- 1Key Laboratory of Eco-Environments in Three Gorges Reservoir Region, Engineering Research Center of South Upland Agriculture, Ministry of Education, College of Agronomy and Biotechnology, Southwest University, Chongqing, China
- 2Department of Agronomy, University of Agriculture Faisalabad, Faisalabad, Pakistan
Plants face a combination of different abiotic stresses under field conditions which are lethal to plant growth and production. Simultaneous occurrence of chilling and drought stresses in plants due to the drastic and rapid global climate changes, can alter the morphological, physiological and molecular responses. Both these stresses adversely affect the plant growth and yields due to physical damages, physiological and biochemical disruptions, and molecular changes. In general, the co-occurrence of chilling and drought combination is even worse for crop production rather than an individual stress condition. Plants attain various common and different physiological and molecular protective approaches for tolerance under chilling and drought stresses. Nevertheless, plant responses to a combination of chilling and drought stresses are unique from those to individual stress. In the present review, we summarized the recent evidence on plant responses to chilling and drought stresses on shared as well as unique basis and tried to find a common thread potentially underlying these responses. We addressed the possible cross talk between plant responses to these stresses and discussed the potential management strategies for regulating the mechanisms of plant tolerance to drought and/or chilling stresses. To date, various novel approaches have been tested in minimizing the negative effects of combine stresses. Despite of the main improvements there is still a big room for improvement in combination of drought and chilling tolerance. Thus, future researches particularly using biotechnological and molecular approaches should be carried out to develop genetically engineered plants with enhanced tolerance against these stress factors.
Introduction
Crops grown under open environments often pass through periods of abiotic stress during their life cycle. Such stresses many a time overlap so that the crop growth and productivity of the crops is adversely affected. Plants pass through a series of morphological, physiological, biochemical, and molecular changes in a quest to mitigate such adversities of the abiotic stresses. A lot of work reports the adaptive responses of crops plants to different abiotic stresses wherein emphasis has been laid on individual stress factors (e.g., Jongdee et al., 2002; Chinnusamy et al., 2007; Nejad et al., 2010; Basu et al., 2016; Wang et al., 2016; Anjum et al., 2017). Nonetheless, understanding the plant responses to combined stress factors is inevitable for enhancing the adaptation of plants under field conditions (Pandey et al., 2015). Crops experience periods of extreme low temperatures in many regions of the world (Ruelland et al., 2009; Wang et al., 2016), and are exposed to limited water availability owing to either drought or disturbed water movement and uptake under low ambient temperatures (Shinozaki et al., 2003; Zhang J.Z. et al., 2004; Beck et al., 2007). Such an exposure of plants to chilling and drought simultaneously hampers plant growth, and hence is detrimental to productivity (Tommasini et al., 2008). Studies show that primarily chilling and drought pose a similar impact on stomatal development and leaf growth, nevertheless, the mechanisms of drought-induced changes in some physiological processes are quite different than those induced by chilling (Deng et al., 2012). A little is known about the combined effect of chilling and drought stresses on plants, and whether or not plant responses to these are unique or shared is also unclear. Plants may exhibit common molecular and physiological responses on exposure chilling and drought (shared response), others may be specific to a given stress factor (Atkinson et al., 2013; Sewelam et al., 2014). In general, chilling stress thermodynamically declines the kinetics of several physiological as well as metabolic processes occurring in plants (Ruelland et al., 2009; Hussain et al., 2016). It severely reduces the rate and uniformity of germination, hampers seedling vigor, and delays ontogenic plant development (Cruz and Milach, 2004; Oliver et al., 2007), resulting in severe crop yield losses (Ruelland et al., 2009).
Drought stress in plants is characterized by reduced leaf water potential and turgor pressure, stomatal closure, and decreased cell growth and enlargement (Farooq et al., 2009b). Drought stress reduces the plant growth by influencing various physiological as well as biochemical functions such as photosynthesis, chlorophyll synthesis, nutrient metabolism, ion uptake and translocation, respiration, and carbohydrates metabolism (Jaleel et al., 2008; Farooq et al., 2009b; Li et al., 2011). Nevertheless, plants experience water deficit not only during drought, but low temperature may also cause turgor stress at cellular level (Thomashow, 1994; Janska et al., 2009; Yadav, 2010) owing to poor root hydraulic conductance and diminished root activity (Aroca et al., 2003).
Oxidative stress is crucial in relation to chilling- and drought-induced injuries in plants (Srivalli et al., 2003; Hussain et al., 2016). Drought and chilling stress exacerbates ROS production in plants’ cell. Excess production and the accumulation of ROS causes oxidative damage at cellular level, disrupts cellular membranes, and leads to enzyme inactivation, protein degradation, and ionic imbalance in plants (Baier et al., 2005; Tarchoune et al., 2010). The ROS disturb the cellular macromolecules including DNA, and hence may cause deletion of bases due to alkylation and oxidation which are linked with various physiological and biochemical disorders in plants (Tuteja and Tuteja, 2001). Plants possess a highly efficient and sophisticated antioxidative defense system to control the overproduction of ROS (Hussain et al., 2016). The ROS-induced damages and disruption of cellular homeostasis are alleviated by the action of different enzymatic (e.g., catalase, CAT; superoxide dismutase, SOD; peroxidase, POD; glutathione reductase, GR; glutathione peroxidase, GPX) and non-enzymatic (e.g., ascorbic acid, carotenoids, α-tocopherol, and glutathione content) antioxidants (Gill and Tuteja, 2010; Chen et al., 2016; Hussain et al., 2016). In plants, the levels of ROS are regulated by their production rate as well as the extent of their detoxification by enzymatic and/or non-enzymatic antioxidant systems. Mechanism of ROS production and its scavenging by high antioxidative capacity has been associated with tolerance of plants to abiotic stresses (Gill and Tuteja, 2010).
Studies involving chilling and drought as absolute stress factor have been well documented for several crops. Nevertheless, little focus has been laid on their combined effect, and plant response to both of these. Some recent studies ponder that the physiological and molecular response of the crops to combination of two different environmental stresses is unique so that such responses cannot be directly extrapolated when looked in the context of any of these stress applied individually (Mittler, 2006). Based on the available work on the effect of multiple stresses on crop plants, an effort is made to comprehend the current understanding of chilling and drought stress on crop plants. We have tried to give a general overview of shared and unique responses of crop plants to drought and chilling stresses and discuss some possible mitigation strategies to cope with these stresses for minimizing their damage on crop plants.
Plant Responses to Chilling and Drought Stresses
Water scarcity and suboptimal temperature at any growth stage of crop plants evoke negative effects on crop growth and development. Such detrimental effects on plants depend on the magnitude of stress and its period and the plant growth stage. Such effects are elicited in terms of morphological, physiological, biochemical, and molecular processes in plants.
Morphological and Yield Responses
Germination
Some field crops are extremely sensitive to chilling and drought particularly during germination and early phases of seedling development. Each seed requires optimum temperature and soil moisture for germination. Chilling stress severely impairs the germination and often lowers the seedling vigor (Kang and Saltveit, 2002; Wang et al., 2016), delays plant development and ultimately causes severe yield losses (Cruz and Milach, 2004; Oliver et al., 2007; Ruelland et al., 2009). Chilling stress is known to thermodynamically limit the kinetics of various physiological as well as metabolic functions in plants (Ruelland et al., 2009). Chilling stress evokes shoot water deficit due to its thermophilic nature and reduced root water uptake (Stewart et al., 1990).
Drought stress impairs germination by limiting water imbibition, and reduces seedling vigor (Kaya et al., 2006; Farooq et al., 2009b). Both chilling stress and limited water supply results in disturbance of osmotic balance, impaired metabolic activity at cellular level and excessive ROS production leading to alterations in DNA, RNA and protein structures, membrane damage, reduced respiration and less ATP production (Priestley, 1986) ultimately causing loss in seed germination and vigor.
Growth
Both chilling and drought stress are known to cause significant reductions in plant growth and development. Various studies have reported the negative effects of chilling stress on growth of rice (Hussain et al., 2016; Wang et al., 2016), cotton (Zhao et al., 2012), tomato (Starck et al., 2000), potato (Svensson et al., 2002), muskmelons (Wang et al., 2004), and sugarcane (Thakur et al., 2010; Anjum et al., 2011; Zhu et al., 2013). Likewise, drought stress has also been reported to reduce plant height, leaf area, stem diameter, and plant biomass in different field crops (Apel and Hirt, 2004; Farooq et al., 2009b; Zheng et al., 2016). Our recent investigation on maize (Hussain et al., unpublished) has revealed that chilling as well as drought stress severely damaged the shoot and root growth attributes, compared with control. The interactive damage caused by chilling and drought was found to be additive for all growth attributes.
Chilling stress may cause necrotic lesions on leaves, delay leaf development, prolong cell cycle with decreased cell production, induce wilting, and increase susceptibility to pathogens and diseases (Korkmaz and Dufault, 2001; Rymen et al., 2007). Decreased rate of both cell division and elongation causes smaller leaf area (Ben-Haj-Salah and Tardieu, 1995; Jouyban et al., 2013), while slow leaf initiation rate leads to reduced number of leaves under periods of chilling stress (Warrington and Kanemasu, 1983; Lukatkin et al., 2012; Jouyban et al., 2013). Under drought stress, leaf growth is mainly restricted by decreased leaf water potential (Hsiao and Xu, 2000). Whilst, interrupted water flow from xylem to other elongating cells and reduced turgor pressure under severe drought stress, leads to reduced cell elongation and smaller leaf area in crop plants (Nonami, 1998; Schuppler et al., 1998; Taiz and Zeiger, 2006).
Chilling stress restricts root growth by decreasing root length, biomass, and morphology (Cutforth et al., 1986), which ultimately reduces volume of the root system for exploring the nutrients and water (Richner et al., 1996). Nevertheless, the reported effects of drought stress on root growth have been diverse. For instance, drought stress increased the root length of Catharanthus roseus (Jaleel et al., 2008) and sunflower (Tahir et al., 2002), but did not affect the root growth of wheat and maize (Sacks et al., 1997). Increase in root length under drought conditions have been attributed to increased abscisic acid content in roots (Manivannan et al., 2007). Wullschleger et al. (2005) stated that root dry biomass of Populus species was reduced under mild and severe drought stress. In general, varieties having characters of deep and prolific root system were better able to sustain growth and yield under water-deficit conditions (Babu et al., 2001).
Yield and Yield Related Attributes
Water deficit as well as sub-optimal temperatures reduce the growth and metabolic activities leading to reduction in agronomic and yield attributes of the crops. Rizza et al. (1994) reported that drought and chilling stress severely impaired the barley productivity. The chilling stress in chickpea at reproductive stage led to flower abortion and poor pod setting (Clarke and Siddique, 2004; Nayyar et al., 2005). In temperate growing areas, cold temperature is responsible for 30–40% reduction in rice yields because chilling stress resulted in degeneration of spikelets, panicle deformation and poor spikelet fertility (Andaya and Mackill, 2003). In grain crops, low temperature stress during reproductive development may induce flower abscission, ovule abortion, pollen sterility, pollen tube distortion, poor fruit set, and hence reduced final yields (Thakur et al., 2010). Whaley et al. (2004) found that chilling stress during stem elongation reduced the internode extension, caused spikelet death, and lowered biomass accumulation and grain yield in wheat. Low temperature at jointing and booting stages of wheat significantly decreased the number of productive tillers per plant (Li et al., 2015), and negatively affected the development of young spikes (Thakur et al., 2010).
Drought–induced decrease in yield and yield components of maize (Kamara et al., 2003), wheat (Barnabás et al., 2008), sugarcane (Vasantha et al., 2005), sunflower (Tahir et al., 2002), peanut (Furlan et al., 2012), and cotton (Pettigrew, 2004) has also been reported. In legumes, drought can dramatically reduce the seed yield by limiting flower and pod production, increasing flower and pod abortion, and reducing seed size (Davies et al., 1999; Fang et al., 2010). It has been estimated that drought stress may decrease the global chickpea production by 33% (Kashiwagi et al., 2015). In soybean, drought stress significantly reduced the number of branches and total seed yield (Frederick et al., 2001).
Conclusively, both drought and chilling temperature adversely affect yield and yield components of crop plants. Reductions in these traits could be the outcome of stress-induced modifications in metabolic and physiological activities and corresponding negative implication for plant reproductive organs with reduced productivity. Overall, onset of either drought or chilling is injurious to plants, nevertheless, plant reproductive stage is perhaps the most sensitive stage to these stress factors than pre- and post-reproductive stages.
Physiological and Biochemical Responses
Photosynthesis and Gaseous Exchange
Drought and chilling stresses alter the normal rates of photosynthesis and other gas exchange attributes in crop plants. While working on sugarcane, Sales et al., (2013) found that drought stress alone or in combination with chilling stress severely affected the leaf gas exchange, photochemical activity, CO2 assimilation, generation of energetic pressure at the PSII level, electron transport rate and transpiration. Under chilling stress, low photosynthetic rates in crop plants have been attributed owing to poor stomatal and mesophyll conductance of CO2, impaired chloroplastic development, restricted metabolite transport, decreased quantum efficiency and the quantum yield for CO2 assimilation (Nie et al., 1995; Haldimann, 1998; Sowiński et al., 2005). Jung and Steffen (1997) argued that chilling stress reduces photosynthetic efficiencies and enhances photo-inhibition process due to over excitation of thylakoid membranes and subsequent impairment of photosynthetic function. Moreover, chilling slows down the rate of CO2 fixation and restricts the NADP+ supplement available to accept electrons from the electron transport chain (Wise, 1995). On the other hand, drought stress reduces photosynthesis by inhibiting leaf area and decreasing the rate of photosynthesis per unit leaf area (Wahid and Rasul, 2005; Farooq et al., 2009b). Drought stress slows down carbon fixation rate by directly restricting metabolism or by limiting the CO2 entry into the leaf (Apel and Hirt, 2004; Farooq et al., 2009b). Imbalance between capturing and utilization of light (Foyer and Noctor, 2000), decrease in Rubisco activity (Bota et al., 2004), alterations in the photosynthetic pigments (Anjum et al., 2003), and damage to photosynthetic apparatus (Fu and Huang, 2001) are some of the major reasons for drought-induced reductions in photosynthesis. However, the drought-induced limitations of photosynthesis through metabolic distortions are more complex than stomatal limitations, and these mainly occur through reduced synthesis of photosynthetic pigments (Reddy et al., 2004). During drought stress, limited intercellular CO2 concentration leads to the accumulation of reduced photosynthetic electron transport components that can potentially reduce the molecular oxygen, resulting in the production of ROS, which are deleterious to photosynthetic apparatus (Basu et al., 2016). Although, chilling and drought stresses have some unique effects on photosynthetic functions of plants, yet both these stresses are detrimental for plant photosynthetic apparatus and light harvesting mechanism.
Water Relations
Relative water content, leaf water potential, transpiration rate, stomatal conductance, and leaf and canopy temperature are the important attributes describing plant water relations (Farooq et al., 2009a). Sales et al. (2013) reported severe reductions in leaf water potential of sugarcane under the simultaneous occurrence of cold and drought stresses, compared with individual stress factor. Generally, the chilling-sensitive plants exhibit water stress symptoms under low temperatures, which are triggered by reduced root hydraulic conductivity followed by severe decline in leaf water potential and loss of turgor pressure (Aroca et al., 2003). McWilliam et al. (1982) and Lee et al. (1993) also reported that decline in root hydraulic conductivity and loss of stomatal control caused chilling-induced wilting in plants. Aroca et al. (2003) stated that low temperature-induced reduction in vapor pressure deficit between the leaf surface and the atmosphere decreased transpiration rate and root water uptake drastically. Chilling stress caused shoot water deficits in maize plants by significant reductions in root water uptake instead of leaf transpiration (Janowiak and Markowski, 1994). Likewise, Javier et al. (1997) reported that under chilling stress, root water uptake decreased in drought hardened plants similar to non-drought hardened plants, while transpiration rate was lower in drought hardened plants only.
Exposure of drought stress has also been known to substantially decrease the relative water content, turgor pressure, leaf water potential, and transpiration rate in a number of plant species (Siddique et al., 2001; Tezara et al., 2002; Nayyar and Gupta, 2006; Campos et al., 2011). Liu et al. (2004) reported that drought stress significantly declined the water potential of soybean roots, leaves, and pods, compared with control. Likewise, Reddy et al. (2004) observed that tissue water contents were decreased linearly with increase in drought severity. In short, chilling as well as drought stress cause disturbances in plant water relations; nevertheless such effects are likely to be more severe under the combination of both of these factors.
Nutrient Relations
In addition to reduced plant growth and productivity (Tommasini et al., 2008), the nutrient uptake behavior of plants is also impaired under chilling (Xu and Huang, 2006) and drought stress (Hu et al., 2007). Temperature affects the physio-chemical and microbial processes in soils which may modify the plant-nutrient relationships (Yan et al., 2012). Poor root system of plants under chilling stress reduces the uptake of several nutrients, including N, P, and K (Pan et al., 1985; Steffens, 1986; Domisch et al., 2002; Yan et al., 2012). Under chilling stress, shoot growth of maize seedlings was reduced by the direct effect of low temperature on shoot meristems and by restricted supply of nutrients via roots (Hund et al., 2007). Farooq et al. (2009a) concluded that reduced root length, low hydraulic conductance, poor root branching, and thicker root axis under chilling stress lead to reduced mineral nutrient uptake in plants. However, the variations in such effects may arise based on plant species, stress period, physiological plant growth stage, or the frequency of changing the nutrient solutions.
