Effects of a One-Time Organic Fertilizer Application on Long-Term Crop and Residue Yields, and Soil Quality Measurements Using Biointensive Agriculture
- 1Soil and Crop Sciences, Cornell University, Ithaca, NY, United States
- 2Agroecology, Elon University, Elon, NC, United States
- 3Department of Mathematics and Statistics, Elon University, Elon, NC, United States
- 4Co-Executive Director, Grow Biointensive Agricultural Center of Kenya (G-BIACK), Thika, Kenya
Background: Closing the yield gap, especially in Africa, using environmentally appropriate and sustainable methods is important to meet current and future food demands. Grow Biointensive Sustainable Agriculture (GBSA) is an organic method that combines eight sustainable principles that may close the yield gap, increase food production, improve soil quality, and reduce soil nutrient loss.
Method: Utilizing the GBSA method, four Kenyan farms over 4 years tested the effect of a one-time application of organic fertilizers (recommended based on soil testing) on crop and residue yields and soil parameter levels.
Results: Yearly crop edible yields increased overall for maize, sorghum, and sweet potatoes. Amaranth showed no significant change. Yearly crop residue yields increased for sweet potatoes and increased marginally for amaranth, maize, and sorghum. Harvesting practice changes in cowpeas and lablab made interpretation more complex, but both edible and residue yields for these crops varied from unchanged to significantly increased. Fourteen soil parameters were tested. Boron, electrical conductivity, magnesium, organic matter, phosphorus, potassium, sulfur, and zinc increased significantly. Calcium, copper, iron, pH, and sodium showed no significant changes, and only manganese levels decreased significantly.
Conclusion: Combining a single application of recommended organic fertilizers and the GBSA method, farmers may be able to improve their crop yields and maintain soil fertility in a manner that is more sustainable.
Introduction
Global food systems face two main challenges: (1) feeding a growing population with increasing consumption levels of calories and animal products (Bodirsky et al., 2015; Sans and Combris, 2015); and (2) supporting ecosystem services, restoring natural resources degraded by unsustainable agricultural practices, and staying within safe operating biophysical boundaries (Rockström et al., 2009, 2015; Godfray et al., 2010; Foley et al., 2011).
Africa faces these challenges in unique and demanding ways. Forty percent of children under the age of five in Sub-Saharan Africa (SSA) are stunted from malnutrition (Montpellier Panel Report, 2013) and 23% of the African population are classified as hungry (FAO, 2012). The projected doubling in population size by 2050 (United Nations Department of Economic Social Affairs, 2010) and increased caloric and animal product demand (Roxburgh et al., 2011) place increased burdens on food production systems. To address these food needs within the constraints of sustainable development such as limited water, energy and land availability, and biodiversity loss adds additional challenges (Foley et al., 2011; Montpellier Panel Report, 2013). Meeting these goals within the context of food sovereignty is increasingly important to improve food security given the challenges described above (Declaration of Nyeleni, 2007).
Modern conventional food production shows signs of production stagnation, resource depletion, and stress on the environment (Stocking, 2003; Moore, 2010). Common African low-input agricultural practices have proven detrimental to soil health and exhibit low yields (Akinnifesi et al., 2010). To further exacerbate these concerns, in SSA, climate change is expected to have negative impacts on future food security (IPCC, 2007; Cairns et al., 2013; Liu et al., 2013; Folberg et al., 2014).
Several alternative systems of food production can reduce negative environmental impacts while supporting ecosystems services. These more natural production systems include organic (Reganold and Wachter, 2016; Muller et al., 2017), agroecological (Silici, 2014), sustainable, conservation agriculture (Brouder and Gomez-Macpherson, 2014), Sustainable Intensification (SI) (Montpellier Panel Report, 2013), and Grow Biointensive Sustainable Agriculture (GBSA) (Jeavons, 2001). Many of these alternative systems have been both proposed as solutions and challenged by claims that they are not viable options to feed the world (Badgley and Perfecto, 2007; Seufert et al., 2012). In addition, they may still have negative environmental impacts (Kirchmann and Bergstrom, 2001; Seufert et al., 2012).
In particular, SI has been proposed and supported as a potential solution to these challenges by major international development organizations (Godfray et al., 2010; Montpellier Panel Report, 2013; Prasad et al., 2016; World Bank, 2018). Yet, the term has a clouded meaning (Petersen and Snapp, 2015) and can have negative environmental consequences if increased application rates of chemical-based fertilizers are used (Tilman et al., 2011). For many, the promotion and usage of chemical-based fertilizers in SI compromises the term “sustainable” (Petersen and Snapp, 2015; Mahon et al., 2018). Pretty et al. (2011) emphasizes the need for both increased productivity and decreased negative environmental impact in his definition: “Sustainable agricultural intensification is defined as producing more output from the same area of land while reducing the negative environmental impacts and at the same time increasing contributions to natural capital and the flow of environmental services.” This definition is in line with an agroecology principle to minimize use of or eliminate external inputs (Gliessman, 1990).
One method to attain the intensification goals, especially those articulated by Pretty et al. (2011), is GBSA. Eight principles are combined and work synergistically in GBSA to improve yields and soil fertility, meet human nutritional needs, reduce external input dependence, support ecosystem services, and address food sovereignty (Jeavons, 2001; Bomford, 2009; Moore, 2010; Rajbhsndari, 2011; Omondi et al., 2014).
The first three principles focus on soil building and water and nutrient cycling.
Principle 1—Build and maintain deep soil quality (to 60 cm in depth) supported by permanent pathways and beds.
Principle 2—Design for and grow soil building carbon and nitrogen crops.
Principle 3—Efficiently produce compost to optimize soil biological activity and reduce system nutrient loss.
The next two principles work to achieve higher yields through intensive plant spacing, multi-cropping, using transplants to improve soil coverage, and leveraging allelopathic relationships.
Principle 4–Plant intensification.
Principle 5–Functional biodiversity.
The next two principles address human nutritional needs.
Principle 6–Design and produce nutritionally complete human diets.
Principle 7–Achieve seed sovereignty primarily through open pollinated varieties that are locally sourced and bred to meet local cultural demands, and yield improvements, pest resistance, and climate adaptability, creating a resilient system.
The last principle brings all of the principles together into a cohesive production system.
Principle 8–Use all principles to achieve as sustainable a food production system as possible, which relies minimally on inputs of water, energy, and land use to meet human needs and reduce demands on and support ecosystem services (Jeavons, 2001).
GBSA has shown a high positive energy efficiency ratio primarily from renewable energy sources (Moore, 2010). Reducing land requirements via high yields has multiple advantages. They include increased land tenure, increased urban and peri-urban production and reduced need for agriculture expansion into natural ecosystems as production demands increase.
Much has been written about the significance of reducing the yield gap with inorganic and/or organic amendments, but there are also challenges with the use of these amendments (Epule et al., 2015). Synthetic and organic fertilizers can be expensive and difficult to acquire. They carry environmental costs in their production, transportation, and application. In addition, synthetic and organic fertilizers can reduce food sovereignty by creating dependency on external or imported inputs. Many cite that only inorganic fertilizers can fully reduce the yield gap (Epule et al., 2015). Epule argues that organic fertility sources cannot sustain yields and are not sufficient for a new African agricultural revolution.
GBSA may offer a holistic approach to the “feed people and save the planet” conundrum. This system is especially appropriate for smallholder farms. Globally, 84% of farms are ≤2 ha, with 72% of them ≤1 ha (Lowder et al., 2016). FAO data indicate that 1.5 billion people who live in smallholder households in low-income countries provide food for 2.5 billion people (FAO, 2012), highlighting their potential to impact food supplies.
In Africa and in other demonstration plots, GBSA has shown the capacity to improve crop yields sustainably and increase overall food sovereignty (Jeavons, 2001; Rajbhsndari, 2011; Omondi et al., 2014). GBSA is designed to maximize yields and reduce the area needed to produce a complete and sustainable diet, while relying on few if any inputs sourced beyond the farm itself. With GBSA's emphasis on “closed-loop” fertility to minimize losses of soil nutrients and the reduced need for annual inputs of fertilizers, this method of agriculture is appropriate for the large diversity of smallholder farms (Murphy, 2017).
This experiment was designed to test the effect of a one-time application of organic fertilizers using GBSA practices on soil fertility and crop and residue yields over a 4-years period at four locations in Kenya.
Methods and Materials
Farm Selection
Four Kenyan GBSA farmers participated in this study, including and led by Samuel Nderitu, a founder and director of the Grow Biointensive Agricultural Center of Kenya (G-BIACK) and a prominent leader, teacher, and practitioner with 13 years of experience in GBSA. The four participating farms were located in the Central Province of Kenya. Farm characteristics are shown in Table 1. The farms had an average elevation of 1,488 ± 38 m.
