. Open 7:bio029744. doi: 10.1242/bio.029744
Keywords: Drosophila, fruitless, doublesex, terminal selectors, neural sexual dimorphism
Citation: Sato K and Yamamoto D (2023) Molecular and cellular origins of behavioral sex differences: a tiny little fly tells a lot. Front. Mol. Neurosci. 16:1284367. doi: 10.3389/fnmol.2023.1284367
Edited by:
Youngseok Lee, Kookmin University, Republic of KoreaReviewed by:
Woo Jae Kim, Harbin Institute of Technology, ChinaSubhabrata Sanyal, California Life Company (Calico), United States
Copyright © 2023 Sato and Yamamoto. This is an open-access article distributed under the terms of the Creative Commons Attribution License (CC BY). The use, distribution or reproduction in other forums is permitted, provided the original author(s) and the copyright owner(s) are credited and that the original publication in this journal is cited, in accordance with accepted academic practice. No use, distribution or reproduction is permitted which does not comply with these terms.
*Correspondence: Daisuke Yamamoto, daichan@nict.go.jp; Kosei Sato, kosei@nict.go.jp