Drought stress also decreases the availability, uptake, transfer and metabolism of nutrients (Farooq et al., 2009b). Exposure of drought stress in plants generally decreases both the uptake of nutrients by roots and translocation from roots to shoots (Hu and Schmidhalter, 2005). Drought-induced reductions in uptake and translocation of macro-nutrients (N, P, and K) have been reported in various plant species (Kuchenbuch et al., 1986; Subramanian et al., 2006; Asrar and Elhindi, 2011; Suriyagoda et al., 2014). Low soil moisture availability under drought stress reduced the root growth and the rate of nutrient inflow in terms of both per unit of root length and root biomass (Kuchenbuch et al., 1986). Moreover, drought stress causes the difference in active transport and membrane permeability of cations (K+, Ca2+, and Mg2+), thus resulting in decreased absorption of these cations via roots (Hu and Schmidhalter, 2005; Farooq et al., 2009b). Drought stress limits the activities of enzymes involved in nutrient assimilation. For instance, the nitrate reductase activities in the leaves and nodules of dhainicha (Sesbania aculeata L.) and common bean (Phaseolus vulgaris L.) were significantly decreased under drought stress (Ashraf and Iram, 2005). Apart from macro-nutrient, drought can induce the deficiencies of some micro nutrients, i.e., Mn, Fe, and Mo (Hu and Schmidhalter, 2005) whereas these micronutrients become increasingly available under well-watered conditions due to their conversion to more soluble and reduced forms (Havlin et al., 1999). In summary; there exists a significant interaction among the nutrient acquisition, soil moisture content and soil temperature. Both chilling and drought stresses decreases the availability, uptake, translocation and metabolism of nutrients in plants. Most of the previous reports have focused on the nutrient relation with individual stress, while rare studies have examined the nutrient acquisition in plants under combine stress factors. Therefore, quantification of the effects of combined chilling and drought stresses on the nutrient uptake, transport, and assimilation need to be investigated further.
Oxidative Status
Imbalance between generation and safe detoxification of ROS represents metabolic state that is referred to as oxidative stress (Baier et al., 2005). Excess ROS accumulation causes protein oxidation, membrane lipid peroxidation, DNA and RNA damage, and may even lead to cell death (Mittler, 2002; Apel and Hirt, 2004). Hydrogen peroxide (H2O2), singlet oxygen (1O2), superoxide radicals (O2-), and hydroxyl radicals (OH-) are the major ROS produced in plants under unfavorable environmental conditions (Apel and Hirt, 2004). Generally, the production of ROS is linear with the severity of stress conditions.
Chilling and drought stresses cause oxidative damage by overproduction of ROS in plant cells (Xing and Rajashekar, 2001; Guo et al., 2006; Cruz de Carvalho, 2008; Farooq et al., 2009a,b; Gill and Tuteja, 2010; Shu et al., 2011). Chinnusamy et al. (2007) reported that chilling stress negatively affects the membrane properties and enzyme activities resulting in tissue necrosis. Under suboptimal growth temperatures, bundle sheath proteins were more sensitive to oxidative damage than those of mesophyll in maize leaves (Kingston-Smith and Foyer, 2000). Overproduction of ROS under chilling stress cause serious cellular damages by rapidly reacting with essential cellular structures (Sattler et al., 2000). Zhang et al. (1995) stated that balance between the formation and detoxification of ROS is crucial to cell survival under chilling stress. Likewise, numerous studies have reported the higher ROS accumulation and oxidative damage under drought stress (Mano, 2002; Blokhina et al., 2003; Cruz de Carvalho, 2008), owing primarily to limited carbon dioxide fixation and higher photorespiration (Cruz de Carvalho, 2008). Robinson and Bunce (2000) documented that considerably higher accumulation of ROS under drought stress resulted from the increased O2 photo reduction rate in chloroplasts.
To cope with oxidative damage the plants have evolved excellent antioxidative defense systems under unfavorable conditions like drought and chilling stresses. Increased activities/levels of several enzymatic (SOD, APX, POD, CAT, GR, GST, GPX etc.), and non-enzymatic (reduced and oxidized glutathione, ascorbic acid, α-tocopherol, and carotenoids) antioxidants have been reported in plants to maintain the cellular homeostasis and to mitigate the oxidative damage (Gill and Tuteja, 2010). The specific role of various enzymatic and non-enzymatic antioxidants in drought and chilling stress tolerance of various transgenic plants is summerized in Table 1. Chilling and drought stress tolerance of many crops is regulated by abscisic acid induced higher antioxidant enzyme activities (Lu et al., 2003; Zhou et al., 2005). In contrast, Thomashow (1999) claimed that chilling stress activated the antioxidant defense response by causing damage to membrane components and ROS-induced protein denaturation. Previously, it has been observed that greater activities/levels of antioxidants were well concominant with chilling tolerance in rice (Guo et al., 2006; Hussain et al., 2016), maize (Zhang et al., 1995; Prasad, 1997; Taka, 2004; Sharma et al., 2012), cucumber (Kang and Saltveit, 2002), and tabbaco (Deng et al., 2012). Likewise, several authors documented the role of different enzymatic and non-enzymatic antioxidants in drought tolerance of maize (Anjum et al., 2017), rice (Sharma and Dubey, 2005), wheat (Sairam and Saxena, 2000; Keles and Öncel, 2002), coffea (Lima et al., 2002), cotton (Ratnayaka et al., 2003), beans (Turkan et al., 2005), and alfalfa (Rubio et al., 2002). This implies that maintaining higher levels of antioxidants may contribute toward stress tolerance in plants.
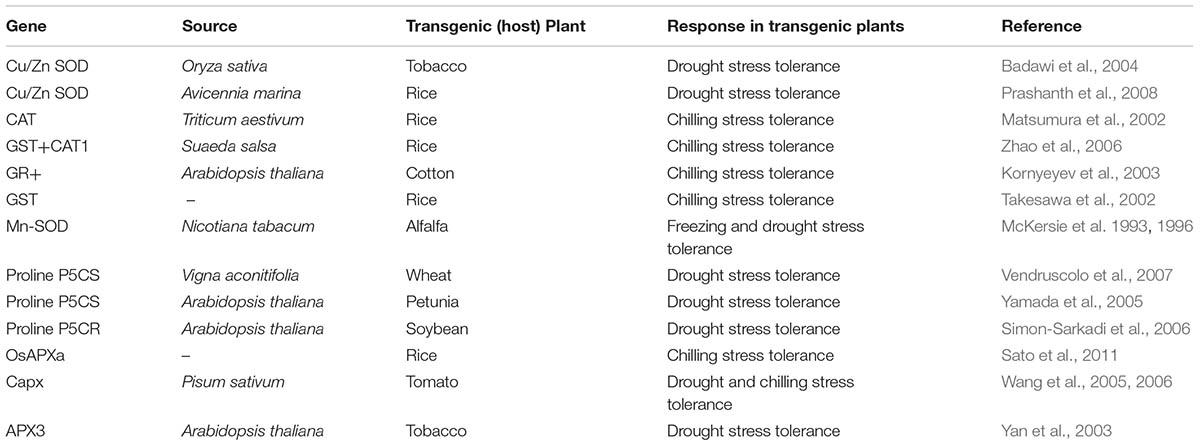
TABLE 1. Role of enzymatic and non-enzymatic antioxidants for inducing drought and chilling tolerance in transgenic plants.
Osmotic Balance
Osmotic adjustment is the decrease of osmotic potential owing to the net accumulation of solutes in response to water deficit conditions (Zhang et al., 1999). Under osmotic stress, plants maintain the water relations by accumulating various osmotically active molecules/ions such as soluble sugars, organic acids, proline, glycinebetaine, potassium, calcium, and chloride ions. Osmoregulation/osmotic adjustment helps plants to sustain growth, photosynthetic rate, and assimilate partitioning under stressful conditions (Subbarao et al., 2000). Farooq et al. (2009a) stated that osmotic adjustment is directly related to the accumulation of osmolytes in different concentrations, which largely depends on stress severity, plant type and the growth stage at which the stress is imposed (Shao et al., 2006). As chilling stress has been reported to disturb plant osmotic balance and cause dehydration stress (Farooq et al., 2009a; Wang et al., 2016), therefore, under chilling and drought stress, osmotic adjustment assists the plants to maintain higher turgor potential (Zhang et al., 1999; Chaves et al., 2009). Morgan (1990) reported that osmotic adjustment is crucial in postponing the dehydration stress under water limited conditions. Greater accumulation of osmolytes is generally linked with drought tolerance (Blum, 2005) because these facilitate in maintenance of leaf turgor to improve conductance of stomata for efficient CO2 intake (Kiani et al., 2007), and promote the water uptake ability of roots (Chimenti et al., 2006). Mercado et al. (1997) reported that protein content in the tissues of chilling-sensitive plants are usually decreased with chilling stress, mainly because of reduced synthesis which, in turn, increases the level of free amino acids particularly proline (Jouve et al., 1993). Endogenous concentrations of proline were linked with chilling tolerance capability in maize (Songstad et al., 1990), and drought tolerance in rice (Hsu et al., 2003), and pea (Alexieva et al., 2001). Proline mainly accumulates in younger leaves and is recognized as the most improtant solute against abiotic stresses (Perez-Perez et al., 2009). Drought stress alters the endogenous levels of glycinebetaine. Cotton cultivars with higher endogenous glycinebetaine concentrations were better adapted to drought conditions than those which accumulaed less glycinebetaine (Naidu et al., 1998). Most of the studies have shown that osmotic potential of plants is more affected by drought as compared to chilling stress (Farooq et al., 2009b). However, further studies are needed to understand the alterations in plant osmotic balance under combined drought and chilling stresses.
Molecular Responses
Crop growth and productivity is negatively affected by molecular alterations under various environmental stresses. Chilling and drought stress induce the expression of number of genes in plants, however, gene expression in response to both stresses are quite different (Liu et al., 1998). In Arabidopsis, expression of DREB1 (also called as CBFs or the c-box binding factors) and DREB2 families was affected in response to chilling and drought stresses, respectively (Stockinger et al., 1997; Liu et al., 1998). Likewise, Yamaguchi-Shinozaki and Shinozaki (1994) identified the dehydration-responsive element with the core sequence A/GCCGAC as a cis-acting promoter element, which were regulated in response to drought, and chilling stresses. Expression of homeodomain leucine zipper (4D-Zip) protein, which interacts with CaCBFIB (Mahajan and Tuteja, 2005) was highly regulated by drought and low temperature stresses (Kim et al., 2004). Chinnusamy et al. (2007) stated that cold temperature affects membrane fluidity, disrupts nucleic acid and protein structures, hinders water and nutrient uptake, and causes significant changes in the plant transcriptome. It also drastically disturbs the cellular metabolism either by directly decreasing the rates of biochemical reactions or indirectly by gene expression reprogramming. Chilling stress rapidly increased the levels of cytoplasmic calcium in Arabidopsis (Polisensky and Braam, 1996) and alfalfa (Monroy and Dhindsa, 1995) due to an influx of calcium from extracellular stores (Mahajan and Tuteja, 2005), suggesting that cell signaling and genes expression are altered under chilling stress. Lee and Lee (2003) observed that expression of majority of pollen-specific genes remained unaffected under chilling stress. However, Jouyban et al. (2013) reported that response of plants to chilling stress was associated with changes in gene transcription of low molecular weight proteins. Differences in genes expression in response to stress also occur among plant parts. Kreps et al. (2002) found that leaves and roots exhibited differential gene expressions during cold acclimation; and 86% of the cold-induced genes were not shared between leaves and roots. Chilling stress regulates the expression of ETHYLENE RESPONSE FACTOR/APETALA2 family transcription factors (e.g., CBFs), which may bind to cis-elements in the promoters of COR (cold-responsive) genes and induce their expression (Chinnusamy et al., 2007). Under cold stress, ICE1 (INDUCER OF CBF EXPRESSION1), was constitutively regulated in Arabidopsis, nevertheless, transgenic overexpression of ZAT12 down-regulated the expression of CBFs (Chinnusamy et al., 2007). Chilling stress also induces the accumulation of proline, which in turn induces the expression of genes having proline-responsive element (PRE and ACTCAT) in their promoters (Satoh et al., 2002; Chinnusamy et al., 2007).
Water deficit conditions also cause the fluctuations in gene expression (up- and down-regulation) in plants (Deng et al., 2009; Jain and Chattopadhyay, 2010). Drought stress altered the expression of dehydrin/late embryogenesis abundant (LEA) genes and molecular chaperones, which protect from protein denaturation in cell (Mahajan and Tuteja, 2005). Drought stress regulates the production of ABA in roots that is transported to the shoots leading to stomatal closure and restricted plant growth (Mittler and Blumwald, 2015). Drought-induced increase in ABA level regulates the expression of dehydrin genes (DHN 1/RAB and DHN 2), glycine rich protein gene, and a conserved element in proximal promoter region of gene RAB28 (Gomez et al., 1988; Pla et al., 1991; Campbell et al., 1998; Rorat, 2006). Under drought stress, miR398 was significantly up-regulated in peanut, while, it decreased the expression levels of isoforms Cu/Zn superoxide dismutase viz., AhCSD1-1/AhCSD1-2.1 and AhCSD2 (Park and Grabau, 2017). Bhargava and Sawant (2013) found that drought stress up-regulated the expression of genes involved in osmolyte synthesis, aquaporins, signaling molecules and transcription factors (TFs), and genes coding for LEA proteins. Moreover, TFs of ERF/AP2 family are known to bind with drought-response element present in promoters of many drought-response genes (Yamaguchi-Shinozaki and Shinozaki, 2005; Maruyama et al., 2009). Yang et al. (2010) observed that gene expression of TFs belonging to AP2/ERF, bZIP, HD-ZIP, bHLH, MYB, NF-Y, EAR, NAC, and ZPT2 families was regulated under drought stress. Bhargava and Sawant (2013) reported that drought stress induces the expression of a variant of histone H1 (called H1-S), which is involved in regulation of stress-responsive gene and plays a crucial role in stomatal closure (Scippa et al., 2004). Chinnusamy and Zhu (2009) reported that drought regulated the DNA demethylation in coding sequence of a glycerophosphodiesterase-like protein gene in tobacco, whereas, DNA hyper-methylation was regulated in pea under drought stress.
In summary, profound changes in gene expression occur under drought or/and chilling stresses (Maqbool et al., 2017). Many stress-inducible genes are responsive to both drought stress and chilling, while some genes are only regulated specifically by drought or chilling stress (Rabbani et al., 2003). Overall, majority of the transcription factors were up-regulated under these stresses, a few transcription factors having a role in primary growth processes were down-regulated (Bhargava and Sawant, 2013).
Crosstalk Between Chilling and Drought Responses
Different abiotic stresses generally induce modify the gene expression in plants which can be stress-specific. Nevertheless, various points of crosstalk may occur amongst stress responses, and interplay of signaling pathways in plants. Cross talk refers to any instance of two pathways from different stress factors that converge and act in synchronization to alleviate the effects of stress (Knight and Knight, 2001). The plant responses to drought and chilling share various common domains including ROS and antioxidant response, calcium signaling, osmotic stress signal perception, transcription factors, hormones, dehydrins, and mitogen-activated protein kinase (MAPK) cascades, to name a few.
Identification of cross talk between different signaling cascades is important in strengthening our knowledge on plants responses to a specific stress condition. In recent era, researches dealing with the cross talk between different abiotic stress signaling pathways have paid attention on genes/gene products involved in two different stress conditions, and thus are known to be part of a shared response. The transgenic up- or down-regulation of these genes indicate that they play key role in enhancing tolerance to multiple abiotic stresses. Therefore, these genes can be crucial in conferring plant tolerance against combined stress factors often prevailing under field conditions.
Among four transcriptional regulatory systems in plants, two are ABA-dependent while two are ABA-independent (Shinozaki et al., 2003), and the crosstalk between these regulatory systems has been shown by molecular and genetic evidence in various reports. Genetic analyses suggested that there is no clear line of delineation between ABA-dependent and ABA-independent pathways, and the contributing components may often cross talk or even congregate in the signaling pathway (Kreps et al., 2002). Generally, the signaling pathways associated with chilling stress are reported to be rather less dependent on ABA than those involved in drought stress response (Shinozaki and Yamaguchi-Shinozaki, 2000; Shinozaki et al., 2003; Chen and Zhu, 2004; Zhang J.Z. et al., 2004). However, it is evident that some cross-talk, at least, does occur between different abiotic stress signaling pathways as the transcription of members of similar gene families is regulated and identical products are rather accumulated (Shinozaki et al., 2003; Chen and Zhu, 2004; Chinnusamy et al., 2004). For instance, the hydrophilic proteins (COR78 and COR15a) were accumulated in Arabidopsis thaliana, in response to both chilling and drought stress (Rajashekar, 2000).
Genomic analyses of stress-induced gene expressions using microarrays also have recently revealed crosstalk in stress-responsive genes (Kreps et al., 2002; Seki et al., 2002). Many drought-responsive genes were regulated by high-salinity stress or ABA; however, only 10% of drought-responsive genes were indeed cold-inducible as well. In fact, ABA is involved in regulation of osmotic stress-responsive genes but it does not specifically play a key role in low-temperature stress response (Kreps et al., 2002; Seki et al., 2002). Presence of ABRE (cis acting element) is generally important for ABA induced gene expressions (Seki et al., 2002).
As both drought and chilling result in cellular dehydration, it is not uncommon to expect some overlap in various signaling pathways focused on delivering protection against cellular dehydration. This commonality presumably occurs due to the involvement of the CBF regulon in the abiotic stress response (Fowler et al., 2005). Haarke et al. (2002) reported that CBF4/DREB1D expression was induced by the occurrence of osmotic-stress. The CBF/DREB1 family genes are mainly regulated by chilling stress, while CBF4 has been reported to be activated by drought stress which provides crosstalk between CBF/DREB1 and DREB2 regulatory systems. The drought-induced expression of CBF4 is controlled by ABA-dependent pathways, indicating that CBF4 may function in drought stress response that relies on ABA accumulation.
Calcium (a secondary messenger) and ROS network (which balances scavenging with production) are the ubiquitous components of both chilling and drought stress signaling pathways. Genes involved in Ca and ROS signaling constitute a crucial part of common molecular response of concurrent stresses in plants (Rabbani et al., 2003; Torres and Dangl, 2005). Generally, rapid increase in Ca levels in plant cells has been reported with ABA, drought, and chilling (Rabbani et al., 2003). Plants are also evolved in high degree of control over ROS toxicity, to extent level that ROS are exploited as signaling molecules (Timperio et al., 2008). The plant cell senses ROS through redox-sensitive transcription factors (e.g., heat-shock factors), which trigger functional proteins involved in the reinstatement of cellular homeostasis (Mittler et al., 2004).