Experiment Method
Each farm created 10 new beds, each 1.52 m by 6.1 m, or 9.3 m2 (100 ft2), surrounded by a 45-cm path. Deep cultivation was achieved using the double-digging method (Jeavons, 2001, 2017). For each of the 4 years, from 2012 to 2015, soil from each of the beds was sampled (see Supplemental Materials-Soil Sampling) annually prior to cultivation and sent to Crop Nutritional Laboratory Services (cropnuts.com, Mashiara Park, Kaptagat Road, Loresho, Nairobi, Kenya) for analysis. Crop Nutritional Laboratory Services was selected as the analytical lab due to its ISO-17025 accreditation status, location, and recommendation by John Recha, Participatory Action Research Specialist, CGIAR Research Program on Climate Change, Agriculture and Food Security (CCAFS). All extractions were done by Mehlich III. B, Ca, Cu, Fe, Mg, Mn, P, K, Na, S, and Zn levels were determined by inductively coupled plasma spectroscopy (ICP), pH and EC were determined potentiometrically, and organic matter was determined colorimetrically. No additional soil properties were tested.
The initial soil test results were reviewed by Timberleaf Soil Testing (timberleafsoiltesting.org, 39648 Old Spring Rd., Murrieta, CA 92563) and recommendations for the application of organic fertilizers based on soil test results (Table 2) were finalized by Grow Your Soil (growyoursoil.org, P.O. Box 4095, Ithaca, NY 14852).
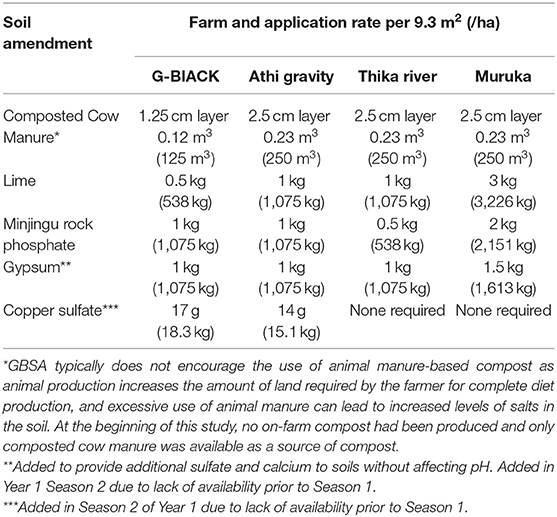
Table 2. Application rates of fertilizer applied per site prior to Season 1 of first year unless otherwise specified.
Immediately prior to the planting of the first season crops, the recommended organic fertilizers and compost were added. To the acidic soils, lime was added first and the additional recommended fertilizers and compost were added 1 month later. Gypsum and/or copper sulfate, if needed, were added prior to the second season planting due to difficulty in acquiring them prior to the first season planting. After the inputs were applied, crops were immediately planted using close, offset spacing as recommended by GBSA (Jeavons, 2017) (Table 3). In the first season of the first year, each farm grew four beds of maize, two beds of sorghum, and one bed each of amaranth, cowpeas, lablab, and sweet potatoes (Table 3). For all subsequent seasons and years, each farm grew three beds of maize, two beds of sorghum, two beds of amaranth, and one bed each of cowpeas, lablab, and sweet potatoes.
Crop selection was based on GBSA's goal to maximize nutritional and crop residue production. The intent of producing maize, sorghum, and amaranth was to produce not only crops that generate familiar foods but also compost material to maintain and increase soil organic matter levels. Maize provides 65% of the total staple food caloric intake and 36% of the total food caloric intake in Kenya (Mohajan, 2014). All crops were rotated the same at all four sites, and crop rotation was done so that no bed grew the same crop in consecutive seasons, with the exception of sorghum planted as a ratoon crop (see below). No supplementary irrigation was applied to the crops at any of the four farms during this 4-years study. GBSA lowers irrigation demand through close plant spacing to reduce evaporation (Wallace, 2000; Rockström, 2003), increased soil organic matter for water retention (Magdof and Van Es, 2009; Hatfield and Morton, 2013; Weil and Brady, 2017), and deep soil structure to increase water infiltration and water holding capacity (Baig et al., 1999, 2013).
After this initial one-time organic fertilizer application, all four farmers managed their 10 beds using GBSA to retain and recycle as much of the soil nutrients as possible. No more than 10% of the crop was sold off the farm; the remainder was consumed on the farm, and all crop residues were efficiently composted on site and returned to the soil. In this study, we used all of the crop residues to create GBSA compost piles by adding 2 volumes of brown (stovers, straw, etc.) material, 2 volumes of green (fresh cut plant material), and 1/4 volume of soil in order to create an initial carbon to nitrogen ratio ranging from 30 to 45:1. GBSA compost is generally turned over only once to encourage slower composting to retain more nitrogen and carbon and generate more overall cured compost (Haug, 1993; Larsen and McCartney, 2000; Eiland et al., 2001; Tiquia et al., 2002; Zhang et al., 2020). This results in fungal-based compost that retains many of the original nutrients (Jeavons, 2001; Barrington et al., 2002; Man et al., 2015; WSU, 2019). The cured compost that was generated was not tested prior to being added back to these soils. Since the compost is composed only of crops grown on these soils, we would not expect substantial additional nutrients to be added beyond what the soil already contained.
Crops were harvested manually and immediately weighed, and yields were recorded. In this experiment, sorghum was a ratoon crop in all sites for Years 2, 3, and 4, meaning that after the first year's planting, the sorghum was allowed to regrow rather than being replanted. Results for lablab and cowpeas were impacted by changes in harvesting practices during the course of the study (see Results and Discussion sections for details).
Statistical Methods
Yield measurements and residue were both modeled using three-way fixed-effects ANOVA with season, site, and year as explanatory variables. When warranted, an interaction between year and season was included. Analysis of covariances (ANCOVA) was used to model the soil parameter levels based on site and year. The equal slopes model was deemed appropriate for all parameters. A Type I Error rate of α = 0.05 was used for each test and confidence. Analysis was conducted using SAS 9.4, and graphs were generated using R 3.4.4.
Results
Edible Yield
Edible yields are summarized by year, season, and site in Figure 1 and Table 4. There are n = 32 overall yield measurements of each crop: 4 years, 2 seasons, and 4 sites. Yearly edible yields increased for maize, sorghum, and sweet potatoes. Amaranth and cowpea yields did not significantly change, and lablab yields increased through the second season of Year 3 when harvesting practices were changed. For crop, year, season, and site data, see Figure 1 and Supplemental Materials: Edible Yield, Residue Yield, and Cured Compost Added.
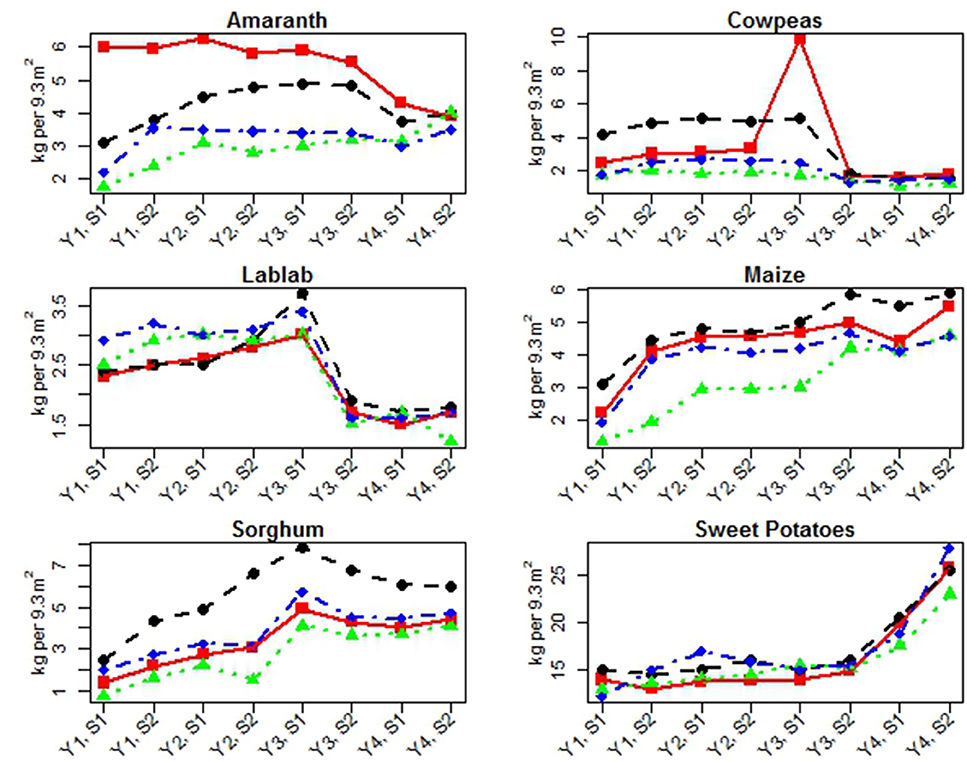
Figure 1. Edible yields for each crop by site (harvest weight). Yields are reported in kilograms per 9.3 m2 (100 ft2) per year (Y) and season (S). Lines indicate sites: Athi Gravity (red square), G-Biack (black circle), Muruka (green triangle), and Thika River (blue diamond).