Tommasini et al. (2008) investigated the drought- and low temperature-responsive genes in barley. About 44% genes specifically responded to drought, while only 3.8% were chilling specific, and 9.5% genes were shared between both stresses. Of 2,622 up-regulated genes, 13.0% were chilling specific, and 32.7% were drought specific suggesting that drought induced nearly three times as many stress specific changes as chilling (Kreps et al., 2002; Seki et al., 2002; Rabbani et al., 2003). Genes involved in osmotic stress signal perception (bZIP, MYB29, and MYB-CC), calcium signaling proteins, signal transduction (e.g., MAPK), protection of membranes and proteins (e.g., LEA proteins), and antioxidative defense mechanisms (e.g., glutathione related genes) were commonly regulated in chilling and drought stresses (Table 2). Chilling stress specifically activated the genes involved in cell cycle and DNA processing, signaling cascades and transcriptional control, protein modification and destination, oxidative stress, cellular transport, and cold shock response (Table 2). However, genes related to transcriptional regulation, protection of membranes and proteins, cell growth and cell cycle, water and ion uptake and transport, and ABA synthesis were specifically activated by drought stress (Table 2). Beck et al. (2007) concluded that accumulation of dehydrins is regulated under chilling, salinity, and ABA exposure along with drought stress. They further reported that extremely hydrophilic proteins as dehydrins not only protect biomembranes in ripening seeds (LEA proteins) but also accumulate in the tissue of drought-tolerant plants during cold adaptation.
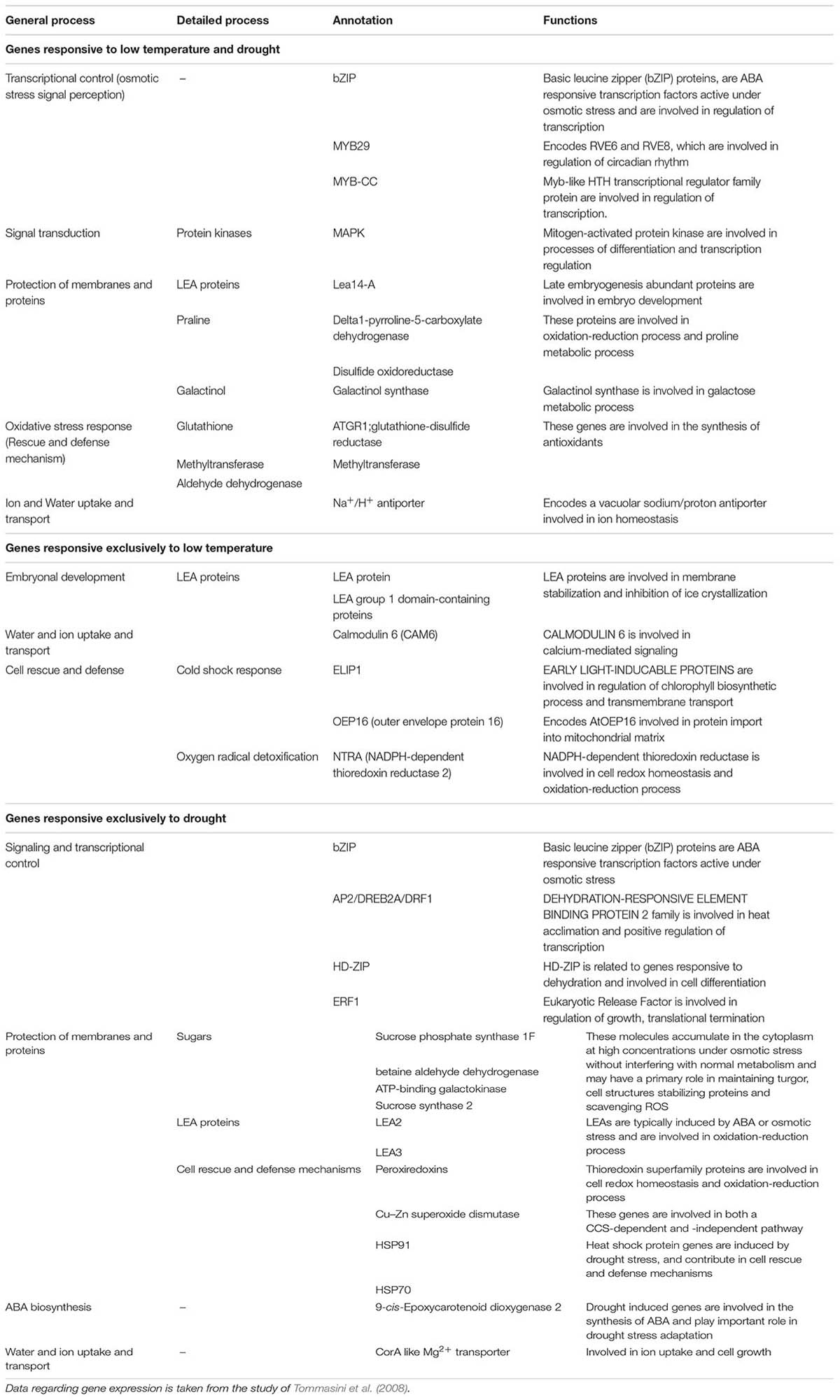
TABLE 2. Shared and unique gene expression patterns in barley induced by low temperature and drought stresses.
Each stress is a multigenic trait and, thus, the manipulation of such genes may lead to alteration of a large number of genes as well as gene products. A deep insight into transcription factors regulating these genes, the products of the major stress-evoked genes, and cross talk between different pathways and responses should remain an area of future research activities. The fact that these cross talk genes regulate both chilling and drought response of plants highlights their importance in enhancing plant tolerance to combined stress factors. However, this should be validated by conducting studies on concurrent stresses wherein expression of cross talk genes needs to be examined.
Management Strategies
Both drought and chilling stresses have adverse effects on the growth and productivity of crop plants (Liu et al., 1998), and plant tolerance to these stresses might have certain protective mechanisms in shared (Stockinger et al., 1997). Plants often develop a cascade of strategies through physiological, biochemical, and molecular modifications at cell level to cope with these stresses. Studies have shown that mechanisms of plant tolerance to chilling and drought stresses can be regulated through developing tolerant plant genotypes, genetic modifications, seed treatments, application of plant growth regulators and compatible solutes and use of plant mineral nutrients.
Selection and Breeding Strategies
Integration of conventional, molecular and omic-based techniques can successfully be employed to develop tolerant plant genotypes (Maqbool et al., 2017). Conventional breeding has been instrumental in developing chilling tolerant cultivars in various crops (Jha et al., 2017). Various rice varieties, e.g., ‘Koshihikari’ in Japan, ‘Silewah’ in Indonesia and ‘Padi Labou Alumbis’ in Malaysia were released for chilling stress tolerance by conventional breeding method (Ahmad P. et al., 2014). Based on both open air and controlled conditions, Rodriguez et al. (2007) reported that ‘EP80 × Puenteareas’ population in maize was an important source of low temperature tolerance. Implementation of the modern omics approaches and identification of QTLs/genes for chilling tolerance can significantly support crop improvement strategies aimed to develop high yielding cultivars under low-temperature condition (Ahmad P. et al., 2014; Jha et al., 2017). In rice, selection using different parameters led to the development of low temperature tolerant genotypes such as ‘HSC55,’ ‘M103,’ and ‘Jyoudeki’ based on low spikelet sterility (Farrell et al., 2006; Ye et al., 2009). Suh et al. (2010) reported that phenotyping selection and SSR makers’ identification methods are helpful in screening of plant genotypes against chilling stress. They found three QTLs responsible for seed setting percentage under chilling stress by using a recombinant inbred line (RIL) population developed by tropical japonica × temperate japonica.
Under drought stress conditions, estimating final grain yield is a simple and easy method for screening tolerant crops. Promising tolerant genotypes can be identified by direct selection of grain yield under water deficit conditions (Verulkar et al., 2010). Hence, conventional breeding approach includes the selection of crop plants for its yield and related attributes under drought conditions (Ahmad P. et al., 2014). In general, breeding strategies have been employed to manipulate the genetic makeup of crops for enhancing their tolerance against drought (Maqbool et al., 2017), however, marker assisted selection (MAS) proved better than classical breeding for screening traits associated with drought tolerance (Ahmad P. et al., 2014) and enhancing drought tolerance in plants (Jongdee et al., 2002). The molecular basis of drought tolerance is generally based on QTL analysis and segregation mapping (Ali et al., 2017). The identification of QTLs associated with drought tolerance is an important tool for MAS of plants with desired characters (Farooq et al., 2009b). Marker assisted recurrent selection involves the accumulation of more superior alleles responsible for drought tolerance in plants (Varshney et al., 2012). A large number of QTLs have already been identified for several drought tolerance traits, nevertheless, epiQTLs and Epistatic QTLs discovered in future can be used for molecular breeding (Gupta et al., 2017). Sequential or simultaneous transfer of QTLs at different developmental stages of rice may lead to cultivars with increased drought tolerance capacities (Lang et al., 2013). Overexpression of an LEA gene has been reported to increase drought tolerance in rice (Xiao et al., 2007). Furthermore, while discussing the constraints in breeding approaches, Ouk et al. (2006) reported that low heritability of grain yield and lack of effective selection criteria are the main hindrances to develop drought tolerant cultivars. Similarly, Kumar et al. (2008) reported that many previous trials on drought stress tolerance failed to impose severe stress, therefore true and precise drought-tolerant lines were not selected. Although, conventional breeding methods for drought tolerance have been used for a long time, nevertheless, advancements in genomics and molecular breeding approaches would have the significant role in the development of drought tolerant cultivars (Kumar et al., 2015). Moreover, selection of crop cultivars for their unique responses to drought and chilling stress might be helpful for breeding programs to develop stress tolerant plant types.
Molecular and Functional Genomics Approaches
Plants respond to drought or chilling stress through a series of mechanistic changes in morpho-physiological features (Shinozaki and Yamaguchi-Shinozaki, 1996). Many genes that respond to drought or low temperature at the transcriptional level have been previously identified (Skriver and Mundy, 1990; Thomashow, 1994; Kasuga et al., 2004; Yadav, 2010; Todaka et al., 2015; Ali et al., 2017; Kaur and Asthir, 2017). However, the products of some specific stress-responsive genes could have significant roles in mitigating stress-induced damage through still elusive mechanisms (Shinozaki et al., 2003). Liu et al. (1998) stated that ABA is generally produced under both drought and chilling stresses and has significant contributions in stress tolerance in plants. They further stated that overexpression of various regulatory elements induces drought and low temperature tolerance in plants (Liu et al., 1998). Kasuga et al. (2004) reported that overexpression of DREB1A enhanced drought and chilling tolerance in tobacco plants. Furthermore, genes encoding transcription factors were recognized better for improving stress tolerance in plants than various other genes responsible for cold tolerance in Arabidopsis and rice (Yadav, 2010). Tuberosa et al. (2008) stated that identification of QTLs involved in chilling stress tolerance may directly relate to acquire valuable genetic diversity in the physiological features in plants. Low-temperature restrictions have been overwhelmed by the support of cold-tolerant genes in genetically modified crops (Sanghera et al., 2011). Chilling stress induces three C-repeat binding factor genes or dehydration-responsive elements such as DREB1B (AtCBF1), DREB1C (AtCBF2), and DREB1A (AtCBF3) in Arabidopsis (Yadav, 2010). Cold tolerance in tobacco can also be enhanced by the transgenic overexpression of the chloroplast omega-3 fatty acid desaturase gene (Kodama et al., 1994). Upchurch (2008) also reported the involvement of omega-3 desaturases to establish chilling tolerance in plants. Liu et al. (2008) described that the overexpression of Lefad7 in transgenic tomato could enhance the chilling stress tolerance. Lukatkin et al. (2012) reported that plant response to low temperature is linked with the extent of change of gene transcription of low molecular weight proteins. Mahajan and Tuteja (2005) described that CBF binds with COR genes carrying CRT/DRE elements, and the over-expression of these COR genes have an imperative role for chilling tolerance and cold acclimation in plants (Thomashow, 1994; Stockinger et al., 1997; Yadav, 2010). These findings signify the roles of cold-inducible genes to protect plant cells against cold stress (Yadav, 2010).
Mechanism of drought tolerance is related to the drought induced regulations of multiple genes expression (Lang and Bui, 2008). Drought tolerant plants developed by recent genomics approaches require clear understandings of the genetic basis of drought tolerance (Xiong et al., 2006), and identification of transcriptions factors associated with drought tolerance is important in this regard. Recently, an active form of DREB2 was found to transactivate target stress-evoked genes that led to better drought stress tolerance in Arabidopsis (Todaka et al., 2015). Ali et al. (2017) also reported the involvement of water stress induced transcription factors (DREB2A and DREB2B) in the expression of various genes responsible for drought stress tolerance in plants. Kaur and Asthir (2017) reported that responses of ABA-responsive elements-binding proteins (AREB) at both transcriptional and post-transcriptional levels of drought also determine the drought tolerance abilities of the plants. Moreover, Afzal et al. (2016) described the importance of aquaporins in regulating the plant-water relations and could be promising targets for developing drought tolerant plant genotypes. Differential responses of two contrastive barley genotypes were found in low-molecular dehydrins under water deficit conditions which were involved in drought stress tolerance (Škodáèek and Prášil, 2011). Bruce et al. (2002) enlighten the necessities to advent the whole genomics to identify the key genes involved in the regulations of drought stress tolerance in plants. Since, drought tolerance is a genetically controlled phenomenon, therefore, identification of QTLs for membrane stability and other functionally related phenomenal genes needs to be explored in future by using bio-informatics whilst many of them has already been characterized (Tripathy et al., 2000; Fu et al., 2007).
Some studies have been shown that some genes expressed by both drought and cold stress concurrently whilst some are only responsive to individual stress (Thomashow, 1994; Liu et al., 1998; Kasuga et al., 2004; Sanghera et al., 2011). There have been several efforts in improving tolerance toward combine stress factors such as concurrent chilling and drought stresses. The improved tolerance to chilling and water deficit stress in Arabidopsis was attained by overexpression of CBF4 (Haake et al., 2002; Sanghera et al., 2011). In addition, the overexpression of DREB1A has been found to have linked with drought and chilling stress in wheat, groundnut and tobacco plants (Kasuga et al., 2004; Pellegrineschi et al., 2004). In order to improve tolerance against chilling and drought stress, applications of various molecular and engineering strategies are needed. It is well evident that the integration of traditional and molecular breeding approaches with genetic engineering and MAS may help the scientists to improve individual and concurrent environmental stresses in crop plants (Chaves and Oliveira, 2004).
Agronomic and Physiological Measures
Plants respond to drought and chilling stress via series of agronomic and physiological processes. Exogenous application of compatible solutes, plant growth hormones, plant mineral nutrient and seed priming can be effective in alleviating the adverse effects of drought and chilling stresses.
Application of Compatible Solutes
Compatible solutes protect the plants from osmotic stress by not posing any detrimental effects on enzymes, membranes, and other macromolecules even at higher concentrations (Cechin et al., 2006; Kiani et al., 2007). Compatible solutes include glycinebetaine, soluble sugars, proline, sugar alcohols, trehalose and some organic acids (Kiani et al., 2007). Many of the osmolytes are organic solutes in nature whilst some of the essential ions such as K+ (Yokoi et al., 2002).
Amongst various osmolytes, proline has been found to have multiple roles in plant stress regulation and tolerance potential (Yamada et al., 2005; Farooq et al., 2009a,b). Proline and polyamines have been reported to impart chilling tolerance in maize (Songstad et al., 1990). Proline retains the potential to relieve low temperature injury in cold-sensitive plants. Although, most of the chilling sensitive plants also accumulate proline but the levels of accumualation are not enough to induce cold tolerance, therefore, exogenous application of proline can be a potential option to enhance its level in plants. The beneficial role of proline in augmenting drought tolerance in rice (Hsu et al., 2003) and pea cultivars (Alexieva et al., 2001) has been well reported.
Soluble sugars and sugar alcohols serve as osomoregulators, signelling molecules, and cryoprotectants in plants (Ruelland et al., 2009) and helps in cleansing stress-generated ROS (Van den Ende and Valluru, 2009). Chilling tolerance has been observed by increase of total soluble sugars in many plant species (Levitt, 1980; Farooq et al., 2009a; Wang et al., 2016). Soluble sugars reduce osmotic potential and enhance water absorption under water limited conditions, and thus improve stress tolerance in plants (Farooq et al., 2009b).
Exogenous application of glycinebetaine play critical roles in abiotic stress tolerance in plants by modifying antioxidant activities, membrance integrity, osmotic adjustment and ROS detoxification (Somersalo et al., 1996; Xing and Rajashekar, 2001; Farooq et al., 2008). Xing and Rajashekar (2001) reported that glycinebetaine accumulation was positively related to the cold stress tolerance in plants. Glycinebetaine improved the growth of Solanum tuberosum lants under low temperature conditions (Somersalo et al., 1996) and freezing tolerance in A. thaliana (Xing and Rajashekar, 2001). Its foliar applicationwas helpful in drought tolerance of several plant species (Xing and Rajashekar, 2001; Farooq et al., 2009b). In short, application of campatible solutes such as glycinebetaine, soluble sugars, proline, and polyamines can be helpful in imparting drought and chilling tolerance in crop plants.