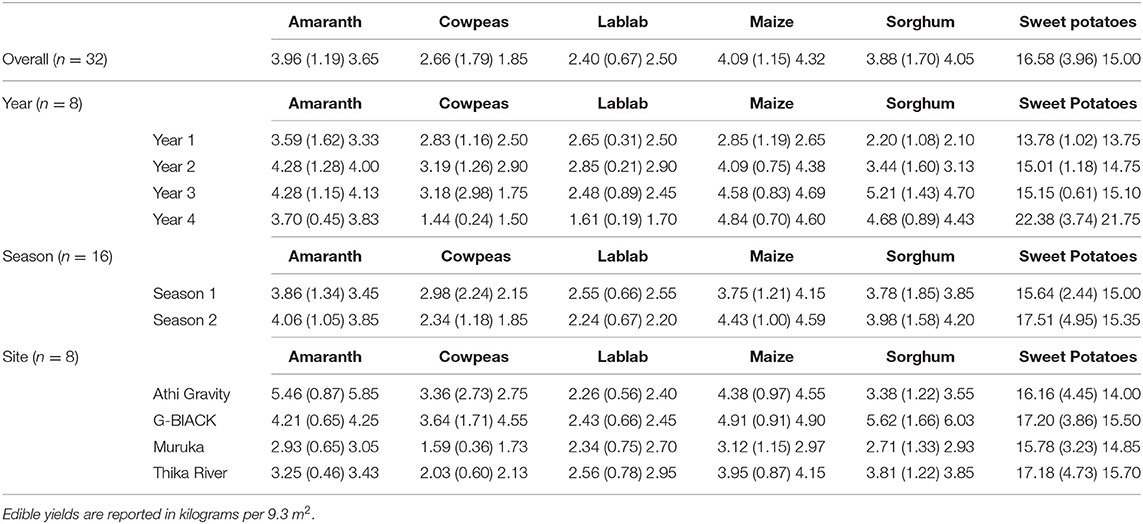
Table 4. Edible Yields—Means, standard deviations, in parenthesis, and medians of edible yields for each crop based on overall experiment, year, season, and site.
Statistical Analysis of Edible Yields
A three-way fixed-effects ANOVA was conducted to compare the yields for each of the six crops based on main effects of year, season, and site (Table 4). Years were quantitatively coded as 1, 2, 3, and 4 for the 4 years of the experiment; seasons were quantitatively coded as 1 and 2 for the first and second growing seasons of each year. No significant pairwise or three-way interactions were found for any crops so only the models with main effects were considered. Harvesting practices for cowpeas and lablab were changed starting with the second season of year three in order to favor residue yields over edible yields, so these crops were modeled separately for the harvest period favoring edible yields (HE) and for the harvesting period favoring residue yields (HR). Further, a model to evaluate changes in yield based on harvesting practice was fitted for cowpeas and lablab that also controlled for differences in site, year, and season.
Yearly comparison—Significant increases in yield (positive slopes or β values) over the time period of this study were found for maize, sweet potatoes, and sorghum {respectively [β = 0.64, t(26) = 8.04, p < 0.0001], [β = 2.59, t(26) = 6.31, p < 0.0001], [β = 0.92, t(26) = 6.98, p < 0.0001]}. Amaranth yields did not significantly change during the study [β = 0.03, t(26) = 0.29, p = 0.7720]. Cowpea yields did not significantly change for either harvesting practice [HE: β = 0.932, t(14) = 1.96, p = 0.0702, HR: β = −0.05, t(6) = −0.51, p = 0.6278]. Cowpea yield did significantly differ between the two harvest periods (HE and HR) by 3.18 kg/9.3 m2 more, on average, during HE (95% CI for Diff = HE – HR: 0.84, 5.53). Lablab yields significantly increased during the initial harvesting practice [HE: β = 0.33, t(14) = 4.73, p = 0.0003], but lablab yields did not change significantly during the remainder of the study [HR: β = −0.075, t(6) = −0.63, p = 0.5499]. Lablab yields were significantly greater during the harvesting period favoring edible yields by an average of 1.65 kg/9.3 m2 more during HE (95% CI for Diff = HE – HR: 1.23, 2.08).
Seasonal comparison—Maize yields were significantly affected by the season in which they were grown [β = 0.68, t(26) = 3.80, p = 0.0008], and sweet potato yields were marginally affected [β = 1.89, t(26) = 2.03, p = 0.0523], likely due to precipitation differences between the two seasons (no additional irrigation was provided). Amaranth, lablab, sorghum, and cowpea crop yields were not significantly affected by season.
Site comparison—Maize, sorghum, amaranth, cowpea (during HE and HR periods), and lablab (during HE period) yields were also significantly different between sites {respectively, [F(3, 26) = 17.76, p < 0.0001], [F(3, 26) = 17.82, p < 0.0001], [F(3, 26) = 21.81, p < 0.0001], [HE: F(3, 14) = 4.91, p = 0.0155; HR: F(3, 6) = 7.70, p = 0.0176], [HE: F(3, 14) = 4.08, p = 0.0281]}. There was no significant site effect detected for lablab during HR period [HR: F(3, 6) = 1.98, p = 0.2184] or sweet potato [F(3, 26) = 0.62, p = 0.6107].
A summary of three-way analysis of variance on crop edible yields for each crop can be found in Supplemental Materials: Summary of Three-way Analysis of Variance on Crop Yield (ANOVA).
Residue Yield (Aboveground Non-edible Biomass)
Crop residue yields in this study are summarized by year, season, and site in Figure 2 and Table 5. Since no residue yields were recorded in the first season of Year 1, the overall number of observations for each crop was n = 28. Yearly crop residue yields increased for sweet potatoes, increased marginally for amaranth, maize, and sorghum for some seasons, and marginally increased during some time periods for cowpeas and lablab. For crop, year, season, and site data, see Figure 2 and Supplemental Materials: Crop Residue Yield Analysis SAS Output.
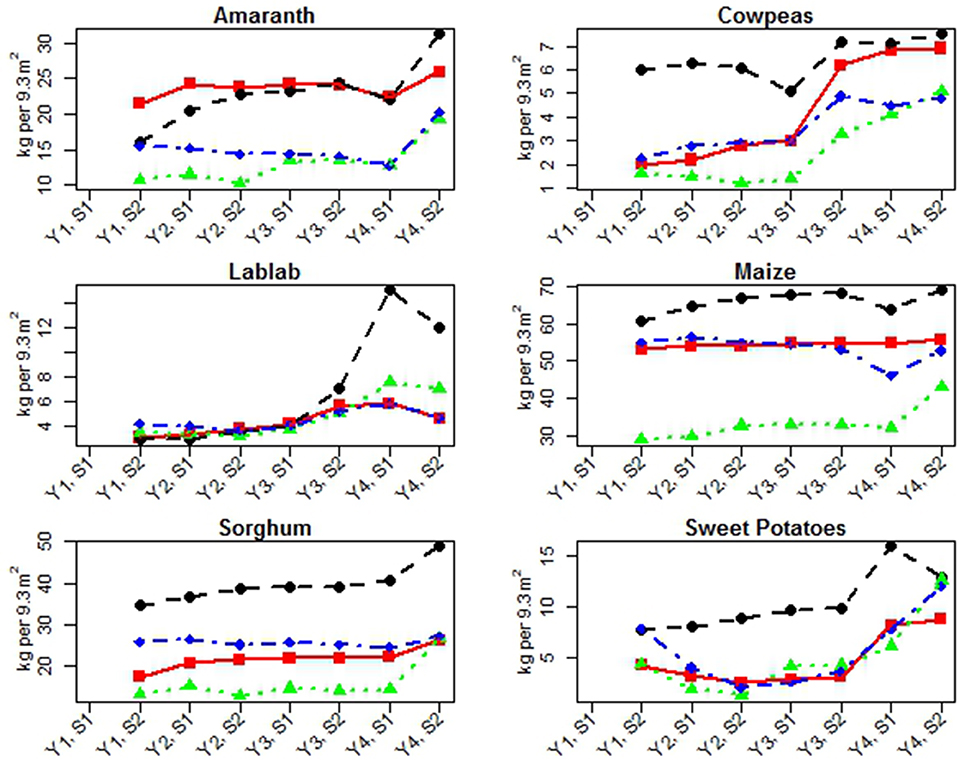
Figure 2. Residue yields for each crop (harvest weight) by site reported in kilograms per 9.3 m2 (100 ft2) per year (Y) and season (S). Lines indicate sites: Athi Gravity (red square), G-Biack (black circle), Muruka (green triangle), and Thika River (blue diamond).