Application of Plant Growth Regulators
Plant growth hormones have a dynamic role for increasing the ability of plants to acclimatize against abiotic stress conditions. Auxins (IAA), gibberellins (GA3), cytokinins (Cks), ethylene, abscisic acid (ABA), salicylic acid (SA), jasmonates (JA), and brassinosteroids (BRs) have been widely studied in plants under different abiotic stresses. These plant hormones are being used efficiently under stress conditions for enhancing crop production. Plants normaly synthesize various types of phytohormones under individual and concurrent abiotic stresses depending upon the strength of their defense mechanism (Nadeem et al., 2016). ABA is synthesized in response to both drought and chilling and contribute in stress tolerance of crop plants (Liu et al., 1998). ABA, JA, and SA often activate phosphoprotein cascade pathways that led to expression of genes associated with cold stress tolerance in plants (Kolaksazov et al., 2013). Greater chilling tolerance has been recorded in ABA-treated maize (Xin and Li, 1992), and levels of ABA are increased in chilling tolerant genotypes (Farooq et al., 2009a). Under drought stress, ABA plays a key role in plant signaling betweeen shoot and root, that results in water-saving antitranspirant response, notably stomatal closure and reduced leaf expansion (Wilkinson et al., 2012). ABA is also involved in robust root growth and other morphological modifications under drought stress (Giuliani et al., 2005). During drought stress, increased accumulation of ABA decreases the Cks contents while triggers the ABA/Cks ratio in plants (Wani et al., 2016). Under water stress conditions, plant growth regulator including BRs and ABA significantly improved chlorophyll content and increased water potential in soybean (Zhang M. et al., 2004). Khan et al. (2015) reported that application of BRs led to considerable improvements in plant tolerance against chilling stress in terms of plant growth, photosynthesis and antioxidant system in tomato plants. Reguera et al. (2013) stated that drought tolerance in rice was concomitant with the accumulation of Cks. Drought tolerance induced by Cks has also been observed in tobacco (Rivero et al., 2007) and wheat (Shang, 2000). Kaya et al. (2006) reported that drought tolerance can also be enhanced by the application of GA3 in maize. Exogenous GA3 application breaks the rosette by rapid enlargement of differentiated tissues under cold stress (Khan et al., 2017). Similarly, JA has been reported to trigger the plant defense responses to abiotic stresses including drought and chilling (Pauwels et al., 2009; Seo et al., 2011). Exogenous application of methyl jasmonate improved chilling tolerance in wheat by modulating antioxidant defense system and production of soluble protein content (Qi et al., 2005). The relationship between enhanced ethylene levels and chilling tolerance in crop plants has also been reported by Chu and Lee (1989) and Zhao et al. (2014).
Salicylic acid is also considered as poterntial chemical compound for drought and chilling tolerance in several plant species (Farooq et al., 2009a,b). SA application was found to be effective in alleviating the chilling (Miura and Tada, 2014) and freezing injury (Tasgin et al., 2003). In maize, the harmful effects of low-temperature stress were decreased by the application of SA (Janda et al., 1999; Wang et al., 2012). Low concentrations of applied SA have also been reported to improve drought tolerance in crop plants (Miura and Tada, 2014). Horváth et al. (2007) reported that significant improvement in performance of winter wheat was related with SA-induced higher activities of CAT under drought stress. Exogenous auxin application improved wheat yield significantly under drought stress conditions (Abdoli et al., 2013), and overepxression of auxin efflux carrier gene (OsPIN3t) in rice was linked with enhanced drought tolerance (Zhang et al., 2012).
Non-hormonal growth regulators, e.g., triazoles, paklobutrazol, chlorocholinchloride, meflidid, and unikonazol (Lurie et al., 1994; Feng et al., 2003) are also used to enhance drought and chilling tolerance in crop plants (Feng et al., 2003; Lukatkin et al., 2012). Still and Pill (2003) observed that foliar applications of paclobutrazol remained effective in improving drought tolerance in tomato seedlings. These evidences suggest the exogeneous application of various plant growth regulators is a potent method to improve the drought and chilling tolerance in plants. However, future research should focus on developing genetically engineered or transgenic plants that have the ability to produce specific hormones by using biotechnological and molecular techniques. These transgenic plants would be capable to grow sucessfully under stressful environments.
Use of Mineral Nutrients
Mineral nutrients play important role in improving plant tolerance against stress conditions (Marschner, 1995; Waraich et al., 2012). To alleviate the adverse effects of abiotic stresses, nitrogen (N) fertilization has been reported to have significant role in stress alleviation (Waraich et al., 2011a). Nitrogen as nitric oxide (NO) is a highly reactive that protects plant against stress conditions by acting as scavenger to ROS (Wendehenne et al., 2001; Waraich et al., 2012). Under drought stress, phosphorus (P) helps plants to maitain leaf water potential which, in turn, enhances stomatal conductivity and photosynthetic rates (Waraich et al., 2011b). Supplementation of K doses and its uptake in plant parts could be beneficial for obtaining reasonable yields under drought conditions (Valadabadi and Farahani, 2010). K-induced increases in proline and free sugar contents in rice were reported by Pandey et al. (2004) under water deficit conditions. Kafkafi (1990) reported that low temperature injuries can be avoided in carnation plants by supplimenting K in irrigation water.
Calcium has been reported enhance the concentrations of amino acids, polyamines (putrescine and spermidine) and chlorophyll content in red spruce under low temperature stress (Schaberg et al., 2011). Waraich et al. (2012) reported that Ca is an essential nutrient for stomatal closure in chilling tolerant genotypes. Application of Ca in Vicia faba enhanced plant biomass, plant water relations, and chlorophyll content while reduced membrane leakage (Abdel-Basset, 1998). Magnesium (Mg) also improve root morphological charcaters which helps to increase uptake of water and nutrients via roots, whlist boron nutrition enhances sugar transport in the plant body and improves seed germination and grain formation process (Waraich et al., 2012). Application of Se was found to improve plant tolerance against chilling (Hawrylak-Nowak et al., 2010) and drought stress (Valadabadi et al., 2010). Silicon application improved the plant water status and biomass accumulation in sorghum (Hattori et al., 2005) and wheat (Gong et al., 2003) under drought stress. These studies suggest that use of plant mineral nutrient is a potiential option to achieve better crop growth and productivity and to alleviate the dterimental effects of drought and/or chilling stresses in a sustainable way.
Seed Priming
Seed priming is considered to improve the abiotic stress tolerance (including chilling and drought) in different plant species (Hasanuzzaman and Fujita, 2011; Hussain et al., 2016; Wang et al., 2016; Samota et al., 2017). Seed priming promotes the germination related metabolic functions without radicle protrusion from seeds (Hussain et al., 2016; Wang et al., 2016; Zheng et al., 2016). It is a useful technique to acclimatize against stress conditions (Samota et al., 2017) by modulating physio-biochemical processes in developing seedlings (Hasanuzzaman and Fujita, 2011). Seed- priming with plant hormones and other chemical compounds can play effective role in alleviating the adverse effects of drought and chilling stresses at earlier growth stages. Seed priming activated antioxidant defense system thus improved seed germination and early growth in tobacco seedlings (Xu et al., 2011). Seed priming with ascorbic acid, SA and H2O2 improved seedling establishment under suboptimal temperature (Ahmad I. et al., 2014). Hussain et al. (2016) found that the seed priming with selenium and SA were effective in improving chilling tolerance in rice cultivars. Likewise, seed priming either with 2.5% K2HPO4 or 2.5% K2HPO4 + KNO3 were observed to induce chilling tolerance at early growth stages than non-primed seeds (Farooq et al., 2009a). Seed priming with SA improved relative water and chlorophyll contents, antioxidant activities, plant biomass and grain yield of wheat (Singh and Usha, 2003), whereas selenium priming regulated activities/levels of enzymatic and non-enzymatic antioxidants in rapeseed under drought stress (Hasanuzzaman and Fujita, 2011). The priming of tomato and bean seeds with 0.1–0.5 mM SA improved plant tolerance to drought and low temperature stresses (Senaratna et al., 2000). Significant improvements in physio-biochemical features and the expression of rice drought-responsive RD1 and RD2 genes of AP2/ERF family in drought tolerant and sensititve rice genotypes were observed when seeds were primed with methyl jasmonate, SA, and paclobutrazol (Samota et al., 2017). Above evidence suggest that seed priming offers a realistic solution to increase the stress tolerance of plants against the drought and suboptimal temperatures.
Conclusions and Future Perspectives
Emergence of intricate stress combinations and their impacts on crop growth and productivity in modern day agriculture are the outcomes of global climate change. Climate change is a multi-facet field that could have long-term impacts in the form of different abiotic stresses. Both drought and chilling stresses are amongst those abiotic stresses that have deleterious effects on crop plants. Plants depict a wide range of responses to these stresses (Figure 1) that could lead to significant reductions in crop yields or some time complete crop failure. Actually co-occurrence of drought and chilling stresses is a major challenge for crop production under field conditions instead of individual stress. Both stresses are also characterized by unique changes in plant growth and development starting from seed germination to yield. The ability of plants to withstand these stresses also differs from species to species. Above evidences affirms that plant responses to combined stresses are unique than those to individual stress factor. Being unique, chilling stress can change the root morphology more adversely than drought stress. In contrast, reduced crop yields as a result of poor dry matter accumulation, reduced flower and pod formation, increased flower and pod abortion, and small seed size are the outcomes of both stresses. Other noticeable effects are diminished nutrient uptake behavior, CO2 diffusion into chloroplast and photosynthetic system in plants. In general, interaction between drought and chilling conditions have found to be additive for almost all plant growth and physiological attributes leading to enhanced damage under combine stresses. In order to overcome the effects of chilling and drought, plants induce biochemical, physiological and molecular modifications that improve tolerance against these stresses. Concurrent occurrence of chilling and drought cause the osmotic as well as oxidative stress on shared basis. Plants counter-balance these adversities by accumulation of compatible solutes and ROS detoxifying proteins, and regulating the activities of antioxidant enzymes. Gene expression is also changed in response to both drought and chilling stresses; some of these genes are regulated only by drought and some genes are chilling-inducible only. To date, various novel approaches have been tested in minimizing the negative effects of combine stresses. Despite of the main improvements there is still a big room for improvement in combination of drought and chilling tolerance. For proper understanding of the plants responses to drought and chilling stress, the experiments should be designed under field conditions. Furthermore, future studies should focus on developing genetically engineered plants by using molecular and biotechnological approaches that have the capability to create a particular response against drought and chilling stress. Transcriptomics and proteomics analysis, genomic sequencing and bioinformatics can help to determine the common and unique genes modulated under stress condition. Thus, these techniques can improve the sophisticated and efficient network in plant response to drought and chilling stresses and subsequently help in the improvement of plant tolerance and productivity.
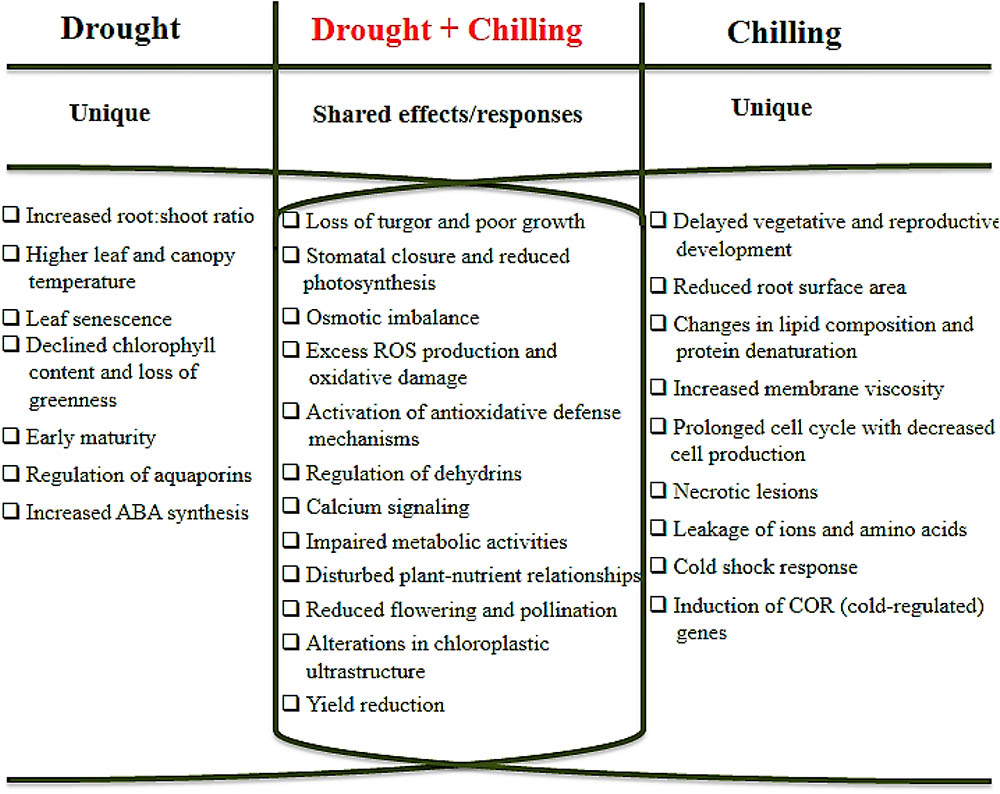
FIGURE 1. A schematic diagram of unique and shared effects of drought and chilling stresses on crop plants.
Author Contributions
HH, SH, and LW conceived the idea of the review and prepared the initial outline. HH, SH, UA, SA, and SM gathered the literature and contributed to writing the different sections. AK and LW provided the technical guidance and editing support.
Funding
This work was financially supported by Special Fund for Agro-Scientific Research in the Public Interest (No. 201503127), the National Science Foundation (No. 31271673), and Chongqing Postgraduate Research and Innovation Project (No. CYS17077).
Conflict of Interest Statement
The authors declare that the research was conducted in the absence of any commercial or financial relationships that could be construed as a potential conflict of interest.
References
Abdel-Basset, R. (1998). Calcium channels and membrane disorders induced by drought stress in vicia faba, plants supplemented with calcium. Acta Physiol. Plant. 20, 149–153. doi: 10.1007/s11738-998-0006-4
Abdoli, M., Saeidi, M., Azhand, M., Jalali-Honarmand, S., Esfandiari, E., and Shekari, F. (2013). The effects of different levels of salinity and indole-3-acetic acid (IAA) on early growth and germination of wheat seedling. J. Stress Physiol. Biochem. 9, 329–338.
Afzal, Z., Howton, T. C., Sun, Y., and Mukhtar, M. S. (2016). The roles of aquaporins in plant stress responses. J. Dev. Biol. 9, 1–22. doi: 10.3390/jdb4010009
Ahmad, I., Basra, S. M. A., and Wahid, A. (2014). Exogenous application of ascorbic acid, salicylic acid and hydrogen peroxide improves the productivity of hybrid maize at low temperature stress. Int. J. Agric. Biol. 3, 14–22.
Ahmad, P., Wani, M. R., Azooz, M. M., and Tran, L. S. P. (2014). Improvement of Crops in the Era of Climatic Changes. New York, NY: Springer. doi: 10.1007/978-1-4614-8830-9
Alexieva, V., Sergiev, I., Mapelli, S., and Karanov, E. (2001). The effect of drought and ultraviolet radiation on growth and stress markers in pea and wheat. Plant Cell Environ. 24, 1337–1344. doi: 10.1046/j.1365-3040.2001.00778.x
Ali, F., Bano, A., and Fazal, A. (2017). Recent methods of drought stress tolerance in plants. Plant Growth Regul. 82, 363–375. doi: 10.1007/s10725-017-0267-2
Andaya, V. C., and Mackill, D. J. (2003). Mapping of QTLs associated with cold tolerance during the vegetative stage in rice. J. Exp. Bot. 54, 2579–2585. doi: 10.1093/jxb/erg243
Anjum, F., Yaseen, M., Rasool, E., Wahid, A., and Anjum, S. (2003). Water stress in barley (Hordeum vulgare L.). i. effect on morphological characters. Pak. J. Agric. Sci. 40, 45–49.
Anjum, S. A., Ashraf, U., Tanveer, M., Khan, I., Hussain, S., Shahzad, B., et al. (2017). Drought induced changes in growth, osmolyte accumulation and antioxidant metabolism of three maize hybrids. Front. Plant Sci. 8:69. doi: 10.3389/fpls.2017.0006
Anjum, S. A., Xie, Y., Wang, L. C., Saleem, M. F., Man, C., and Lei, W. (2011). Morphological, physiological and biochemical responses of plants to drought stress. Afr. J. Agri. Res. 6, 2026–2032.
Apel, K., and Hirt, H. (2004). Reactive oxygen species: metabolism, oxidative stress, and signal transduction. Annu. Rev. Plant Biol. 55, 373–399. doi: 10.1146/annurev.arplant.55.031903.141701
Aroca, R., Vernieri, P., Irigoyen, J. J., Sancher-Diaz, M., Tognoni, F., and Pardossi, A. (2003). Involvement of abscisic acid in leaf and root of maize (Zea mays L.) in avoiding chilling-induced water stress. Plant Sci. 165, 671–679. doi: 10.1016/S0168-9452(03)00257-7
Ashraf, M., and Iram, A. (2005). Drought stress induced changes in some organic substances in nodules and other plant parts of two potential legumes differing in salt tolerance. Flora 200, 535–546. doi: 10.1016/j.flora.2005.06.005
Asrar, A. W. A., and Elhindi, K. M. (2011). Alleviation of drought stress of marigold (Tagetes erecta) plants by using arbuscular mycorrhizal fungi. S. J. Biol. Sci. 18, 93–98. doi: 10.1016/j.sjbs.2010.06.007
Atkinson, N. J., Lilley, C. J., and Urwin, P. E. (2013). Identification of genes involved in the response to simultaneous biotic and abiotic stress. Plant Physiol. 162, 2028–2041. doi: 10.1104/pp.113.222372
Babu, R. C., Shashidhar, H. E., Lilley, J. M., Thanh, N. D., Ray, J. D., Sadasivam, S., et al. (2001). Variation in root penetration ability, osmotic adjustment and dehydration tolerance among accessions of rice adapted to rainfed lowland and upland ecosystems. Plant Breed. 120, 233–238. doi: 10.1046/j.1439-0523.2001.00578.x
Badawi, G. H., Yamauchi, Y., Shimada, E., Sasaki, R., Kawano, N., Tanaka, K., et al. (2004). Enhanced tolerance to salt stress and water deficit by overexpressing superoxide dismutase in tobacco (Nicotiana tabacum) chloroplasts. Plant Sci. 166, 919–928. doi: 10.1016/j.plantsci.2003.12.007
Baier, M., Kandlbinder, A., Golldack, D., and Dietz, K. (2005). Oxidative stress and ozone: perception, signalling and response. Plant Cell Environ. 28, 1012–1020. doi: 10.1111/pce.12288
Barnabás, B., Jäger, K., and Fehér, A. (2008). The effect of drought and heat stress on reproductive processes in cereals. Plant Cell Environ. 31, 11–38.
Basu, S., Ramegowda, V., Kumar, A., and Pereira, A. (2016). Plant adaptation to drought stress. F1000 Res. 5:1554. doi: 10.12688/f1000research.7678.1
Beck, E. H., Fettig, S., Knake, C., Hartig, K., and Bhattarai, T. (2007). Specific and unspecific responses of plants to cold and drought stress. J. Biosci. 32, 501–510. doi: 10.1007/s12038-007-0049-5
Ben-Haj-Salah, H., and Tardieu, F. (1995). Temperature affects expansion rate of maize leaves without change in spatial distribution of cell length (analysis of the coordination between cell division and cell expansion). Plant Physiol. 109, 861–870. doi: 10.1104/pp.109.3.861
Bhargava, S., and Sawant, K. (2013). Drought stress adaptation: metabolic adjustment and regulation of gene expression. Plant Breed. 132, 21–32. doi: 10.1111/pbr.12004
Blokhina, O., Virolainen, E., and Fagerstedt, K. V. (2003). Antioxidants, oxidative damage and oxygen deprivation stress: a review. Ann. Bot. 91, 179–194. doi: 10.1093/aob/mcf118
Blum, A. (2005). Drought resistance, water-use efficiency, and yield potential—are they compatible, dissonant, or mutually exclusive? Aust. J. Agric. Res. 56, 1159–1168. doi: 10.1071/AR05069
Bota, J., Flexas, J., and Medrano, H. (2004). Is photosynthesis limited by decreased Rubisco activity and RuBP content under progressive water stress? New Phytol. 162, 671–681. doi: 10.1093/aob/mcn244
Bruce, W. B., Edmeades, G. O., and Barker, T. C. (2002). Molecular and physiological approaches to maize improvement for drought tolerance. J. Exp. Bot. 53, 13–25. doi: 10.1093/jexbot/53.366.13
Campbell, S. A., Crone, D. E., Ceccardi, T. L., and Close, T. J. (1998). Aca. 40 kDa maize (Zea mays L.) embryo dehydrin is encoded by the dhn2 locus on chromosome 9. Plant Mol. Biol. 38, 417–423. doi: 10.1023/A:1006037308167
Campos, M. K. F. D., Carvalho, K. D., Souza, F. S. D., Marur, C. J., Pereira, L. F. P., Filho, J. C. B., et al. (2011). Drought tolerance and antioxidant enzymatic activity in transgenic ‘swingle’ citrumelo plants over-accumulating proline. Environ. Exp. Bot. 72, 242–250. doi: 10.1016/j.envexpbot.2011.03.009
Cechin, I., Rossi, S. C., Oliveira, V. C., and Fumis, T. F. (2006). Photosynthetic responses and proline content of mature and young leaves of sunflower plants under water deficit. Photosynthetica 44, 143–146. doi: 10.1007/s11099-005-0171-2
Chaves, M. M., Flexas, J., and Pinheiro, C. (2009). Photosynthesis under drought and salt stress: regulation mechanisms from whole plant to cell. Ann. Bot. 103, 551–560. doi: 10.1093/aob/mcn125
Chaves, M. M., and Oliveira, M. M. (2004). Mechanisms underlying plant resilience to water deficits: prospects for water-saving agriculture. J. Exp. Bot. 55, 2365–2384. doi: 10.1093/jxb/erh269
Chen, W., Guo, C., Hussain, S., Zhu, B., Deng, F., Xue, Y., et al. (2016). Role of xylo-oligosaccharides in protection against salinity-induced adversities in Chinese cabbage. Environ. Sci. Pollut. Res. 23, 1254–1264. doi: 10.1007/s11356-015-5361-2
Chen, W. J., and Zhu, T. (2004). Networks of transcription factors with roles in environmental stress response. Trend. Plant Sci. 9, 591–596. doi: 10.1016/j.tplants.2004.10.007
Chimenti, C. A., Marcantonio, M., and Hall, A. J. (2006). Divergent selection for osmotic adjustment results in improved drought tolerance in maize (Zea mays L.) in both early growth and flowering phases. Field Crops Res. 95, 305–315. doi: 10.1016/j.fcr.2005.04.003
Chinnusamy, V., Schumaker, K., and Zhu, J. K. (2004). Molecular genetic perspectives on cross-talk and specificity in abiotic stress signaling in plants. J. Exp. Bot. 55, 225–236. doi: 10.1093/jxb/erh005
Chinnusamy, V., Zhu, J., and Zhu, J. K. (2007). Cold stress regulation of gene expression in plants. Trend. Plant Sci. 12, 444–451. doi: 10.1016/j.tplants.2007.07.002
Chinnusamy, V., and Zhu, J. K. (2009). Epigenetic regulation of stress responses in plants. Curr. Opin. Plant Biol. 12, 1–7. doi: 10.1016/j.pbi.2008.12.006
Chu, C., and Lee, T. M. (1989). The relationship between ethylene biosynthesis and chilling tolerance in seedlings of rice (Oryza sativa L.). Bot. Bull. Acad. Sin. 30, 263–273.
Clarke, H. J., and Siddique, K. H. M. (2004). Response of chickpea genotypes to low temperature stress during reproductive development. Field Crops Res. 90, 323–334. doi: 10.1016/j.fcr.2004.04.001
Cruz, R., and Milach, S. (2004). Cold tolerance at the germination stage of rice: methods of evaluation and characterization of genotypes. Sci. Agric. 61, 1–8. doi: 10.1590/S0103-90162004000100001
Cruz de Carvalho, M. H. (2008). Drought stress and reactive oxygen species: production, scavenging and signaling. Plant Signal. Behav. 3, 156–165. doi: 10.4161/psb.3.3.5536
Cutforth, H. W., Shaykewich, C. F., and Cho, C. M. (1986). Effect of soil water and temperature on corn (Zea mays L.) root growth during emergence. Can. J. Soil. Sci. 66, 51–58. doi: 10.4141/cjss86-006
Davies, S. L., Turner, N. C., Siddique, K. H. M., Plummer, J. A., and Leport, L. (1999). Seed growth of desi and kabuli chickpea (Cicer arietinum L.) in a short season Mediterranean-type environment. Aust. J. Exp. Agric. 39, 181–188. doi: 10.1071/EA98134
Deng, B., Du, W., Liu, C., Sun, W., Tian, S., and Dong, H. (2012). Antioxidant response to drought, cold and nutrient stress in two ploidy levels of tobacco plants: low resource requirement confers polytolerance in polyploids?. Plant Growth Regul. 66, 37–47. doi: 10.1007/s10725-011-9626-6
Deng, X. F., Fu, F. L., Na, N. I., and Li, W. C. (2009). Differential gene expression in response to drought stress in maize seedling. Agric. Sci. China 8, 767–776. doi: 10.1016/S1671-2927(08)60277-1
Domisch, T., Finér, L., and Lehto, T. (2002). Growth, carbohydrate and nutrient allocation of scots pine seedlings after exposure to simulated low soil temperature in spring. Plant Soil 246, 75–86. doi: 10.1023/A:1021527716616
Fang, X., Turner, N. C., Yan, G., Li, F., and Siddique, K. H. M. (2010). Flower numbers, pod production, pollen viability, and pistil function are reduced and flower and pod abortion increased in chickpea (Cicer arietinum L.) under terminal drought. J. Exp. Bot. 61, 335–345. doi: 10.1093/jxb/erp307
Farooq, M., Basra, S. M. A., Wahid, A., Cheema, Z. A., Cheema, M. A., and Khaliq, A. (2008). Physiological role of exogenously applied glycine betaine in improving drought tolerance of fine grain aromatic rice (Oryza sativa L.). J. Agron.Crop Sci. 194, 325–333. doi: 10.1111/j.1439-037X.2008.00323.x
Farooq, M., Aziz, T., Wahid, A., Lee, D. J., and Siddique, K. H. M. (2009a). Chilling tolerance in maize: agronomic and physiological approaches. Crop Pasture Sci. 60, 501–516. doi: 10.1071/CP08427
Farooq, M., Wahid, A., Kobayashi, N., Fujita, D., and Basra, S. M. A. (2009b). Plant drought stress: effects, mechanisms and management. Agron. Sustain. Dev. 29, 185–212. doi: 10.1051/agro:2008021
Farrell, T. C., Fox, K. M., William, R. L., Fukai, S., and Lewin, L. G. (2006). Minimizing cold damage during reproductive development among temperate rice genotypes. II. Genotypic variation and flowering traits related to cold tolerance screening. Aust. J. Agric. Res. 57, 89–100. doi: 10.1071/AR05186
Feng, Z., Guo, A., and Feng, Z. (2003). Amelioration of chilling stress by triadimefon in cucumber seedlings. Plant Growth Regul. 39, 277–283. doi: 10.1023/A:1022881628305
Fowler, S. G., Cook, D., and Thomashow, M. F. (2005). Low temperature induction of Arabidopsis CBF1, 2, and 3 is gated by the circadian clock. Plant Physiol. 137, 961–968. doi: 10.1104/pp.104.058354
Foyer, C. H., and Noctor, G. (2000). Oxygen processing in photosynthesis: regulation and signaling. New Phytol. 146, 359–388. doi: 10.1046/j.1469-8137.2000.00667.x
Frederick, J. R., Camp, C. R., and Bauer, P. J. (2001). Drought-stress effects on branch and main stem seed yield and yield components of determinate soybean. Crop Sci. 41, 759–763. doi: 10.2135/cropsci2001.413759x
Fu, B. Y., Xiong, J. H., Zhu, L. H., Zhao, X. Q., Xu, H. X., Gao, Y. M., et al. (2007). Identification of functional candidate genes for drought tolerance in rice. Mol. Genet. Genomics 278, 599–609. doi: 10.1007/s00438-007-0276-3
Fu, J., and Huang, B. (2001). Involvement of antioxidants and lipid peroxidation in the adaptation of two cool-season grasses to localized drought stress. Environ. Exp. Bot. 45, 105–114. doi: 10.1016/S0098-8472(00)00084-8
Furlan, A., Llanes, A., Luna, V., and Castro, S. (2012). Physiological and biochemical responses to drought stress and subsequent rehydration in the symbiotic association peanut Bradyrhizobium sp. ISRN Agron. 2012, 1–8. doi: 10.5402/2012/318083
Gill, S. S., and Tuteja, N. (2010). Reactive oxygen species and antioxidant machinery in abiotic stress tolerance in crop plants. Plant Physiol. Biochem. 48, 909–930. doi: 10.1016/j.plaphy.2010.08.016
Giuliani, S., Sanguineti, M. C., Tuberosa, R., Bellotti, M., Salvi, S., and Landi, P. (2005). Root-ABA1, a major constitutive QTL, affects maize root architecture and leaf ABA concentration at different water regimes. J. Exp. Bot. 56, 3061–3070. doi: 10.1093/jxb/eri303
Gomez, J., Sanchez-Martinez, D., Stiefel, V., Rigau, J., Puigdomènech, P., and Pages, M. (1988). A gene induced by the plant hormone abscisic acid in response to water stress encodes a glycine-rich protein. Nature 334, 262–264. doi: 10.1038/334262a0
Gong, H., Chen, K., Chen, G., Wang, S., and Zhang, C. (2003). Effects of silicon on growth of wheat under drought. J. Plant Nutr. 26, 1055–1063. doi: 10.1007/s13205-017-0904-5
Guo, Z. F., Ou, W. Z., Lu, S. Y., and Zhong, Q. (2006). Differential responses of antioxidative system to chilling and drought in four rice cultivars differing in sensitivity. Plant Physiol. Biochem. 44, 828–836. doi: 10.1016/j.plaphy.2006.10.024
Gupta, P. K., Balyan, H. S., and Gahlaut, V. (2017). QTL Analysis for drought tolerance in wheat: present status and future possibilities. Agronomy 7, 1–21. doi: 10.3390/agronomy7010005
Haake, V., Cook, D., Riechmann, J. L., Pineda, O., Thomashow, M. F., and Zhang, J. Z. (2002). Transcription factor CBF4 is a regulator of drought adaptation in Arabidopsis. Plant Physiol. 130, 639–648. doi: 10.1104/pp.006478
Haarke, V., Cook, D., Riechmann, J. L., Omaira, P., Thomashow, M. F., and Zang, J. Z. (2002). Transcription factor CBF4 is a regulator of drought adaptation in Arabidopsis. Plant Physiol. 130, 639–648. doi: 10.1104/pp.006478
Haldimann, P. (1998). Low growth temperature-induced changes to pigment composition and photosynthesis in Zea mays, genotypes differing in chilling sensitivity. Plant Cell Environ. 21, 200–208. doi: 10.1046/j.1365-3040.1998.00260.x
Hasanuzzaman, M., and Fujita, M. (2011). Selenium pre-treatment up-regulates the antioxidant defense and methylglyoxal detoxification system and confers enhanced tolerance to drought stress in rapeseed seedlings. Biol. Trace Elem. Res. 143, 1758–1776. doi: 10.1007/s12011-011-8998-9
Hattori, T., Inanaga, S., Hideki, A., Ping, A., Shigenori, M., Miroslava, L., et al. (2005). Application of silicon enhanced drought tolerance in Sorghum bicolor. Physiol. Plant. 123, 459–466. doi: 10.1111/j.1399-3054.2005.00481.x
Havlin, J. L., Beaton, J. D., Tisdale, S. L., and Nelson, W. L. (1999). Soil Fertility and Fertilizers: An Introduction to Nutrient Management, 6th Edn. London: Macmillan Inc., 409.
Hawrylak-Nowak, B., Matraszek, R., and Szymańska, M. (2010). Selenium modifies the effect of short-term chilling stress on cucumber plants. Biol. Trace Elem. Res. 138, 307–315. doi: 10.1007/s12011-010-8613-5
Horváth, E., Pál, M., Szalai, G., Páldi, E., and Janda, T. (2007). Exogenous 4-hydroxybenzoic acid and salicylic acid modulate the effect of short term drought and freezing stress on wheat plants. Biol. Plant. 51, 480–487. doi: 10.1007/s10535-007-0101-1
Hsiao, T. C., and Xu, L. K. (2000). Sensitivity of growth of root versus leaves to water stress; biophysical analysis and relation to water transport. J. Exp. Bot. 51, 1595–1616. doi: 10.1093/jexbot/51.350.1595
Hsu, S. Y., Hsu, Y. T., and Kao, C. H. (2003). The effect of polyethylene glycol on proline accumulation in rice leaves. Biol. Plant. 46, 73–78. doi: 10.1023/A:1022362117395
Hu, Y., Burucs, Z., Tucher, S. V., and Schmidhalter, U. (2007). Short-term effects of drought and salinity on mineral nutrient distribution along growing leaves of maize seedlings. Environ. Exp. Bot. 60, 268–275. doi: 10.1016/j.envexpbot.2006.11.003
Hu, Y., and Schmidhalter, U. (2005). Drought and salinity: a comparison of their effects on mineral nutrition of plants. J. Plant Nutr. Soil Sci. 168, 541–549. doi: 10.1002/jpln.200420516
Hund, A., Richner, W., Soldati, A., Fracheboud, Y., and Stamp, P. (2007). Root morphology and photosynthetic performance of maize inbred lines at low temperature. Eur. J. Agron. 27, 52–61. doi: 10.1016/j.eja.2007.01.003
Hussain, S., Khan, F., Cao, W., Wu, L., and Geng, M. (2016). Seed priming alters the production and detoxification of reactive oxygen intermediates in rice seedlings grown under sub-optimal temperature and nutrient supply. Front. Plant Sci. 7:439. doi: 10.3389/fpls.2016.00439
Jain, D., and Chattopadhyay, D. (2010). Analysis of gene expression in response to water deficit of chickpea (Cicer arietinum L.) varieties differing in drought tolerance. BMC Plant Biol. 10:24. doi: 10.1186/1471-2229-10-24
Jaleel, C. A., Gopi, R., Sankar, B., Gomathinayagam, M., and Panneerselvam, R. (2008). Differential responses in water use efficiency in two varieties of Catharanthus roseus under drought stress. Comp. Rend. Biol. 331, 42–47. doi: 10.1016/j.crvi.2007.11.003
Janda, T., Szalai, G., Tari, I., and Paldi, E. (1999). Hydroponic treatment with salicylic acid decreases the effects of chilling injury in maize (Zea mays L.) plants. Planta 208, 175–180. doi: 10.1007/s004250050547
Janowiak, F., and Markowski, A. (1994). Changes in leaf water relations and injuries in maize seedlings induced by different chilling conditions. J. Agron. Crop Sci. 172, 19–28. doi: 10.1111/j.1439-037X.1994.tb00155.x
Janska, A., Mars, P., Zelenkova, S., and Ovesna, J. (2009). Cold stress and acclimation–what is important for metabolic adjustment? Plant Biol. 12, 395–405. doi: 10.1111/j.1438-8677.2009.00299.x
Javier, P. D. J., José, I. J., and Manuel, S. D. (1997). Chilling of drought-hardened and non-hardened plants of different chilling-sensitive maize lines changes in water relations and aba contents. Plant Sci. 122, 71–79. doi: 10.1016/S0168-9452(96)04540-2
Jha, U. C., Bohra, A., and Jha, R. (2017). Breeding approaches and genomics technologies to increase crop yield under low-temperature stress. Plant Cell Rep. 36, 1–35. doi: 10.1007/s00299-016-2073-0
Jongdee, B., Fukai, S., and Cooper, M. (2002). Leaf water potential and osmotic adjustment as physiological traits to improve drought tolerance in rice. Field Crop Res. 76, 153–163. doi: 10.1016/S0378-4290(02)00036-9
Jouve, L., Engelmann, F., Noirot, M., and Charrier, A. (1993). Evaluation of biochemical markers (sugar, proline, malonedialdehyde and ethylene) for cold sensitivity in microcuttings of two coffee species. Plant Sci. 91, 109–116. doi: 10.1016/0168-9452(93)90194-5
Jouyban, Z., Hasanzade, R., and Sharafi, S. (2013). Chilling stress in plants. Int. J. Agric. Crop Sci. 5:2961.