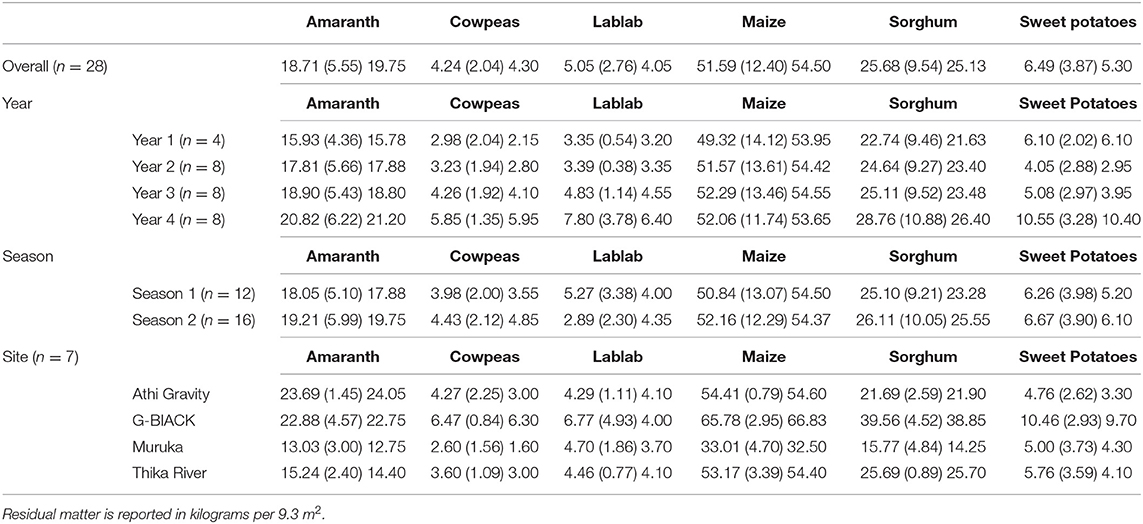
Table 5. Residual Matter—Means, standard deviations, in parenthesis, and median of residual matter for each crop based on overall experiment, year, season, and site.
Statistical Analysis of Crop Residue Yields
A full model including all pairwise interactions and the three-way interaction was used to determine whether to include interaction terms for amaranth, maize, sorghum, and sweet potatoes. Based on this analysis, three-way ANOVA models with a year by season interaction were fit for maize, sorghum, and amaranth only. Only main effects were considered for sweet potatoes. Due to changes in harvesting practices for cowpeas and lablab (see Discussion section for details), similar analyses were performed for residue yields of cowpeas and lablab as described in the Edible Yields section of the Results. With the reduced degrees of freedom, a model with main effects only was fit for cowpeas and lablab for both the HE and HR periods. Further, a model to evaluate changes in yield based on harvesting practice was fit for cowpeas and lablab that also controlled for differences in site, year, and season. See Supplemental Materials: Summary of Three-way Analysis of Variance on Crop Yield (ANOVA).
Yearly comparison—Residue yields of sweet potatoes [β = 2.02, t(22) = 4.27, p = 0.0003] increased significantly as indicated by a positive slope estimate associated with year (β) over the years of this study in the models, which also accounted for the main effects of season and site. Marginally significant main effects of year on the residue yields of maize [F(1, 21) = 3.13, p = 0.0914] and amaranth [F(1, 21) = 4.02, p = 0.0580] were detected with the presence of significant year by season interactions {[F(1, 21) = 5.23, p = 0.0327], [F(1, 21) = 11.13, p = 0.0031], respectively}, which suggested that year effects varied negatively in Season 1 and positively in Season 2. Sorghum did not have a significant change in residue yield based on the main effect of year in the presence of a significant interaction between year and season [F(1, 21) = 5.76, p = 0.0258], which suggested positive overall year effects for both seasons. Cowpea residue yields did not change significantly during the HE period [HE: β = 0.10, t(14) = 0.46, p = 0.6523] and increased marginally during the HR period [HR: β = 0.68, t(6) = 2.19, p = 0.0712]. Cowpea residue yields did significantly differ between the two harvesting periods by 2.03 kg/9.3 m2 more, on average, during HR (95% CI for Diff = HR – HE: 0.83, 3.23). Lablab residue yields increased marginally during the HE period [HE: β = 0.39, t(14) = 2.19, p = 0.0536] and showed no significant change during the HR period [HR: β = 1.35, t(6) = 1.01, p = 0.3504]. Lablab residue yields marginally differed between the two harvest periods by an average of 2.99 kg/9.3 m2 more during HR (95% CI for Diff = HR – HE: −0.33, 6.32).
Seasonal comparison—Amaranth residue yields were also significantly associated with season [F(1, 21) = 5.49, p = 0.0290] in the presence of a significant interaction with year. No significant season effect was detected for cowpeas, lablab, maize, sorghum, lablab, or sweet potatoes residue yield.
Site comparison—With the exception of lablab during the HE period, there was convincing evidence of a site effect on residue yield across the other five crops (p < 0.01 for all).
A summary of three-way analysis of variance on crop residue yields for each crop can be found in Supplemental Materials: Summary of Three-way Analysis of Variance on Crop Residue Yield (ANOVA).
Soil Parameter Responses
Levels of 14 soil parameters were measured at each site over 4 years. Boron, electrical conductivity, magnesium, organic matter, phosphorus, potassium, sulfur, and zinc increased significantly. Calcium, copper, iron, pH, and sodium showed no significant changes, and manganese levels decreased significantly. See Figure 3 and Table 6 for site and year data. Optimal ranges of soil parameters can be seen in Supplemental Materials: Ideal Soil Parameter Ranges.
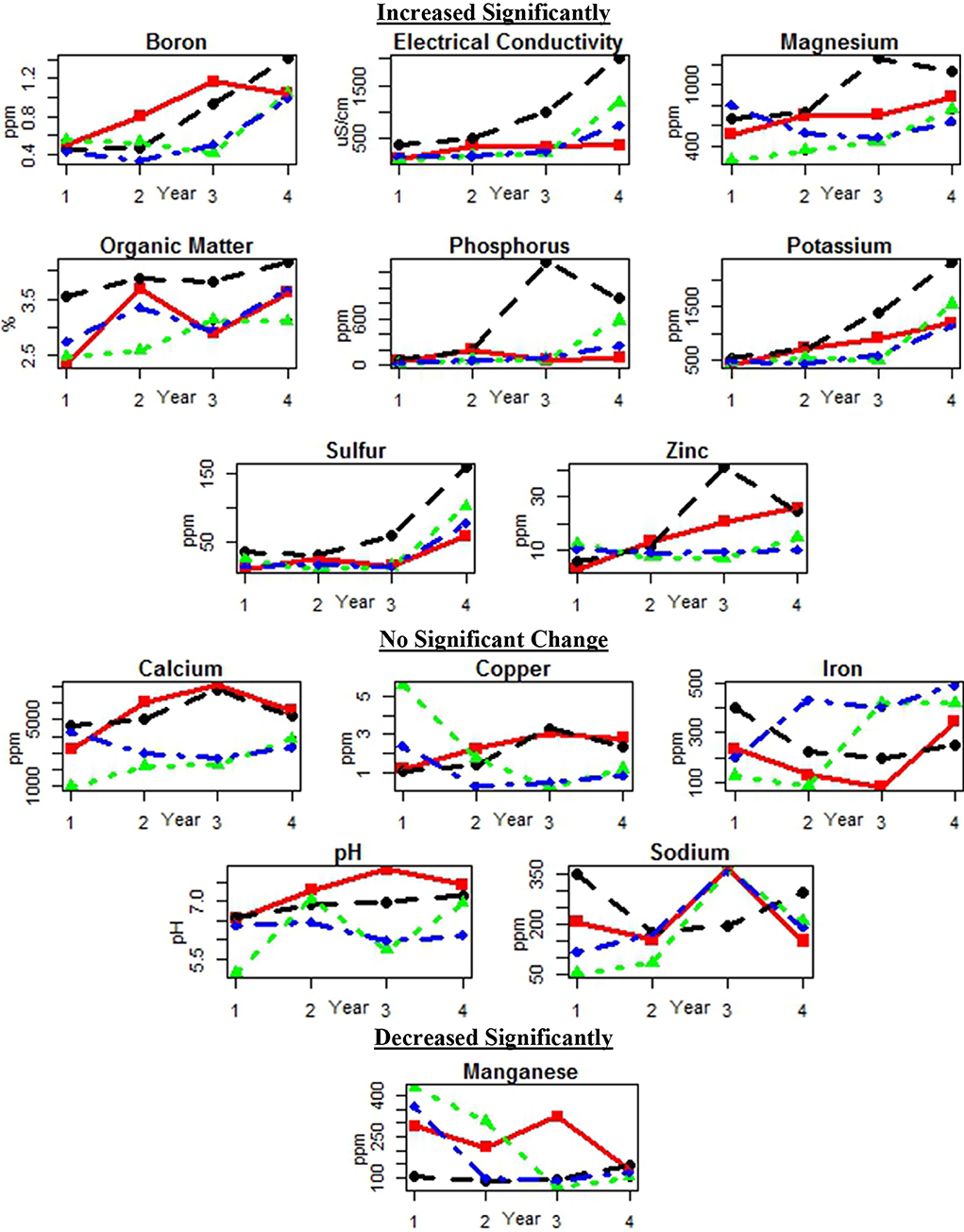
Figure 3. Soil parameters for each site by year categorized by statistical increase, no change, and decrease. Lines indicate sites: Athi Gravity (red square), G-BIACK (black circle), Muruka (green triangle), and Thika River (blue diamond).