Jung, S., and Steffen, K. L. (1997). Influence of photosynthetic photon flux densities before and during long-term chilling on xanthophyll cycle and chlorophyll fluorescence quenching in leaves of tomato (Lycopersicon hirsutum). Physiol. Plant. 100, 958–966. doi: 10.1111/j.1399-3054.1997.tb00023.x
Kafkafi, U. (1990). “The functions of plant K in overcoming environmental stress situations,” in Proceedings of the 22nd Colloquium, International Potash Institute, Bern, 81–93.
Kamara, A. Y., Menkir, A., Badu–Apraku, B., and Ibikunle, O. (2003). The influence of drought stress on growth, yield and yield components of selected maize genotypes. J. Agric. Sci. 141, 43–50. doi: 10.1017/S0021859603003423
Kang, H. M., and Saltveit, M. E. (2002). Chilling tolerance of maize cucumber and rice seedling leaves and roots are differentially affected by salicylic acid. Physiol. Plant. 115, 571–576. doi: 10.1034/j.1399-3054.2002.1150411.x
Kashiwagi, J., Krishnamurthy, L., Purushothaman, R., Upadhyaya, H. D., Gaur, P. M., Gowda, C. L. L., et al. (2015). Scope for improvement of yield under drought through the root traits in chickpea (Cicer arietinum L.). Field Crop Res. 170, 47–54. doi: 10.1016/j.fcr.2014.10.003
Kasuga, M., Miura, S., Shinozaki, K., and Yamaguchi-Shinozaki, K. (2004). A combination of the Arabidopsis DREB1A gene and stress-inducible rd29A promoter improved drought-and low-temperature stress tolerance in tobacco by gene transfer. Plant Cell Physiol. 45, 346–350. doi: 10.1093/pcp/pch037
Kaur, G., and Asthir, B. (2017). Molecular responses to drought stress in plants. Biol. Plant. 61, 201–209. doi: 10.1007/s10535-016-0700-9
Kaya, M. D., Okçub, G., Ataka, M., Çıkılıc, Y., and Kolsarıcıa, Ö. (2006). Seed treatments to overcome salt and drought stress during germination in sunflower (Helianthus annuus L.). Eur. J. Agron. 24, 291–295. doi: 10.1016/j.eja.2005.08.001
Keles, Y., and Öncel, I. (2002). Response of antioxidative defence system to temperature and water stress combinations in wheat seedlings. Plant Sci. 163, 783–790. doi: 10.1016/S0168-9452(02)00213-3
Khan, T. A., Fariduddin, Q., and Yusuf, M. (2015). Lycopersicon esculentum under low temperature stress: an approach toward enhanced antioxidants and yield. Environ. Sci. Pollut. Res. 22, 14178–14188. doi: 10.1007/s11356-015-4658-5
Khan, T. A., Fariduddin, Q., and Yusuf, M. (2017). Low-temperature stress: is phytohormones application a remedy? Environ. Sci. Pollut. Res. 24, 21574–21590. doi: 10.1007/s11356-017-9948-7
Kiani, S. P., Talia, P., Maury, P., Grieu, P., Heinz, R., Perrault, A., et al. (2007). Genetic analysis of plant water status and osmotic adjustment in recombinant inbred lines of sunflower under two water treatments. Plant Sci. 172, 773–787. doi: 10.1016/j.plantsci.2006.12.007
Kim, S., An, C. S., Hong, Y. N., and Lee, K. W. (2004). Cold-inducible transcription factor, CaCBF, is associated with a homeodomain leucine zipper protein in hot pepper (Capsicum annuum L.). Mol. Cell 18, 300–308.
Kingston-Smith, A. H., and Foyer, C. H. (2000). Bundle sheath proteins are more sensitive to oxidative damage than those of the mesophyll in maize leaves exposed to parquet or low temperatures. J. Exp. Bot. 51, 123–130. doi: 10.1093/jexbot/51.342.123
Knight, H., and Knight, M. R. (2001). Abiotic stress signaling pathways: specificity and cross-talk. Trend. Plant Sci. 6, 262–267. doi: 10.1016/S1360-1385(01)01946-X
Kodama, H., Hamada, T., Horiguchi, G., Nishimura, M., and Iba, K. (1994). Genetic enhancement of cold tolerance by expression of a gene for chloroplast [omega]-3 fatty acid desaturase in transgenic tobacco. Plant Physiol. 105, 601–605. doi: 10.1104/pp.105.2.601
Kolaksazov, M., Laporte, F., Ananieva, K., Dobrev, P., Herzog, M., and Ananiev, E. D. (2013). Effect of chilling and freezing stresses on jasmonate content in Arabis alpina. Bulg. J. Agric. Sci. 19, 15–17.
Korkmaz, A., and Dufault, R. J. (2001). Developmental consequences of cold temperature stress at transplanting on seedling and field growth and yield. I. Watermelon. J. Am. Soc. Hort. Sci. 126, 404–409.
Kornyeyev, D., Logan, B. A., Payton, P. R., Allen, R. D., and Holaday, A. S. (2003). Elevated chloroplastic glutathione reductase activities decrease chilling-induced photoinhibition by increasing rates of photochemistry, but not thermal energy dissipation, in transgenic cotton. Funct. Plant Biol. 30, 101–110. doi: 10.1071/FP02144
Kreps, J. A., Wu, Y., Chang, H. S., Zhu, T., Wang, X., and Harper, J. (2002). Transcriptome changes for Arabidopsis in response to salt, osmotic, and cold stress. Plant Physiol. 130, 2129–2141. doi: 10.1104/pp.008532
Kuchenbuch, R., Claassen, N., and Jungk, A. (1986). Potassium availability in relation to soil-moisture. Effect of soil-moisture on potassium diffusion, root-growth and potassium uptake of onion plants. Plant Soil 95, 221–231. doi: 10.1007/BF02375074
Kumar, A., Bernier, J., Verulkar, S., Lafitte, H. R., and Atlin, G. N. (2008). Breeding for drought tolerance: direct selection for yield, response to selection and use of drought-tolerant donors in upland and lowland-adapted populations. Field Crops Res. 107, 221–231. doi: 10.1016/j.fcr.2008.02.007
Kumar, M., Uniyal, M., Kumar, N., Kumar, S., and Gangwar, R. (2015). Conventional and molecular breeding for development of drought tolerant maize cultivars. J. Crop Sci. Tech. 4, 1–3.
Lang, N. T., and Bui, B. C. (2008). Fine mapping for drought tolerance in rice (Oryza sativa L.). Omonrice 16, 9–15.
Lang, N. T., Nha, C. T., Ha, P. T. T., and Buu, C. B. (2013). Quantitative trait loci (QTLs) associated with drought tolerance in rice (Oryza sativa L.). Sabrao J. Breed. Genet. 45, 409–421.
Lee, J. Y., and Lee, D. H. (2003). Use of serial analysis of gene expression technology to reveal changes in gene expression in Arabidopsis pollen undergoing cold stress. Plant Physiol. 132, 517–529. doi: 10.1104/pp.103.020511
Lee, T. M., Lur, H. S., and Chu, C. (1993). Role of abscisic acid in chilling tolerance of rice (Oryza sativa L.) seedlings. Endogenous abscisic acid levels. Plant Cell Environ. 16, 481–490. doi: 10.1111/j.1365-3040.1993.tb00895.x
Levitt, J. (1980). Responses of Plants to Environmental Stress: Chilling, Freezing, and High Temperature Stresses, Vol. 1 Cambridge, MA: Academic Press.
Li, C., Jiang, D., Wollenweber, B., Li, Y., Dai, T., and Cao, W. (2011). Waterlogging pretreatment during vegetative growth improves tolerance to waterlogging after anthesis in wheat. Plant Sci. 180, 672–678. doi: 10.1016/j.plantsci.2011.01.009
Li, X. N., Pu, H. C., Liu, F. L., Zhou, Q., Cai, J., Dai, T. B., et al. (2015). Winter wheat photosynthesis and grain yield responses to spring freeze. Agron. J. 107, 1002–1010. doi: 10.2134/agronj14.0460
Lima, A. L. S., da Matta, F. M., Pinheiro, H. A., Totola, M. R., and Loureiro, M. E. (2002). Photochemical responses and oxidative stress in two clones of Coffea canephora under water deficit conditions. Environ. Exp. Bot. 47, 239–247. doi: 10.1016/S0098-8472(01)00130-7
Liu, F., Jensen, C. R., and Andersen, M. N. (2004). Drought stress effect on carbohydrate concentration in soybean leaves and pods during early reproductive development: its implication in altering pod set. Field Crops Res. 86 1–13. doi: 10.1016/S0378-4290(03)00165-5
Liu, Q., Kasuga, M., Sakuma, Y., Abe, H., Miura, S., Yamaguchi-Shinozaki, Y., et al. (1998). Two transcription factors, DREB1 and DREB2, with an EREBP /AP2 DNA binding domain separate two cellular signal transduction pathways in drought- and low-temperature-responsive gene expression, respectively, in Arabidopsis. Plant Cell 10, 1391–1406. doi: 10.1105/tpc.10.8.1391
Liu, X. Y., Li, B., Yang, J. H., Sui, N., Yang, X. M., and Meng, Q. W. (2008). Overexpression of tomato chloroplast omega-3 fatty acid desaturase gene alleviates the photo inhibition of photosystems 2 and 1 under chilling stress. Photosynthetica 46, 185–192. doi: 10.1007/s11099-008-0030-z
Lu, S., Guo, Z., and Peng, X. (2003). Effects of ABA and S-3307 on drought resistance and antioxidative enzyme activity of turf-grass. J. Hortic. Sci. Biotech. 78, 663–666. doi: 10.1080/14620316.2003.11511681
Lukatkin, A. S., Brazaityte, A., Bobinas, C., and Duchovskis, P. (2012). Chilling injury in chilling-sensitive plants: a review. Agriculture 99, 111–124.
Lurie, S., Ronen, R., Lipsker, Z., and Aloni, B. (1994). Effects of paclobutrazol and chilling temperatures on lipids, antioxidants and ATPase activity of plasma membrane isolated from green bell pepper fruits. Physiol. Plant. 91, 593–598. doi: 10.1111/j.1399-3054.1994.tb02993.x
Mahajan, S., and Tuteja, N. (2005). Cold, salinity and drought stresses: an overview. Arch. Biochem. Biophys. 444, 139–158. doi: 10.1016/j.abb.2005.10.018
Manivannan, P., Jaleel, C. A., Sankar, B., Kishorekumar, A., Somasundaram, R., Alagu Lakshmanan, G. M., et al. (2007). Growth, biochemical modifications and proline metabolism in Helianthus annuus L. as induced by drought stress. Colloids Surf. B Biointerfaces 59, 141–149. doi: 10.1016/j.colsurfb.2007.05.002
Mano, J. (2002). “Early events in environmental stresses in plants induction mechanisms of oxidative stress,” in Oxidative Stress in Plants, eds D. Inze and M. V. Montganu (Abingdon: Taylor & Francis), 217–246.
Maqbool, M. A., Aslam, M., and Ali, H. (2017). Breeding for improved drought tolerance in Chickpea (Cicer arietinum L.). Plant Breed. 136, 300–318. doi: 10.1111/pbr.12477
Maruyama, K., Takeda, M., Kidokoro, S., Yamada, K., Sakuma, Y., Urano, K., et al. (2009). Metabolic pathways involved in cold acclimation identified by integrated analysis of metabolites and transcripts regulated by DREB1A and DREB2A. Plant Physiol. 150, 1972–1980. doi: 10.1104/pp.109.135327
Matsumura, T., Tabayashi, N., Kamagata, Y., Souma, C., and Saruyama, H. (2002). Wheat catalase expressed in transgenic rice can improve tolerance against low temperature stress. Physiol. Plant. 116, 317–327. doi: 10.1034/j.1399-3054.2002.1160306.x
Mckersie, B. D., Bowley, S. R., Harjanto, E., and Leprince, O. (1996). Water-deficit tolerance and field performance of transgenic alfalfa overexpressing superoxide dismutase. Plant Physiol. 111, 1177–1181. doi: 10.1104/pp.111.4.1177
McKersie, B. D., Chen, Y. R., Debeus, M., Bowley, S. R., Bowler, C., Inze, D., et al. (1993). Superoxide dismutase enhances tolerance of freezing stress in transgenic alfalfa (Medicago sativa L.). Plant Physiol. 103, 1155–1163. doi: 10.1104/pp.103.4.1155
McWilliam, J. R., Kramer, P. J., and Musser, R. L. (1982). Temperature-induced water stress in chilling-sensitive plants. Aust. J. Plant Physiol. 9, 343–352. doi: 10.1071/PP9820343
Mercado, J. A., Reid, M. S., Valpuesta, V., and Quesada, M. A. (1997). Metabolic changes and susceptibility to chilling stress in Capsicum annuum plants grown at suboptimal temperature. Aust. J. Plant Physiol. 24, 759-767. doi: 10.1071/PP97066
Mittler, R. (2002). Oxidative stress, antioxidants and stress tolerance. Trends Plant Sci. 7, 405-410. doi: 10.1016/S1360-1385(02)02312-9
Mittler, R. (2006). Abiotic stress, the field environment and stress combination. Trends Plant Sci. 11, 15–19. doi: 10.1016/j.tplants.2005.11.002
Mittler, R., and Blumwald, E. (2015). The roles of ROS and ABA in systemic acquired acclimation. Plant Cell 27, 64–70. doi: 10.1105/tpc.114.133090
Mittler, R., Vanderauwera, S., Gollery, M., and Van Breusegem F. (2004). Reactive oxygen gene network of plants. Trend. Plant Sci. 9, 490–498. doi: 10.1016/j.tplants.2004.08.009
Miura, K., and Tada, Y. (2014). Regulation of water, salinity, and cold stress responses by salicylic acid. Fron. Plant Sci. 5:4. doi: 10.3389/fpls.2014.00004
Monroy, A. F., and Dhindsa, R. S. (1995). Low-temperature signal transduction: induction of cold acclimation-specific genes of alfalfa by calcium at 25 degrees C. Plant Cell 7, 321–331. doi: 10.2307/3869854
Morgan, P. W. (1990). “Effects of abiotic stresses on plant hormone systems,” in Stress Responses in Plants: Adaptation and Acclimation Mechanisms, eds R. G. Alscher and J. R. Cumming (New York, NY: Wiley-Liss, Inc.), 113–146.
Nadeem, S. M., Ahmad, M., Zahir, Z. A., and Kharal, M. A. (2016). “Role of phytohormones in stress tolerance of plants,” in Plant, Soil and Microbes eds K. R. Hakeem and M. S. Akhtar (Cham: Springer International Publishing), 385–421. doi: 10.1007/978-3-319-29573-2_17
Naidu, B. P., Cameron, D. F., and Konduri, S. V. (1998). “Improving drought tolerance of cotton by glycine betaine application and selection,” in Proceedings of the 9th Australian Agronomy Conference, Wagga Wagga, NSW.
Nayyar, H., Bains, T., and Kumar, S. (2005). Low temperature induced floral abortion in chickpea: relationship to abscisic acid and cryoprotectants in reproductive organs. Environ. Exp. Bot. 53, 39–48. doi: 10.1016/j.envexpbot.2004.02.011
Nayyar, H., and Gupta, D. (2006). Differential sensitivity of C3 and C4 plants to water deficit stress: association with oxidative stress and antioxidants. Environ. Exp. Bot. 58, 106–113. doi: 10.1016/j.envexpbot.2005.06.021
Nejad, T. S., Bakhshande, A., Nasab, S. B., and Payande, K. (2010). Effect of drought stress on corn root growth. Rep. Opin. 2, 47–52.
Nie, G. Y., Hendrix, D. L., Weber, A. N., Kimball, B. A., and Long, S. P. (1995). Increased accumulation of carbohydrates and decreased photosynthetic gene transcript levels in wheat grown at an elevated CO2 concentration in the field. Plant Physiol. 108, 975–983. doi: 10.1104/pp.108.3.975
Nonami, H. (1998). Plant water relations and control of cell elongation at low water potentials, J. Plant Res. 111, 373–382. doi: 10.1007/BF02507801
Oliver, S. N., Dennis, E. S., and Dolferus, R. (2007). ABA regulates apoplastic sugar transport and is a potential signal for cold-induced pollen sterility in rice. Plant Cell Physiol. 48, 1319–1330. doi: 10.1093/pcp/pcm100
Ouk, M., Basnayake, J., Tsubo, M., Fukai, S., Fischer, K. S., Cooper, M., et al. (2006). Use of drought response index for identification of drought tolerant genotypes in rainfed lowland rice. Field Crops Res. 99, 48–58. doi: 10.1016/j.fcr.2006.03.003
Pan, W. L., Jackson, W. A., and Moll, R. H. (1985). Nitrate uptake and partitioning by corn (Zea mays L.) root systems and associated morphological differences among genotypes and stages of root development. J. Exp. Bot. 36, 1341–1351. doi: 10.1093/jxb/36.9.1341
Pandey, P., Ramegowda, V., and Senthil-Kumar, M. (2015). Shared and unique responses of plants to multiple individual stresses and stress combinations: physiological and molecular mechanisms. Front. Plant Sci. 6:723. doi: 10.3389/fpls.2015.00723
Pandey, R., Agarwal, R. M., Jeevaratnam, K., and Sharma, G. L. (2004). Osmotic stress induced alterations in rice (Oryza sativa L.) and recovery. Plant Growth Regul. 42, 79–87. doi: 10.1023/B:GROW.0000014893.45112.55
Park, S. Y., and Grabau, E. (2017). Bypassing miRNA-mediated gene regulation under drought stress: alternative splicing affects CSD1 gene expression. Plant Mol. Biol. 95, 243–252. doi: 10.1007/s11103-017-0642-4
Pauwels, L., Inzé, D., and Goossens, A. (2009). Jasmonate-inducible gene: what does it mean?. Trend. Plant Sci. 14, 87–91. doi: 10.1016/j.tplants.2008.11.005
Pellegrineschi, A., Reynolds, M., Pacheco, M., Brito, R. M., Almeraya, R., Yamaguch-Shinozaki, K., et al. (2004). Stress induced expression in wheat of the Arabidopsis thaliana DREB1A gene delays water stress symptoms under greenhouse conditions. Genome 47, 493–500. doi: 10.1139/g03-140
Perez-Perez, J. G., Robles, J. M., Tovar, J. C., and Botia, P. (2009). Response to drought and salt stress of lemon ‘Fino 49’ under field conditions: water relations, osmotic adjustment and gas exchange. Sci Hortic. 122, 83–90. doi: 10.1016/j.scienta.2009.04.009
Pettigrew, W. T. (2004). Physiological consequences of moisture deficit stress in cotton. Crop Sci. 44, 1265–1272. doi: 10.2135/cropsci2004.1265
Pla, M., Gómez, J., Goday, A., and Pagès, M. (1991). Regulation of the abscisic acid-responsive gene rab28, in maize viviparous, mutants. Mol. Gen. Genet. 230, 394–400. doi: 10.1007/BF00280296
Polisensky, D. H., and Braam, J. (1996). Cold-shock regulation of the Arabidopsis TCH genes and the effects of modulating intracellular calcium levels. Plant Physiol. 111, 1271–1279. doi: 10.1104/pp.111.4.1271
Prasad, T. K. (1997). Role of catalase in inducing chilling tolerance in pre emergence maize seedlings. Plant Physiol. 114, 1369–1376. doi: 10.1104/pp.114.4.1369
Prashanth, S. R., Sadhasivam, V., and Parida, A. (2008). Over expression of cytosolic copper/zinc superoxide dismutase from a mangrove plant Avicennia marina in indica rice var Pusa Basmati-1 confers abiotic stress tolerance. Transgen. Res. 17, 281–291. doi: 10.1007/s11248-007-9099-6
Priestley, D. A. (1986). Seed Ageing: Implications for Seed Storage and Persistence in the Soil. Ithaca, NY: Comstock Associates, 304.