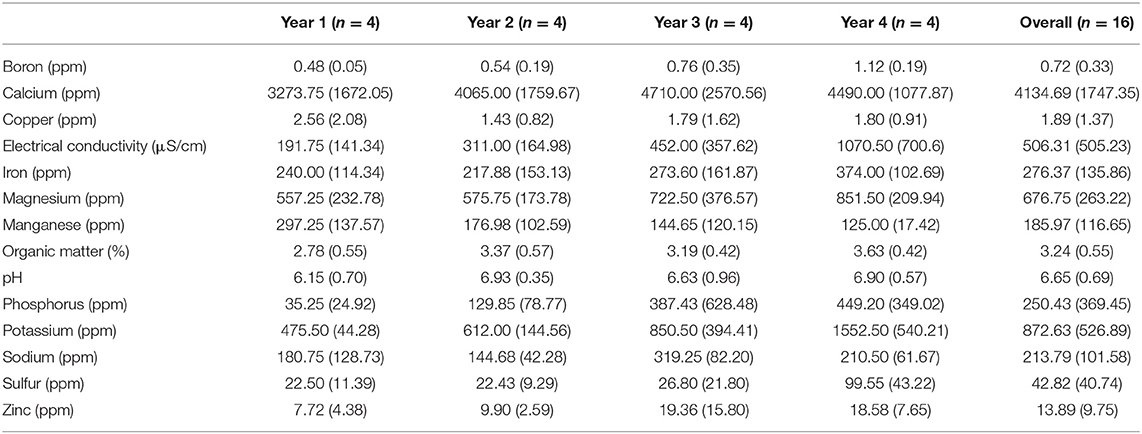
Table 6. Mean and standard deviation, in parenthesis, of soil parameter levels for each year and across the entire study.
Statistical Analysis of Soil Parameter Responses
For each of the soil parameters, ANCOVA was used to model soil parameter levels based on differences in site and year. At the α = 0.05 level, the equal slopes model was deemed appropriate for all nutrients.
Significant positive yearly increases in boron, electrical conductivity, magnesium, organic matter, phosphorus, potassium, sulfur, and zinc were detected (Table 7). Significantly decreasing levels were detected for manganese, and no significant change was detected for calcium, copper, iron, pH, and sodium (Table 7). Full model details are available in the Supplemental Materials: Soil Parameter Analysis SAS Output.
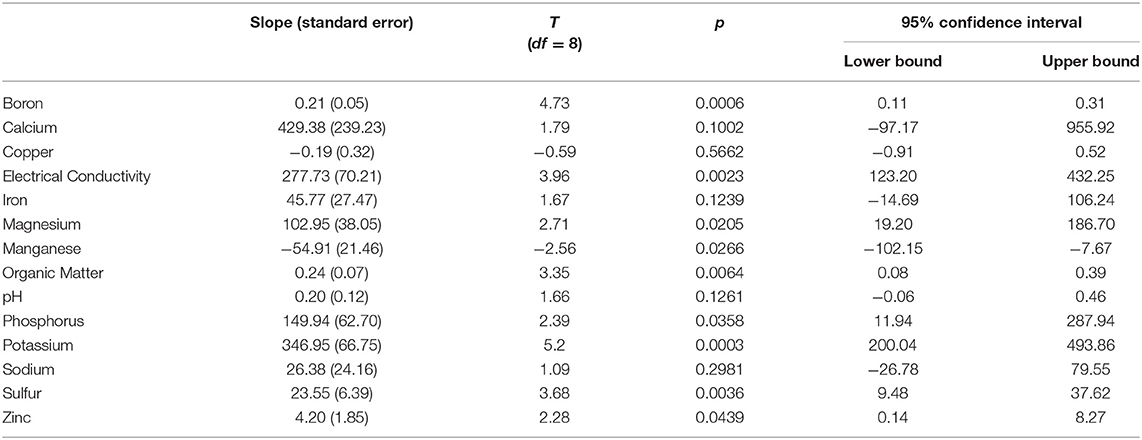
Table 7. Slopes and standard error, in parenthesis, associated with equal slopes models for each soil parameter, test statistics, two-sided p values, and 95% confidence intervals.
Discussion
Edible Yields
Edible yields of many of the crops increased from Year 1 and continued to increase each year (Figure 4), suggesting that the one-time addition of organic fertilizers and subsequent compost additions increased soil nutrient availability, which had a positive effect on crops. In many cases, soil nutrient availability continued to increase over the next 4 years. It is possible that the continued increase in nutrient availability was due at least in part to the practices of GBSA in which all crop residues are returned to the soil and soil erosion is minimized. Using GBSA, residues are returned through efficient and effective composting, and the soil remains covered and protected through continual, densely planted cropping. In addition, soil nutrients already present in the soil may have become more available through increased microbial activity resulting from the continual additions of compost, as well as the adjustment of soil pH where soil testing warranted (Schimel and Schaeffer, 2012; Weil and Brady, 2017; Banwart et al., 2019). Increased soil microbial activity also helped to immobilize nutrients that may otherwise have leached from the soil. It is also possible that soil biological activity and the GBSA practice of deep soil preparation improved soil structure and allowed greater access to nutrients deeper in the soil, which were then returned to the soil surface through composting.
Based on soil parameter results, the Muruka site had the least fertile soil (lowest pH, highest aluminum level, second lowest organic matter level, and lowest calcium, magnesium, and iron levels), which generally correlated with having the lowest edible and residue yields. This trend was not initially overcome by the one-time application of organic fertilizers and compost alone. However, edible and residue yields, and even soil parameter levels, suggest that the Muruka soil was comparably fertile to the other sites by the end of this 4-years study. This steady increase in fertility may be due to the combined effects of a one-time application of organic fertilizers and GBSA and warrants further study.
Maize grain yields increased over time across all sites but did show significant differences in yield between the rainy and dry seasons, which were expected since no supplemental irrigation was provided. Sorghum grain yields increased on average between Years 1, 2, and 3, but declined in Year 4 (Figure 1 and Table 4). Ratoon sorghum typically decreases in grain yield in Year 4 and is then replanted, correlating with our findings. Sweet potato tuber yields increased over this study period (Figure 1, Table 4), though there were small, temporary dips at each site. Increased tuber production due to increased soil fertility is expected since canopy photosynthesis has been significantly correlated to tuber yield (Bhagsari and Ashley, 1990). Amaranth leaf yields increased on average between Years 1 and 2, plateaued in Year 3, and declined in Year 4 (Figure 1 and Table 4). This decline in Year 4 was site specific, and most evident at Athi Gravity and to a lesser degree at G-BIACK (Figure 1). The decline in leaf yield at Athi Gravity and to a lesser extent at G-BIACK is attributed to decreased rainfall, which led to decreased production of amaranth leaves during growth.
Lablab and cowpea yields were initially difficult to interpret as they were affected by a change in harvesting practices during the course of this experiment. As with amaranth, the harvesting of lablab and cowpea leaf is generally done in a way that minimizes damage to the health of the plant. However, there are still rates of harvesting within this parameter that can increase leaf yield but reduce the overall productivity of the plant slightly or reduce leaf yield to increase overall productivity and the plant's residue yield.
Up to and including Year 3 Season 1, leaf harvesting of cowpeas and lablab was done with the goal of maximizing edible yield (either leaf or seed, respectively). However, in Year 3 Season 2 and for the rest of the experiment, leaf harvesting of cowpeas and lablab was done with the goal of maximizing crop residue yield and compost production. As a result of the change in harvesting practices, the interpretations of cowpea and lablab edible and residue yields were divided into two periods: harvesting for edible yields (HE) and harvesting for residue yields (HR). Within each harvesting period, cowpea edible yields did not significantly change, but comparing between periods, they were significantly higher during the HE period. Lablab yields significantly increased during the HE period, but they did not change significantly during the HR period. Comparing between harvesting periods, lablab edible yields were significantly higher in the HE period. The harvesting method used during the HE period was shown to be successful in boosting edible yields for cowpeas and lablab. The effect of this harvesting method on residue yields is described in more detail below.
Athi Gravity cowpea leaf yields were unusually high in Year 3 Season 1 (Figure 1 and Table 4). The farmer and authors attribute this increased yield to fortunate seasonal rainfall, which, due to timing and the unavailability of irrigation, had significant effect on cowpea yields in this semi-arid region.
Edible yields of maize, sorghum, and sweet potatoes are significantly higher than average yields reported by FAO over this 4-year period (Table 8; note: FAO data for amaranth, cowpeas and lablab were not available). These increased yields represent a 70% increase in calories. The trend of increasing percentages of yield differences between conventional agriculture and GBSA correlates with the trend of increasing yields with GBSA found during this study. The trend of increasing percentages of yield differences also suggests that the increases were due to management differences between conventional agriculture and GBSA rather than environmental differences.
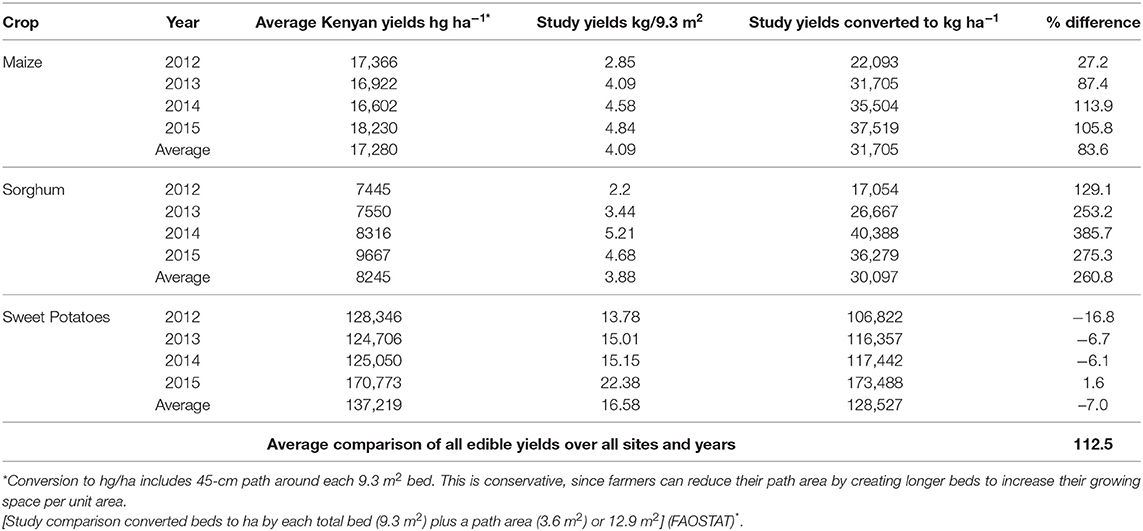
Table 8. 2012–2015 FAO reported yearly average Kenyan edible yields compared to study edible yield results.
Maize grain yields increased steadily and were 27.2, 87.4, 113.9, and 105.8% higher than average yields reported by FAO in Years 1 to 4, respectively (Table 8). Since there were no control beds managed with GBSA that did not receive the one-time application of organic fertilizers, we cannot attribute this performance to the fertilizers, the GBSA method, or both. However, Omondi et al. (2014) found substantial higher yields of maize grown with GBSA using the same spacing (131,800 hg ha−1). While the inclusion of pathway space and yields from whole beds may reduce their yield estimate somewhat, it collaborates the high yield potential of GBSA for maize grown in Kenya.
Residue Yields
The residue yields of maize increased slightly overall (Figure 5) but their responses varied by site with increases at G-BIACK and Muruka, slight increases at Athi Gravity, and variable responses at Thika River (Figure 2). While we would expect increases in corn residue yields that correspond to increases in maize grain yields in response to increased soil fertility and nitrogen availability (Sindelar et al., 2012), both Athi Gravity and Thika River experienced lodging of the corn crops each year due to heavy rains and winds. Sorghum residue yields increased over this study period. That the residue yields did not decline at Athi Gravity and Thika suggests that sorghum was less prone to lodging than maize. Sweet potato residue yields increased though each site experienced small dips (Figure 2 and Table 5) over the course of this study, which is expected from increased soil fertility.
Amaranth residue yields steadily increased over this study period, despite the decline in rainfall and leaf yield in Year 4. This is due to two factors: (1) green amaranth leaves are harvested when they are young and tender, and at a rate to minimize the damage to the health of the amaranth plant, which is based largely on the experience of the farmer and requires significant judgment; and (2) most of the total weight of a mature amaranth plant at harvest can be attributed to its heavy stalks and very little to the remaining dried leaves. It is likely that the lack of rainfall at Athi Gravity and G-BIACK reduced amaranth leaf harvesting rates, yet there were not overall declines but rather increases in residue yields.
Due to change in harvesting practices of cowpeas and lablab described earlier, we interpreted cowpea and lablab residue yields within and between these two harvesting periods (HE and HR). Cowpea yields did not change significantly during the HE period and increased marginally during the HR period. Lablab yields increased marginally during the HE period and showed no significant change during the HR period. However, both cowpea and lablab residue yields were greater in the HR period. The harvesting method used during the HR period was successful in boosting residue yields for cowpeas and lablab. Overall, the harvesting practices used in the HE and HR periods were successful at increasing edible or residual yields, respectively, for cowpeas and lablab, but increasing edible yields came at the cost of decreasing residual yields and vice versa. Altering harvesting methods of these crops can increase edible yields and human nutritional output. However, this approach likely provides only a short-term advantage as a decline in residue yields and on-farm compost production could lead to a decrease in soil organic matter and a loss of overall fertility if used continuously for multiple years.
The combined increases in crop residues led to each site being able to apply 0.073 m3 of compost to each bed during both seasons of the fourth year, rather than 0.049 m3 that had been applied per bed in previous seasons and years. The increase in compost material was reflected by the significant increase in soil organic matter across all sites over this 4-years study. This increase demonstrates the ability of GBSA, combined with a one-time application of organic fertilizers, to improve soil fertility and to help mitigate climate change. In addition, this increase suggests that soil organic matter levels could continue to increase past the 4-years period of this study.
Soil Parameter Responses
Over the course of this study, there was no significant decline in 13 of the 14 soil parameters overall. The one soil parameter that did decline significantly was manganese (Figure 3 and Table 7). The decline in manganese may have been due to seasonal rains that reduced available oxygen in the soil, causing manganese to become more mobile, in combination with weak adsorption by organic matter, the primary source of cation adsorption in these soils. Manganese is the most weakly held divalent cation by organic matter, with the exception of magnesium, but magnesium mobility is not increased under reducing conditions (Mengel et al., 2001). In addition, manganese levels in these soils were excessive in Year 1 (mean of 297.25 ppm) with ideal manganese levels from Mehlich III extractions ranging from 20 to 250 ppm and were still moderately high in Year 4 (mean of 125 ppm) (see Supplemental Materials: Ideal Soil Parameters).
Phosphorus, potassium, organic matter, sulfur, magnesium, boron, zinc, and electrical conductivity levels significantly increased over this study (Table 7). The single application of organic fertilizers included nutrients needed by these soils, as well as lime, which increased soil pH and could have made some nutrients more available. It is also possible the combination of needed nutrients, pH optimization, and the addition of compost allowed soil biology to further increase soil nutrient availability through mobilization and decrease nutrient losses through immobilization. Losses would still be expected from this system through leaching, volatilization, and erosion, as well as the lack of recycling nutrients contained in the human waste from those who consumed the crops. Further research is required to determine the point in time when those losses are greater than the amounts of nutrients added through biogeological processes.
While a steady increase in phosphorus can be explained through continual breakdown of rock phosphate added prior to Season 1 and increased microbial activity, the spike in phosphorus at G-BIACK in Year 3 (Figure 3) is anomalous. Upon receiving the test results that year, the laboratory retested the results on request and found a similarly high P level. Since no phosphorus-containing fertilizer had been added, it is possible that this was due to an uneven distribution of rock phosphate prior to Season 1 Year 1 and sample inhomogeneity in Year 3.
Sulfur (specifically sulfate) levels rose steadily over the course of this study and even faster in Year 4. Soil sulfate levels are highly variable even over the course of a growing season (Bloem et al., 2001), but are also directly correlated with soil microbial activity (Kersetz and Mirleau, 2004). The rise in sulfate in Year 4 could be attributed to the slow dissolving of gypsum, combined with the steady increase in soil organic matter, which led to the increase in total sulfate held in the soil and greater microbial activity and sulfate availability.
GBSA is a food production system that requires only human-powered tools, minimizes the need for external inputs, and has been shown to significantly improve yields (Jeavons, 2001; Rajbhsndari, 2011; Omondi et al., 2014), thus making it a system that is available and advantageous to almost any farmer in the world. However, if farmers are unable to test their soil and add recommended organic fertilizers, they must settle with crop yields that, while still higher due to GBSA, are increasingly constrained by soil nutrient limitations. This study suggests that edible and residue crop yields can increase over 4 years by testing your soil, applying appropriate organic fertilizers, and using GBSA. This approach allows costs to be distributed over at least 4 years and makes the process of improving soil fertility and crop yields more affordable and available to farmers globally.
After decades of reducing global hunger, since 2015, the number of those hungry has increased. In SSA in particular, the percentage of undernourished people went from 20.9 in 2015 to 22.8 in 2018 (FAO, 2019). Also, Africa has made the least progress in reducing stunting with 40% of global stunting in 2018 (FAO, 2019). To compound this existing problem, SSA population is expected to double (United Nations Department of Economic Social Affairs, 2010) and increase per-capita caloric demands.
GBSA combined with a one-time soil test and application of appropriate organic fertilizers offers a potential way for individuals, families, and communities to improve their food security.
Conclusion
A one-time application of appropriate organic amendments used within the GBSA system sustained or improved edible and crop residue yield over 4 years at four farms in Kenya. Along with positive plant responses, soil quality and nutrient cycling were sustained or improved. GBSA is a synergistic compilation of eight well-documented sustainable principles to offer farmers, especially small shareholders, a viable option to address the increased demand for food.