Qi, F., Li, J., Duan, L., and Li, Z. (2005). Inductions of coronatine and MeJA to low-temperature resistance of wheat seedlings. Acta Bot. Boreal Occident. Sin. 26, 1776–1780.
Rabbani, M. A., Maruyama, K., Abe, H., Khadri, M. A., Katsura, K., Ito, Y., et al. (2003). Monitoring expression profiles of rice genes under cold, drought, and high-salinity stresses and abscisic acid application using cDNA microarray and RNA gel-blot analyses. Plant Physiol. 133, 1755–1767. doi: 10.1104/pp.103.025742
Rajashekar, C. B. (2000). “Cold response and freezing tolerance in plants,” in Plant–Environment Interactions, 2nd Edn, ed. R. E. Wilkinson (New York, NY: Marcel Dekker, Inc), 321–341.
Ratnayaka, H. H., Molin, W. T., and Sterling, T. M. (2003). Physiological and antioxidant responses of cotton and spurred anoda under interference and mild drought. J. Exp. Bot. 54, 2293–2305. doi: 10.1093/jxb/erg251
Reddy, A. R., Chaitanya K. V., and Vivekanandan, M. (2004). Drought induced responses of photosynthesis and antioxidant metabolism in higher plants. J. Plant Physiol. 161, 1189–1202. doi: 10.1016/j.jplph.2004.01.013
Reguera, M., Peleg, Z., Abdel-Tawab, Y. M., Tumimbang, E. B., Delatorre, C. A., and Blumwald, E. (2013). Stress induced cytokinin synthesis increases drought tolerance through the coordinated regulation of carbon and nitrogen assimilation in rice. Plant Physiol. 163, 1609–1622. doi: 10.1104/pp.113.227702
Richner, W., Soldati, A., and Stamp, P. (1996). Shoot-to-root relations in field grown maize seedlings. Agron. J. 88, 56–61. doi: 10.2134/agronj1996.00021962008800010012x
Rivero, R. M., Kojima, M., Gepstein, A., Sakakibara, H., Mittler, R., Gepstein, S., et al. (2007). Delayed leaf senescence induces extreme drought tolerance in a flowering plant. Proc. Natl. Acad. Sci. U.S.A. 104, 19631–19636. doi: 10.1073/pnas.0709453104
Rizza, F., Crosatti, C., Stanca, A. M., and Cattivelli, L. (1994). Studies for assessing the influence of hardening on cold tolerance of barley genotypes. Euphytica 75, 131–138. doi: 10.1007/BF00024540
Robinson, M., and Bunce, J. A. (2000). Influence of drought-induced water stress on soybean and spinach leaf ascorbate dehydroascorbate level and redox status. Int. J. Plant Sci. 161, 271–279. doi: 10.1086/314257
Rodriguez, V. M., Malvar, R. A., Butron, A., Ordas, A., and Revilla, P. (2007). Maize populations as sources of favorable alleles to improve cold-tolerant hybrids. Crop Sci. 47, 1779–1786. doi: 10.2135/cropsci2007.01.0008
Rorat, T. (2006). Plant dehydrins-tissue location, structure and function. Cell. Mol. Biol. Lett. 11, 536–556. doi: 10.2478/s11658-006-0044-0
Rubio, M. C., Gonzalez, E. M., Minchin, F. R., Webb, K. J., Arrese-Igor, C., Ramos, J., et al. (2002). Effects of water stress on antioxidant enzymes of leaves and nodules of transgenic alfalfa overexpressing superoxide dismutases. Physiol. Plant. 115, 531–540. doi: 10.1034/j.1399-3054.2002.1150407.x
Ruelland, E., Vaultier, M. N., Zachowski, A., and Hurry, V. (2009). Cold signalling and cold acclimation in plants. Adv. Bot. Res. 49, 35–150. doi: 10.1016/S0065-2296(08)00602-2
Rymen, B., Fiorani, F., Kartal, F., Vandepoele, K., Inzé, D., and Beemster, G. T. S. (2007). Cold nights impair leaf growth and cell cycle progression in maize through transcriptional changes of cell cycle genes. Plant Physiol. 143, 1429–1438. doi: 10.1104/pp.106.093948
Sacks, M. M., Silk, W. K., and Burman, P. (1997). Effect of water stress on cortical cell division rates within the apical meristem of primary roots of maize. Plant Physiol. 114, 519–527. doi: 10.1104/pp.114.2.519
Sairam, R. K., and Saxena, D. C. (2000). Oxidative stress and antioxidants in wheat genotypes: possible mechanism of water stress tolerance. J. Agron. Crop Sci. 184, 55–61. doi: 10.1046/j.1439-037x.2000.00358.x
Sales, C. R. G., Ribeiro, R. V., Silveira, J. A. G., Machado, E. C., Martins, M. O., and Lagôa, A. M. M. A. (2013). Superoxide dismutase and ascorbate peroxidase improve the recovery of photosynthesis in sugarcane plants subjected to water deficit and low substrate temperature. Plant Physiol. Biochem.73, 326–336. doi: 10.1016/j.plaphy.2013.10.012
Samota, M. K., Sasi, M., Awana, M., Yadav, O. P., Amitha, M. S. V., Tyagi, A., et al. (2017). Elicitor-induced biochemical and molecular manifestations to improve drought tolerance in rice (Oryza sativa L.) through seed-priming. Front. Plant Sci. 8:934. doi: 10.3389/fpls.2017.0093
Sanghera, G. S., Wani, S. H., Hussain, W., and Singh, N. B. (2011). Engineering cold stress tolerance in crop plants. Curr. Genom. 12, 30–43. doi: 10.2174/138920211794520178
Sato, Y., Masuta, Y., Saito, K., Murayama, S., and Ozawa, K. (2011). Enhanced chilling tolerance at the booting stage in rice by transgenic overexpression of the ascorbate peroxidase gene, OsAPXa. Plant Cell Rep. 30, 399–406. doi: 10.1007/s00299-010-0985-7
Satoh, R., Nakashima, K., Seki, M., Shinozaki, K., and Shinozaki, K. Y. (2002). ACTCAT, a novel cis-acting element for proline- and hypoosmolarity-responsive expression of the ProDH gene encoding proline dehydrogenase in Arabidopsis. Plant Physiol. 130, 709–719. doi: 10.1104/pp.009993
Sattler, U., Calsou, P., Boiteux, S., and Salles, B. (2000). Detection of oxidative base dna damage by a new biochemical assay. Arch. Biochem. Biophys. 376, 26–33. doi: 10.1006/abbi.2000.1701
Schaberg, P. G., Minocha, R., Long, S., Halman, J. M., Hawley, G. J., and Eagar, C. (2011). Calcium addition at the hubbard brook experimental forest increases the capacity for stress tolerance and carbon capture in red spruce (Picea rubens) trees during the cold season. Trees 25, 1053–1061. doi: 10.1007/s00468-011-0580-8
Schuppler, U., He, P., John, P. C. L., and Munns, R. (1998). Effect of water stress on cell division and cell-division-cycle 2-like cell-cycle kinase activity in wheat leaves. Plant Physiol. 117, 667–678. doi: 10.1104/pp.117.2.667
Scippa, G. S., Di Michele, M., Onelli, E., Patrignani, G., Chiatante, D., and Bray, E. A. (2004). The histone-like protein H1-S and the response of tomato leaves to water deficit. J. Exp. Bot. 55, 99–109. doi: 10.1093/jxb/erh022
Seki, M., Narusaka, M., Ishida, J., Nanjo, T., Fujita, M., Oono, Y., et al. (2002). Monitoring the expression profiles of ca. 7000 Arabidopsis genes under drought, cold, and high-salinity stresses using a full-length cDNA microarray. Plant. J. 31, 279–292. doi: 10.1046/j.1365-313X.2002.01359.x
Senaratna, T., Touchell, D., Bunn, E., and Dixon, K. (2000). Acetyl salicylic acid (aspirin) and salicylic acid induce multiple stress tolerance in bean and tomato plants. Plant Growth Regul. 30, 157–161. doi: 10.1023/A:1006386800974
Seo, J. S., Joo, J., Kim, M. J., Kim, Y. K., Nahm, B. H., Song, S. I., et al. (2011). OsbHLH148, a basic helix-loop-helix protein, interacts with OsJAZ proteins in a jasmonate signaling pathway leading to drought tolerance in rice. Plant J. 65, 907–921. doi: 10.1111/j.1365-313X.2010.04477.x
Sewelam, N., Oshima, Y., Mitsuda, N., and Ohme-Takagi, M. (2014). A step towards understanding plant responses to multiple environmental stresses: a genome-wide study. Plant Cell Environ. 37, 2024–2035. doi: 10.1111/pce.12274
Shang, Z. (2000). Effect of 6-BA and KT on photophosphorylation activity in wheat flag leaves under water stress. Acta Agric. Boreal Sin. 15, 34–38.
Shao, H. B., Chen, X. Y., Chu, L. Y., Zhao, X. N., Wu, G. Y., and Yong, B. (2006). Investigation on the relationship of proline with wheat anti drought under soil water deficits. Colloids Surf. B. Bio. Interfaces 53, 113–119. doi: 10.1016/j.colsurfb.2006.08.008
Sharma, P., and Dubey, R. S. (2005). Drought induces oxidative stress and enhances the activities of antioxidant enzymes in growing rice seedlings. Plant Growth Regul. 46, 209–221. doi: 10.1007/s10725-005-0002-2
Sharma, P., Jha, A. B., Dubey, R. S., and Pessarakli, M. (2012). Reactive oxygen species, oxidative damage, and antioxidative defense mechanism in plants under stressful conditions. J. Bot. 2012:217037. doi: 10.1016/j.plaphy.2016.05.038
Shinozaki, K., and Yamaguchi-Shinozaki, K. (1996). Molecular responses to drought and cold stress. Curr. Opin. Biotechnol. 7, 161–167. doi: 10.1016/S0958-1669(96)80007-3
Shinozaki, K., and Yamaguchi-Shinozaki, K. (2000). Molecular responses to dehydration and low temperature: differences and cross-talk between two stress signaling pathways. Curr. Opin. Plant Biol. 3, 217–223. doi: 10.1016/S1369-5266(00)00067-4
Shinozaki, K., Yamaguchi-Shinozaki, K., and Seki, M. (2003). Regulatory network of gene expression in the drought and cold stress responses. Curr. Opin. Plant Biol. 6, 410–417. doi: 10.1016/S1369-5266(03)00092-X
Shu, D. F., Wang, L. Y., Duan, M., Deng, Y. S., and Meng, Q. W. (2011). Antisense-mediated depletion of tomato chloroplast glutathione reductase enhances susceptibility to chilling stress. Plant Physiol. Biochem. 49, 1228–1237. doi: 10.1016/j.plaphy.2011.04.005
Siddique, M., Hamid, A., and Islam, M. (2001). Drought stress effects on water relations of wheat. Bot. Bull. Acad. Sin. 41, 35–39.
Simon-Sarkadi, L., Kocsy, G., Várhegyi,Á., Galiba, G., and De Ronde, J. A. (2006). Stress-induced changes in the free amino acid composition in transgenic soybean plants having increased proline content. Biol. Plant. 50, 793–796. doi: 10.1007/s10535-006-0134-x
Singh, B., and Usha, K. (2003). Salicylic acid induced physiological and biochemical changes in wheat seedlings under water stress. Plant Growth Regul. 39, 137–141. doi: 10.1023/A:1022556103536
Škodáèek, Z., and Prášil, I. T. (2011). New possibilities for research of barley (Hordeum vulgare L.) drought resistance. Úroda 8, 24–29.
Skriver, K., and Mundy, J. (1990). Gene expression in response to abscisic acid and osmotic stress. Plant Cell 2, 503–512. doi: 10.1105/tpc.2.6.503
Somersalo, S., Kyei-Boahen, S., and Pehu, E. (1996). Exogenous glycine betaine application as a possibility to increase low temperature tolerance of crop plants. Nordisk Jordbruksforsk. 78:10.
Songstad, D. D., Duncan, D. R., and Widholm, J. M. (1990). Proline and polyamine involvement in chilling tolerance of maize suspension cultures. J. Exp. Bot. 41, 289–294. doi: 10.1093/jxb/41.3.289
Sowiński, P., Rudzińska-Langwald, A., Adamczyk, J., Kubica, I., and Fronk, J. (2005). Recovery of maize seedling growth, development and photosynthetic efficiency after initial growth at low temperature. J. Plant Physiol. 162, 67–80. doi: 10.1016/j.jplph.2004.03.006
Srivalli, B., Sharma, G., and Khana-Chopra, R. (2003). Antioxidative defense system in an upland rice cultivar subjected to increasing intensity of water stress followed by recovery. Physiol. Plant. 119, 503–512. doi: 10.1046/j.1399-3054.2003.00125.x
Starck, Z., Niemyska, B., Bogdan, J., and Tawalbeh, R. N. A. (2000). Response of tomato plants to chilling stress in association with nutrient or phosphorus starvation. Plant Soil 226, 99–106. doi: 10.1023/A:1026497104077
Steffens, D. (1986). “Root system and potassium exploitation,” in Proceedings of the IPI-Congress: Nutrient Balances and the Need for Potassium, Berne, 107–188.
Stewart, C. R., Martin, B. A., Reding, L., and Cerwick, S. (1990). Seedling growth, mitochondrial characteristics, and alternative respiratory capacity of corn genotypes differing in cold tolerance. Plant Physiol. 92, 761–766. doi: 10.1104/pp.92.3.761
Still, J. R., and Pill, W. G. (2003). Germination, emergence and seedlings growth of tomato and impatiens in response to seed treatment with paclobutrazol. Hortic. Sci. 38, 1201–1204.
Stockinger, E. J., Gilmour, S. J., and Thomashow, M. F. (1997). Arabidopsis thaliana CBF1 encodes an AP2 domain-containing transcriptional activator that binds to the C-repeat /DRE, a cis-acting DNA regulatory element that stimulates transcription in response to low temperature and water deficit. Proc. Natl. Acad. Sci. U.S.A. 94, 1035–1040. doi: 10.1073/pnas.94.3.1035
Subbarao, G. V., Nam, N. H., Chauhan, Y. S., and Johansen, C. (2000). Osmotic adjustment, water relations and carbohydrate remobilization in pigeonpea under water deficits. J. Plant Physiol. 157, 651–659. doi: 10.1016/S0176-1617(00)80008-5
Subramanian, K. S., Santhanakrishnan, P., and Balasubramanian, P. (2006). Responses of field grown tomato plants to arbuscular mycorrhizal fungal colonization under varying intensities of drought stress. Sci. Hortic. 107, 245–253. doi: 10.1016/j.scienta.2005.07.006
Suh, J. P., Jeung, J. U., Lee, J. I., Choi, Y. H., Yea, J. D., Virk, P. S., et al. (2010). Identification and analysis of QTLs controlling cold tolerance at the reproductive stage and validation of effective QTLs in cold-tolerant genotypes of rice (Oryza sativa L.). Theor. Appl. Genet. 120, 985–995. doi: 10.1007/s00122-009-1226-8
Suriyagoda, L., De Costa, W. A. J. M., and Lambers, H. (2014). Growth and phosphorus nutrition of rice when inorganic fertiliser application is partly replaced by straw under varying moisture availability in sandy and clay soils. Plant Soil 384, 53–68. doi: 10.1007/s11104-014-2049-1
Svensson, J., Ismail, A., Palva, E. T., and Close, T. J. (2002). “Dehydrins,” in Cell and Molecular Responses to Stress, eds K. B. Storey J. B. Storey (Amsterdam: Elsevier), 155–171. doi: 10.1016/S1568-1254(02)80013-4
Tahir, M. H. N., Imran, M., and Hussain, M. K. (2002). Evaluation of sunflower (Helianthus annuus L.) inbred lines for drought tolerance. Int. J. Agric. Biol. 3, 398–400.