Limitations
Sample sizes were admittedly smaller than ideal in this initial study. However, the degree to which edible and residual yields and soil parameter levels were sustained or increased were beyond our expectations. This approach to increase food production and food sovereignty while reducing resource usage appears promising and should be further tested at additional sites under various climates, crops, and soils.
Data Availability Statement
All datasets generated for this study are included in the article/Supplementary Material.
Author Contributions
JB designed the research. SN conducted the research. LT provided the statistical analysis of the data. JB, SM, and LT interpreted the statistical analysis. JB and SM interpreted the results and had primary responsibility for writing the paper, with significant contributions to the Results section from LT. All authors reviewed and approved the final manuscript.
Funding
Funding to conduct the research was provided by REAP—Renewing Earth and Its People Foundation. The REAP Foundation is no longer active. Funding for open source publication was provided by Ecology Action.
Conflict of Interest
JB, SM, and SN have worked closely with those researching and teaching GBSA. They feel that this provides a sound understanding of the GBSA principles being tested but, in all cases, seek to have the research, data, and statistical analysis speak for itself without bias. JB is the owner of Grow Your Soil.
The remaining author declares that the research was conducted in the absence of any commercial or financial relationships that could be construed as a potential conflict of interest.
Acknowledgments
We are grateful to Dr. Laura Lengnick for her insights, comments, and contributions. We appreciate working with the G-BIACK farmers and hope their soils continue to thrive. We would like to thank Carol Moore and Meghan Beeby for proofreading for grammar, spelling, and clarity of ideas. The authors also would like to thank Dr. John Doran for his support.
Supplementary Material
The Supplementary Material for this article can be found online at: https://www.frontiersin.org/articles/10.3389/fsufs.2020.00067/full#supplementary-material
References
Akinnifesi, F. K., Ajay, O. C., Sileshi, G., Chirwa, P. W., and Chianu, J. (2010). Fertilizer trees for sustainable food security in the maize-based production systems of East and Southern Africa. A review. Agron. Sustain. Dev. 30, 615–629. doi: 10.1051/agro/2009058
Badgley, C., and Perfecto, I. (2007). Can organic agriculture feed the world? Renew. Agric Food Syst. 22, 80–86. doi: 10.1017/S1742170507001986
Baig, M. B., Shahid, S. A., and Straquadine, G. S. (2013). Marking rainfed agriculture sustainable through environmental friendly technologies in Pakistan: a review. Int. Soil Water Conserv. Res. 1, 36–52. doi: 10.1016/S2095-6339(15)30038-1
Baig, M. B., Zia, M. S., and Tahir, M. B. (1999). Soil environmental issues and their impact on agricultural productivity of low potential areas of Pakistan. Sci. Vision 4, 56–60.
Banwart, S. A., Nikolaidis, N. P., Zhu, Y.-G., Peacock, C. L., and Sparks, D. L. (2019). Soil functions: connecting earth's critical zone. Ann. Rev. Earth Plane Sci. 47, 333–359. doi: 10.1146/annurev-earth-063016-020544
Barrington, S., Choiniere, D., Trigui, M., and Knight, W. (2002). Effect of carbon source on compost nitrogen and carbon losses. Bioresour. Technol. 83, 189–194. doi: 10.1016/S0960-8524(01)00229-2
Bhagsari, A. S., and Ashley, D. A. (1990). Relationship of photosynthesis and harvest index to sweet potato yield. J. Am. Soc. Horticult. Sci. 115, 288–293. doi: 10.21273/JASHS.115.2.288
Bloem, E., Haneklaus, S., Sparovek, G., and Schnug, E. (2001). Spatial and temporal variability of sulphate concentration in soils. Commun. Soil Sci. Plant Anal. 32, 1391–1403. doi: 10.1081/CSS-100104201
Bodirsky, B. L., Rolinski, S., Biewald, A., Weindl, I., Popp, A., et al. (2015). Global food demand scenarios for the 21st century. PLoS ONE 10:e0139201. doi: 10.1371/journal.pone.0139201
Bomford, M. K. (2009). Tomatoes love basil but hate brussels sprouts? Competition and land-use efficiency of popularly recommended and discouraged crop mixtures in biointensive. J. Sustain. Agric. 33, 396–417. doi: 10.1080/10440040902835001
Brouder, S., and Gomez-Macpherson, H. (2014). The impact of conservation agriculture on smallholder agricultural yields: a scoping review of the evidence. Agric. Ecosyst. Environ. 187, 11–32. doi: 10.1016/j.agee.2013.08.010
Cairns, J. E., Hellin, J., Sonder, K., Araus, J. L., MacRobert, J. F., Thierfelder, C., et al. (2013). Adapting maize production to climate change in sub-Saharan Africa. Food Secur. 5:345–360. doi: 10.1007/s12571-013-0256-x
Declaration of Nyeleni (2007). Defining Food Sovereignty. Available ponline at: https://nyeleni.org/spip.php?article290 (accessed Augest 21, 2018).
Eiland, F., Klamer, M., Lind, A. M., Leth, M., and Baath, E. (2001). Influence of initial C/N ratio on chemical and microbial composition during long term composting of straw. Microbial. Ecol. 41, 272–289. doi: 10.1007/s002480000071
Epule, E. T., Bryant, C. R., Akkari, C., and Daouda, O. (2015). Can organic fertilizers set the pace for a greener arable agricultural revolution in Africa? Analysis, synthesis and way forward. Land Use Policy 47, 179–187. doi: 10.1016/j.landusepol.2015.01.0330264-8377
FAO IFAD, UNICEF, WFP WHO. (2019). The State of Food Security and Nutrition in the World 2019. Safeguarding Against Economic Slowdowns and Downturns. Rome, FAO. License: CC BY-NC-SA 3.0 IGO. Available online at: https://www.wfp.org/publications/2019-state-food-security-and-nutrition-world-sofi-safeguarding-against-economic (accessed July 29, 2019).
FAO. (2012). Sustainability Pathways: Smallholders and Family Farmers. Available online at: http://www.fao.org/fileadmin/templates/nr/sustainability_pathways/docs/Factsheet_SMALLHOLDERS.pdf. (accessed Augest 4, 2019).
Folberg, C., Yang, H., Gaiser, T., Liu, J., Wang, X., Williams, J., et al. (2014). Effects of ecological and conventional agriculture intensification practices on maize yields in sub-Saharan Africa under potential climate change. Environ. Res. Lett. 9:044004. doi: 10.1088/1748-9326/9/4/044004
Foley, J. A., Ramankutty, N., Brauman, K. A., Cassidy, E. S., Gerber, J. S., Johnston, M., et al. (2011). Solutions for a cultivated planet. Nature 478:337–342. doi: 10.1038/nature10452
Gliessman, S. R., (ed.). (1990). Agroecology: Researching the Ecological Basis for Sustainable Agriculture. Boca Raton, FL: Springer-Verlag. doi: 10.1007/978-1-4612-3252-0_1
Godfray, H. C. J., Beddington, J. R., Crute, I. R., Haddad, L., Lawrence, D., Muir, J. F., et al. (2010). Food security: the challenge of feeding 9 billion people. Science 327, 812–818. doi: 10.1126/science.1185383
Hatfield, J. L., and Morton, L.W. (2013). “Marginality principle,” in Principles of Sustainable Soil Management in Agroecosystems, eds R. La, and B. A. Stewart (Boca Raton, FL: CRC Press), 19–55.
Haug, R. T. (1993). The Practical Book of Compost Engineering. Lewis Publishers. Boca Raton, FL: CRC Press.
IPCC. (2007) “Climate change 2007: synthesis report,” in Contribution of Working Groups I II and III to the Fourth Assessment Report of the Intergovernmental Panel on Climate Change, eds R.K. Pachauri and A. Reisinger (Geneva: IPCC), 104.
Jeavons, J. (2001). Biointensive sustainable mini-farming. J. Sust. Agricult. 19, 49–106. doi: 10.1300/J064v19n02_10
Jeavons, J. (2017). How to Grow More Vegetables 9th ed. Ten Speed Press. USA 249 pp. ISBN: 978-0-399-57918-9.
Kersetz, M., and Mirleau, P. (2004). The role of soil microbes in plant sulphur nutrition. J. Exp. Botany 55, 1939–1945. doi: 10.1093/jxb/erh176
Kirchmann, H., and Bergstrom, L. (2001). Do organic farming practices reduce nitrate leaching? Commun. Soil Sci. Plant Analysis 32, 585–599. doi: 10.1081/CSS-100104101
Larsen, K. L., and McCartney, D. M. (2000). Effect of C:N ratio on microbial activity and N retention: bench-scale study using pulp and paper biosolids. Compost Sci. Utiliz. 8, 147–159. doi: 10.1080/1065657X.2000.10701760
Liu, J., Folberth, C., Yang, H., Rockström, J., Abbaspour, K., and Zehnder, A. J. B. (2013). A global and spatial explicit assessment of climate change impacts on crop production and consumptive water use. PLoS ONE 8:e57750. doi: 10.1371/journal.pone.0057750
Lowder, S., Skoet, J., and Raney, T. (2016). The number, size and distribution of farms, smallholder farms, and family farms worldwide. World Dev. 87, 16–29. doi: 10.1016/j.worlddev.2015.10.041
Magdof, F., and Van Es, H. (2009). Building Soil for Better Crops: Sustainable Soil Management 3rd ed. Waldorf, MD: Sustainable Agriculture Publications.