Taka, T. (2004). The relationship of antioxidant enzymes and some physiological parameters in maize during chilling. Plant Soil Environ. 50, 27–32. doi: 10.17221/3638-PSE
Takesawa, T., Ito, M., Kanzaki, H., Kameya, N., and Nakamura, I. (2002). Over-expression of ζ glutathione S-transferase in transgenic rice enhances germination and growth at low temperature. Mol. Breed. 9, 93–101. doi: 10.1023/A:1026718308155
Tarchoune, I., Sgherric, C., Izzo, R., Lachaal, M., Ouerghi, Z., and Navari-Izzo, F. (2010). Antioxidative response of Ocimum basilicum to sodium chloride and sodium sulphate salinization. Plant Physiol. Biochem. 48, 772–777. doi: 10.1016/j.plaphy.2010.05.006
Tasgin, E., Atici, O., and Nalbantoglu, B. (2003). Effects of salicylic acid and cold on freezing tolerance in winter wheat leaves. Plant Growth Regul. 41, 231–236. doi: 10.1023/B:GROW.0000007504.41476.c2
Tezara, W., Mitchell, V. J., Driscoll, S. D., and Lawlor, D. W. (2002). Effects of water deficit and its interaction with CO2 supply on the biochemistry and physiology of photosynthesis in sunflower. J. Exp. Bot. 53, 1781–1791. doi: 10.1093/jxb/erf021
Thakur, P., Kumar, S., Malik, J. A., Berger, J. D., and Nayyar, H. (2010). An overview: cold stress effects on reproductive development in grain crops. Environ. Exp. Bot. 67, 429–443. doi: 10.1016/j.envexpbot.2009.09.004
Thomashow, M. F. (1994). “Arabidopsis thaliana as a model for studying mechanisms of plant cold tolerance,” in Arabidopsis, eds E. M. Meyerowitz and C. R. Somerville (Cold Spring Harbor, NY: Cold Spring Harbor Laboratory Press), 807–834.
Thomashow, M. F. (1999). Plant cold acclimation: freezing tolerance genes and regulatory mechanisms. Annu. Rev. Plant Biol. 50, 571–599. doi: 10.1146/annurev.arplant.50.1.571
Timperio, A. M., Egidi, M. G., and Zolla, L. (2008). Proteomics applied on plant abiotic stresses: role of heat shock proteins (HSP). J Proteom. 71, 391–411. doi: 10.1016/j.jprot.2008.07.005
Todaka, D., Shinozaki, K., and Yamaguchi-Shinozaki, K. (2015). Recent advances in the dissection of drought-stress regulatory networks and strategies for development of drought-tolerant transgenic rice plants. Front. Plant Sci. 6:84. doi: 10.3389/fpls.2015.00084
Tommasini, L., Svensson, J. T., Rodriguez, E. M., Wahid, A., Malatrasi, M., Kato, K., et al. (2008). Dehydrin gene expression provides an indicator of low temperature and drought stress: transcriptome-based analysis of Barley (Hordeum vulgare L.). Funct. Integr. Genomics. 8, 387–405. doi: 10.1007/s10142-008-0081-z
Torres, M. A., and Dangl, J. L. (2005). Functions of the respiratory burst oxidase in biotic interactions, abiotic stress and development. Curr. Opin. Plant Biol. 8, 397–403. doi: 10.1016/j.pbi.2005.05.014
Tripathy, J. N., Zhang, J., Robin, S., Nguyen, T. T., and Nguyen, H. T. (2000). QTLs for cell-membrane stability mapped in rice (Oryza sativa L.) under drought stress. Theor. Appl. Genet. 100, 1197–1202. doi: 10.1007/s001220051424
Tuberosa, R., Frascaroli, E., Salvi, S., Sanguineti, M. C., Conti, S., and Landi, P. (2008). QTLS for tolerance to abiotic stresses in maize: Present status and prospects. Maydica 53, 559–569.
Turkan, I., Bor, M., Özdemir, F., and Koca, H. (2005). Differential responses of lipid peroxidation and antioxidants in the leaves of drought-tolerant P. acutifolius and drought-sensitive P. vulgaris subjected to polyethylene glycol mediated water stress. Plant Sci. 168, 223–231. doi: 10.1016/j.plantsci.2004.07.032
Tuteja, N., and Tuteja, R. (2001). Unravelling DNA repair in human: molecular mechanisms and consequences of repair defect. Crit. Rev. Biochem. Mol. Biol. 36, 261–290. doi: 10.1080/20014091074192
Upchurch, R. G. (2008). Fatty acid unsaturation, mobilization, and regulation in the response of plants to stress. Biotechnol. Lett. 30, 967–977. doi: 10.1007/s10529-008-9639-z
Valadabadi, S. A., and Farahani, H. A. (2010). Studying the interactive effect of potassium application and individual field crops on root penetration under drought condition. Asian J. Agric. Sci. 1, 19–24.
Valadabadi, S. A., Shiranirad, A. H., and Farahani, H. A. (2010). Ecophysiological influences of zeolite and selenium on water deficit stress tolerance in different rapeseed cultivars. J. Ecol. Nat. Environ. 2, 154–159.
Van den Ende, W., and Valluru, R. (2009). Sucrose, sucrosyl oligosaccharides, and oxidative stress: scavenging and salvaging? J. Exp. Bot. 60, 9–18. doi: 10.1093/jxb/ern297
Varshney, R. K., Kudapa, H., Roorkiwal, M., Thudi, M., Pandey, M. K., Saxena, R. K., et al. (2012). Advances in genetics and molecular breeding of three legume crops of semi-arid tropics using, next-generation sequencing and high-throughput genotyping technologies. J. Bio. Sci. 37, 811–820. doi: 10.1007/s12038-012-9228-0
Vasantha, S., Alarmelu, S., Hemaprabha, G., and Shanthi, R. M. (2005). Evaluation of promising sugarcane genotypes for drought. Sugar Technol. 7, 82–83. doi: 10.1007/BF02942536
Vendruscolo, E. C. G., Schuster, I., Pileggi, M., Scapim, C. A., Molinari, H. B. C., Marur, C. J., et al. (2007). Stress-induced synthesis of proline confers tolerance to water deficit in transgenic wheat. J. Plant Physiol. 164, 1367–1376. doi: 10.1016/j.jplph.2007.05.001
Verulkar, S. B., Mandal, N. P., Dwivedi, J. L., Singh, B. N., Sinha, P. K., Mahato, R. N., et al. (2010). Breeding resilient and productive genotypes adapted to drought-prone rainfed ecosystem of India. Field Crop. Res. 117, 197–208. doi: 10.1016/j.fcr.2010.03.005
Wahid, A., and Rasul, E. (2005). “Photosynthesis in leaf, stem, flower and fruit,” in Handbook of Photosynthesis, 2nd Edn, ed. M. Pessarakli (Boca Raton FL: CRC Press), 479–497.
Wang, B., Vinocur, O., and Altman, A. S. (2004). Role of plant heat-shock proteins and molecular chaperones in the abiotic stress response. Trend Plant Sci. 9, 244–252. doi: 10.1016/j.tplants.2004.03.006
Wang, W., Chen, Q., Hussain, S., Mei, J., Dong, H., Peng, S., et al. (2016). Pre-sowing seed treatments in direct-seeded early rice: consequences for emergence, seedling growth and associated metabolic events under chilling stress. Sci. Rep. 6:19637. doi: 10.1038/srep19637
Wang, Y., Hu, J., Qin, G., Cui, H., and Wang, Q. (2012). Salicylic acid analogues with biological activity may induce chilling tolerance of maize (Zea mays) seeds. Botany 90, 845–855. doi: 10.1139/b2012-055
Wang, Y., Wisniewski, M., Meilan, R., Cui, M., and Fuchigami, L. (2006). Transgenic tomato (Lycopersicon esculentum) overexpressing cAPX exhibits enhanced tolerance to UV-B and heat stress. J. Appl. Hortic. 8, 87–90.
Wang, Y., Wisniewski, M., Meilan, R., Cui, M., Webb, R., and Fuchigami, L. (2005). Overexpression of cytosolic ascorbate peroxidase in tomato confers tolerance to chilling and salt stress. J. Am. Soc. Hortic. Sci. 130, 167–173.
Wani, S. H., Kumar, V., Shriram, V., and Sah, S. K. (2016). Phytohormones and their metabolic engineering for abiotic stress tolerance in crop plants. Crop J. 4, 162–176. doi: 10.1016/j.cj.2016.01.010
Waraich, E. A., Ahmad, R., Ashraf, M. Y., Saifullah., and Ahmad, M. (2011a). Improving agricultural water use efficiency by nutrient management in crop plants. Acta Agric. Scand. Section B Plant Soil Sci. 61, 291–304.
Waraich, E. A., Ahmad, R., and Ashraf, M. Y. (2011b). Role of mineral nutrition in alleviation of drought stress in plants. Aust. J. Crop Sci. 5, 764–777.
Waraich, E. A., Ahmad, R., Halim, A., and Aziz, T. (2012). Alleviation of temperature stress by nutrient management in crop plants: a review. J. Soil Sci. Plant Nutr. 12, 221–244. doi: 10.4067/S0718-95162012000200003
Warrington, I. J., and Kanemasu, E. T. (1983). Corn growth response to temperature and photoperiod I. Seedling emergence, tassel initiation, and anthesis. Agron. J. 75, 749–754. doi: 10.2134/agronj1983.00021962007500050008x
Wendehenne, D., Pugin, A., Klessig, D. F., and Durner, J. (2001). Nitric oxide: comparative synthesis and signaling in animal and plant cells. Trend. Plant Sci. 6, 177–183. doi: 10.1016/S1360-1385(01)01893-3
Whaley, J. M., Kirby, E. J. M., Spink, J. H., Foulkes, M. J., and Sparkes, D. L. (2004). Frost damage to winter wheat in the UK: the effect of plant population density. Eur. J. Agron. 21, 105–115. doi: 10.1016/S1161-0301(03)00090-X
Wilkinson, S., Kudoyarova, G. R., Veselov, D. S., Arkhipova, T. N., and Davies, W. J. (2012). Plant hormone interactions: innovative targets for crop breeding and management. J. Exp. Bot. 63, 3499–3509. doi: 10.1093/jxb/ers148
Wise, R. R. (1995). Chilling-enhanced photooxidation: the production, action and study of reactive oxygen species produced during chilling in the light. Photosyn. Res. 45, 79–97. doi: 10.1007/BF00032579
Wullschleger, S. D., Yin, T. M., DiFazio, S. P., Tschaplinski, T. J., Gunter, L. E., Davis, M. F., et al. (2005). Phenotypic variation in growth and biomass distribution for two advanced-generation pedigrees of hybrid poplar. Can. J. For. Res. 35, 1779–1789. doi: 10.1139/x05-101
Xiao, B., Huang, Y., Tang, N., and Xiong, L. (2007). Overexpression of a LEA gene in rice improves drought resistance under the field conditions. Theor. Appl. Genet. 115, 35–46. doi: 10.1007/s00122-007-0538-9
Xin, Z., and Li, P. H. (1992). Abscisic acid-induced chilling tolerance in maize suspension-cultured cells. Plant Physiol. 99, 707–711. doi: 10.1104/pp.99.2.707
Xing, W., and Rajashekar, C. B. (2001). Glycinebetaine involvement in freezing tolerance and water stress is Arabidopsis thaliana. Environ. Exp. Bot. 46, 21–28. doi: 10.1016/S0098-8472(01)00078-8
Xiong, L., Wang, R., Mao, G., and Koczan, J. M. (2006). Identification of drought tolerance determinants by genetic analysis of root response to drought stress and abscisic acid. Plant Physiol. 142, 1065–1074. doi: 10.1104/pp.106.084632
Xu, Q., and Huang, B. (2006). Seasonal changes in root metabolic activity and nitrogen uptake for two cultivars of creeping bentgrass. Hortic. Sci. 41, 822–826.
Xu, S., Hu, J., Li, Y., Ma, W., Zheng, Y., and Zhu, S. (2011). Chilling tolerance in Nicotiana tabacum induced by seed priming with putrescine. Plant Growth Regul. 63, 279–290. doi: 10.1007/s10725-010-9528-z
Yadav, S. K. (2010). Cold stress tolerance mechanisms in plants. A review. Agron. Sustain. Dev. 30, 515–527. doi: 10.1051/agro/2009050
Yamada, M., Morishita, H., Urano, K., Shiozaki, N., Yamaguchi-Shinozaki, K., Shinozaki, K., et al. (2005). Effects of free proline accumulation in petunias under drought stress. J. Exp. Bot. 56, 1975–1981. doi: 10.1093/jxb/eri195
Yamaguchi-Shinozaki, K., and Shinozaki, K. (1994). A novel cis-acting element in an Arabidopsis gene is involved in responsiveness to drought, low-temperature, or high-salt stress. Plant Cell 6, 251–264. doi: 10.1105/tpc.6.2.251
Yamaguchi-Shinozaki, K., and Shinozaki, K. (2005). Organization of cisacting regulatory elements in osmotic- and cold-stress-responsive promoters. Trend Plant Sci. 10, 88–94. doi: 10.1016/j.tplants.2004.12.012
Yan, J., Wang, J., Tissue, D., Holaday, A. S., Allen, R., and Zhang, H. (2003). Photosynthesis and seed production under water-deficit conditions in transgenic tobacco plants that overexpress an ascorbate peroxidase gene. Crop Sci. 43, 1477–1483. doi: 10.2135/cropsci2003.1477
Yan, Q., Duan, Z., Jingdong, M., Xun, L., and Fei, D. (2012). Effects of root-zone temperature and N, P, and K supplies on nutrient uptake of cucumber (Cucumis sativus L.) seedlings in hydroponics. Soil Sci. Plant Nutr. 58, 707–717. doi: 10.1080/00380768.2012.733925
Yang, S., Vanderbeld, B., Wan, J., and Huang, Y. (2010). Narrowing down the targets: towards successful genetic engineering of drought-tolerant crops. Mol. Plant 3, 469–490. doi: 10.1093/mp/ssq016
Ye, C., Fukai, S., Godwin, I., Reinke, R. B., Snell, P. B., Schiller, J., et al. (2009). Cold tolerance in rice varieties at different growth stages. Crop Past. Sci. 60, 328–338. doi: 10.1071/CP09006
Yokoi, S., Quintero, F. J., Cubero, B., Ruiz, M. T., Bressan, R. A., Hasegawa, P. M., et al. (2002). Differential expression and function of Arabidopsis thaliana NHX Na+ /H+ antiporters in the salt stress response. Plant J. 30, 529–539. doi: 10.1046/j.1365-313X.2002.01309.x
Zhang, J., Cui, S., Li, J., Wei, J., and Kirkham, M. B. (1995). Protoplasmic factors, antioxidant responses, and chilling resistance in maize. Plant Physiol. Biochem. 33, 567–575.
Zhang, J., Nguyen, H. T., and Blum, A. (1999). Genetic analysis of osmotic adjustment in crop plants. J. Exp. Bot. 50, 291–302. doi: 10.1093/jxb/50.332.291
Zhang, J. Z., Creelman, R. A., and Zhu, J. K. (2004). From laboratory to field. Using information from Arabidopsis to engineer salt, cold and drought tolerance in crops. Plant Physiol. 135, 615–621. doi: 10.1104/pp.104.040295
Zhang, M., Duan, L., Zhai, Z., Li, J., Tian, X., Wang, B., et al. (2004). “Effects of plant growth regulators on water deficit-induced yield loss in soybean,” in Proceedings of the 4th International Crop Science Congress, Brisbane, QLD.
Zhang, Q., Li, J., Zhang, W., Yan, S., Wang, R., Zhao, J., et al. (2012). The putative auxin efflux carrier ospin3t is involved in the drought stress response and drought tolerance. Plant J. 72, 805–816. doi: 10.1111/j.1365-313X.2012.05121.x
Zhao, F. Y., Wang, X. Y., Zhao, Y. X., and Zhang, H. (2006). Transferring the Suaeda salsa glutathione S-transferase and catalase genes enhances low temperature stress resistance in transgenic rice seedlings. J. Plant Physiol. Mol. Biol. 32, 231–238.
Zhao, J., Li, S., Jiang, T., Liu, Z., and Zhang, W. (2012). Chilling stress- the key predisposing factor for causing Alternaria alternata infection and leading to cotton (Gossypium hirsutum L.) leaf senescence. PLoS One 7:e36126. doi: 10.1371/journal.pone.0036126
Zhao, M., Liu, W., Xia, X., Wang, T., and Zhang, W. H. (2014). Cold acclimation-induced freezing tolerance of Medicago truncatula seedlings is negatively regulated by ethylene. Physiol. Plant. 152, 115–129. doi: 10.1111/ppl.12161
Zheng, M., Tao, Y., Hussain, S., Jiang, Q., Peng, S., Huang, J., et al. (2016). Seed priming in dry direct-seeded rice: consequences for emergence, seedling growth and associated metabolic events under drought stress. Plant Growth Regul. 78, 167–178. doi: 10.1007/s10725-015-0083-5
Zhou, B., Guo, Z., Xing, J., and Huang, B. (2005). Nitric oxide is involved in abscisic acid-induced antioxidant activities in Stylosanthes guianensis. J. Exp. Bot. 56, 3223–3228. doi: 10.1093/jxb/eri319
Keywords: climate change, chilling, drought, plant responses, stress management
Citation: Hussain HA, Hussain S, Khaliq A, Ashraf U, Anjum SA, Men S and Wang L (2018) Chilling and Drought Stresses in Crop Plants: Implications, Cross Talk, and Potential Management Opportunities. Front. Plant Sci. 9:393. doi: 10.3389/fpls.2018.00393
Received: 08 January 2018; Accepted: 12 March 2018;
Published: 10 April 2018.
Edited by:
Rosa M. Rivero, Centro de Edafología y Biología Aplicada del Segura (CSIC), SpainReviewed by:
Nobuhiro Suzuki, Sophia University, JapanCristina Nali, Università degli Studi di Pisa, Italy
Copyright © 2018 Hussain, Hussain, Khaliq, Ashraf, Anjum, Men and Wang. This is an open-access article distributed under the terms of the Creative Commons Attribution License (CC BY). The use, distribution or reproduction in other forums is permitted, provided the original author(s) and the copyright owner are credited and that the original publication in this journal is cited, in accordance with accepted academic practice. No use, distribution or reproduction is permitted which does not comply with these terms.
*Correspondence: Longchang Wang, wanglc@swu.edu.cn
†These authors have contributed equally to this work.