Mahon, N., Crute, I., Di Bonito, M., Simmons, E. A., and Islam, M. M. (2018). Towards a broad-based and holistic framework of Sustainable Intensification indicators. Land Use Policy 77, 576–597. doi: 10.1016/j.landusepol.2018.06.009
Man, Y., Zhang, J., Xu, Y., Xiao, H., An, W., Xi, H., et al. (2015). Fungal community dynamics and driving factors during agricultural waste decomposting. Environ. Sci. Pollut. Res. 22, 19879–19886. doi: 10.1007/s11356-015-5172-5
Mengel, K., Kirkby, E. A., Kosegarten, H., and Appel, T. (2001). “Soil copper,” in Principles of Plant Nutrition, eds K. Mengel, E. A. Kirkby, H. Kosegarten, T. Appel (Dordrecht: Springer), 541, 542, 599.
Mohajan, H. K. (2014). Food and Nutrition Scenario of Kenya. Am. J. Food Nutr. 2, 28–38. doi: 10.12691/ajfn-2-2-3
Montpellier Panel Report. (2013). Sustainable intensification: A new paradigm for Africa agriculture. Agriculture for Impact, 15 Princes Gardens, South Kensington Campus. Imperial College, London, UK, SW7 1NA www.ag4impact.org Available online at: https://ag4impact.org/wp-content/uploads/2013/04/MP_0176_Report_Redesign_2016.pdf (accessed July 29, 2019).
Moore, S. R. (2010). Energy efficiency in small-scale biointensive organic onion production in Pennsylvania, USA. Renew. Agricult. Food Syst. 25, 181–188. doi: 10.1017/S1742170510000098
Muller, A., Schader, C., Scialabba, N., Bruggemann, J., Isensee, A., Erb, K.-H., et al. (2017). Strategies for feeding the world more sustainably with organic agriculture, Nat. Commun. 8:1290. doi: 10.1038/s41467-017-01410
Murphy, D. E. (2017). Factors that lead to success in implementing Biointensive gardens and in serving as Biointensive gardening extension agents in Nicaragua: Using a qualitative approach (master's thesis), Department of Global Health, Milken Institute School of Public Health, The George Washington University, Washington, D.C. United States.
Omondi, E. C., Norton, J. B., and Ashienje, D. S. (2014). Performance of a local open pollinated maize variety and a common hybrid variety under intensive small-scale farming practices. Afric. J. Agricult. Res. 9, 950–955. doi: 10.5897/AJAR2013.7359
Petersen, B., and Snapp, S. (2015). What is sustainable intensification? Views from the experts. Land Use Policy 46, 1–10. doi: 10.1016/j.landusepol.2015.02.002
Pretty, I., Toulmin, C., and Williams, S. (2011). Sustainable intensification in Africa agriculture. Int. J. Agricult. Sust. 9, 5–24. doi: 10.3763/ijas.2010.0583
Rajbhsndari, B. P. (2011). Bio-intensive farming system: Validation of its approaches in increasing food production, improving food security and livelihoods. Nepalese J. Agricult. Sci. 9, 112−123.
Reganold, J. P., and Wachter, J. M. (2016). Organic agriculture in the twenty-first century. Nat. plants 2:15221. doi: 10.1038/NPlANTS.2015.221
Rockström, J. (2003). Water for food and nature in drought-prone tropics: vapour shift in rain-fed agriculture. Philosoph. Trans. R. Soc. B 358, 1997–2009. doi: 10.1098/rstb.2003.1400
Rockström, J., Steffen, W., Noone, K., Persson, A., Chapin, I. I. I. F. S., et al. (2009). Planetary boundaries: exploring the safe operating space for humanity. Ecol. Soc. 14:32. doi: 10.5751/ES-03180-140232
Rockström, J., Williams, J., Daily, G., Noble, A., Matthews, N., Gordon, L., et al. (2015). Sustainable Intensification of Agriculture for Human Prosperity and Global Sustainability. Ambio. Avaialble online at: https://link.springer.com/article/10.1007/s13280-016-0793-6 (retrieved 2/27/2019).
Roxburgh, C., Dorr, N., Leke, A., Tazi-Riffi, A., van Wamelen, A., Lund, S., et al. (2011). Lions on the Move: the Progress and Potential of African Economies. McKinsey Global Institute. Available online at: https://www.mckinsey.com/~/media/McKinsey/Featured%20Insights/Middle%20East%20and%20Africa/Lions%20on%20the%20move/MGI_Lions_on_the_move_african_economies_full_report.ashx (accessed December 2, 2020)
Sans, P., and Combris, P. (2015). World meat consumption patterns: An overview of the last fifty years (1961-2011). Meat Sci. 109, 106–111. doi: 10.1016/j.meatsci.2015.05.012
Schimel, J., and Schaeffer, S. M. (2012). Microbial control over carbon cycling in soil. Front. Microbiol. 3:348. doi: 10.3389/fmicb.2012.00348
Stocking, W. A. (2003). Tropical Soils and Food Security: The Next 50 Years. Science. 302:1356–1359. doi: 10.1126/science.1088579
Seufert, V., Ramankutty, N., and Foley, J. (2012). Comparing the yields of organic and conventional agriculture. Nature. 485, 229–232. doi: 10.1038/nature11069
Silici, L. (2014). Agroecology: What it is and What It Has to Offer. London: Issue paper International Institute for Environment and Development (IIED).
Sindelar, A. J., Lamb, J. A., Sheaffer, C. C., Jung, H. G., and Rosen, C. J. (2012). Response of corn grain, cellulosic biomass, and ethanol yields to nitrogen fertilization. Agronom. J. 104, 363–370. doi: 10.2134/agronj2011.0279
Tilman, D., Balzer, C., Hill, J, and Befort, B. L. (2011). Global food demand and the sustainable intensification of agriculture. Proc. Natl. Acad. Sci. U.S.A. 108, 20260–20264. doi: 10.1073/pnas.1116437108
Tiquia, S. M., Richard, T. L., and Honeyman, M. S. (2002). Carbon, nitrogen and mass loss during composting. Nutr. Cycling Agroecosyst. 62, 15–24. doi: 10.1023/A:1015137922816
United Nations Department of Economic Social Affairs. (2010). Population Division, Population Estimates and Projections Section. Availabe online at: www.esa.un.org/wpp/panel_population (retrieved 8/15/2018).
Prasad, P. V. V., Hijimans, R. J., Pierzynski, G. M., and Middendorf, J. B. (2016). Climate Smart Agriculture and Sustainable Intensification: Assessment and Priority Setting for Rwanda. Available online at: https://2012-2017.usaid.gov/sites/default/files/documents/1860/Rwanda-CSA-SI-Assessment%20Report.pdf (retrieved May 26, 2020).
Wallace, J. S. (2000). Increasing water use efficiency to meet future food production. Agricult. Ecosyst. Environ. 82, 105–119. doi: 10.1016/S0167-8809(00)00220-6
Weil, R. R., and Brady, N. C. (2017). The Nature and Properties of Soil, 15th ed. London: Pearson Education Limited.
World Bank. (2018). Sustainable Agriculture Intensification and Food Security Project. http://documents.worldbank.org/curated/en/231671528451531272/pdf/SAIP-Environmental-and-Social-Management-Framework.pdf (retrieved May 26, 2020).
WSU (2019). Compost Fundamentals-Reclamation of Nitrogen and Other Nutrients. Availabe online at: http://whatcom.wsu.edu/ag/compost/fundamentals/consideration_reclamation.htm (retrieved 4/9/2019).
Keywords: sustainable agriculture, biointensive agriculture, organic, soil fertility, yield gap, sustainability, renewable agriculture, Africa
Citation: Beeby J, Moore S, Taylor L and Nderitu S (2020) Effects of a One-Time Organic Fertilizer Application on Long-Term Crop and Residue Yields, and Soil Quality Measurements Using Biointensive Agriculture. Front. Sustain. Food Syst. 4:67. doi: 10.3389/fsufs.2020.00067
Received: 09 October 2019; Accepted: 20 March 2020;
Published: 19 June 2020.
Edited by:
Stephen James Ramsden, University of Nottingham, United KingdomReviewed by:
Ilias Travlos, Agricultural University of Athens, GreeceManoj Shrivastava, Indian Agricultural Research Institute (ICAR), India
Copyright © 2020 Beeby, Moore, Taylor and Nderitu. This is an open-access article distributed under the terms of the Creative Commons Attribution License (CC BY). The use, distribution or reproduction in other forums is permitted, provided the original author(s) and the copyright owner(s) are credited and that the original publication in this journal is cited, in accordance with accepted academic practice. No use, distribution or reproduction is permitted which does not comply with these terms.
*Correspondence: John Beeby, jsb76@cornell.edu; Steve Moore, harmonyessentials@gmail.com