Targeting TRP channels: recent advances in structure, ligand binding, and molecular mechanisms
- 1Department of Chemistry, University of Massachusetts, Amherst, MA, United States
- 2Modeling and Informatics, Merck & Co., Inc., West Point, PA, United States
Transient receptor potential (TRP) channels are a large and diverse family of transmembrane ion channels that are widely expressed, have important physiological roles, and are associated with many human diseases. These proteins are actively pursued as promising drug targets, benefitting greatly from advances in structural and mechanistic studies of TRP channels. At the same time, the complex, polymodal activation and regulation of TRP channels have presented formidable challenges. In this short review, we summarize recent progresses toward understanding the structural basis of TRP channel function, as well as potential ligand binding sites that could be targeted for therapeutics. A particular focus is on the current understanding of the molecular mechanisms of TRP channel activation and regulation, where many fundamental questions remain unanswered. We believe that a deeper understanding of the functional mechanisms of TRP channels will be critical and likely transformative toward developing successful therapeutic strategies targeting these exciting proteins. This endeavor will require concerted efforts from computation, structural biology, medicinal chemistry, electrophysiology, pharmacology, drug safety and clinical studies.
Introduction
Ion channels are integrated membrane proteins that facilitate and regulate the passage of ions through membranes (Hille, 2001). Their activities are controlled by various cellular stimuli including chemical ligands, voltage, temperature, mechanical force and others (Keynes, 1975; Hebert, 1998; Minor, 2010). Dysfunction or mis-regulation of ion channels can lead to a plethora of diseases (Hübner and Jentsch, 2002; Zaydman et al., 2012), and they are considered one of the most important classes of drug targets (Kaczorowski et al., 2008; Clare, 2010; Bagal et al., 2013; Santos et al., 2017; Hutchings et al., 2019). Transient receptor potential (TRP) channels, in particular, are a large and diverse family of ion channels second in size only to potassium channels (Gees et al., 2012; Cao, 2020). They play critical roles in sensory perception and possess polymodal activation by various physical and chemical stimuli (Nilius and Owsianik, 2011). There are 27 members in the human TRP ion channel superfamily. They can be further divided into six subfamilies based on sequence homology, namely, TRPC1-7 (canonical), TRPV1-6 (vanilloid), TRPM1-8 (melastatin), TRPA1 (ankyrin), TRPML1-3 (mucolipin), and TRPP2-3 (polycystins) (Montell et al., 2002). Note that the sequence-based classification does not necessarily cluster TRP channels with the same or similar functionalities–members within one subfamily can have distinct functions. TRP channels are widely expressed in most cells, tissues and organs with varying expression patterns among members (Nilius and Owsianik, 2011). For example, TRPC, TRPA, TRPM are primarily localized in plasma membrane, whereas TRPML and TRPP channels locate in the cytosolic compartments due to their C-terminal endoplasmic reticulum retention-signaling domain.
As multifunctional signaling proteins, TRP channels can sense a wide range of external and internal stimuli and trigger downstream physiological responses (Clapham, 2003; Voets et al., 2005). While the functions of some TRP channels have been well studied, many others remain insufficiently characterized, especially at the molecular level. For example, TRPV1 has a significant role in thermoregulation (Romanovsky et al., 2009; Szolcsányi, 2015), TRPM8 plays a central role in cold sensing (McKemy et al., 2002; Peier et al., 2002; Brauchi et al., 2004; Bautista et al., 2007), TRPA1 could serve as a sensor for pain, noxious cold temperature, environmental irritants, cellular stress and tissue damage (Caspani and Heppenstall, 2009; Viana, 2016; Meents et al., 2019; Souza Monteiro de Araujo et al., 2020) and TRPV5 and TRPV6, two epithelial calcium channels, are responsible for Ca2+ reabsorption and thus play a key role in calcium homeostasis (van Abel et al., 2005; van Goor et al., 2017; Khattar et al., 2022; Walker and Vuister, 2023). Overall, due to their important sensory perception roles, studies of the physiological function, activation and regulation of TRP channels have been and will continue to be a hot spot in biological and biomedical research.
With their widespread expression in the human body and extensive involvement in various key psychological and pathological processes (Nilius and Owsianik, 2011), TRP channels are attractive therapeutic targets for treatment of both acquired and hereditary channelopathies (Moran et al., 2011; Fallah et al., 2022; Koivisto et al., 2022). Many traditional natural products from plants and animals have been discovered to target TRP channels. For example, capsaicin from Capsicum and resiniferatoxin from resin spurge are activators of TRPV1, cannabinoids from Cannabis activates TRPV2, menthol from mint can target TRPM8 and TRPV3, and various pungent ingredients from wasabi, mustard, radish activate TRPA1. These compounds have been well-curated in several seminal review papers (Calixto et al., 2005; Vetter and Lewis, 2011; Zhang et al., 2019). These examples also highlight great potentials in exploiting natural products for targeting TRP channels. Many drug candidates, either from natural or synthetic origins, are currently in clinical trials, targeting various TRPVs as well as TRPA1 and TRPM8 channels (Moran and Szallasi, 2018; Iftinca et al., 2021). Furthermore, high resolution structures are now available for all subfamilies at multiple functional states, providing a solid basis for rational approaches toward targeting these proteins (Cao, 2020; Huffer et al., 2020; Diver et al., 2022). Yet, significant gaps remain in the current understanding of the activation and regulation of TRP channels at the molecular level. In this review, we summarize the therapeutic potential of TRP channels as well as recent advances in structural studies of TRP channels, with an emphasis on known ligand binding sites and mechanistic features of TRP channel gating and regulation. We also discuss the perspective on how understanding the molecular mechanisms can help to advance therapeutics and drug development targeting TRP channels.
Pathological and therapeutic roles of TRP channels
Hereditary mutations in TRP channels can cause a variety of channelopathies, which is not surprising given their important regulatory roles in membrane excitability of sensory neurons and cellular ion homeostasis (Yue and Xu, 2021). For example, TRPV4, which is involved in the most well-documented mutation-induced inheritable channelopathies, is directly linked to peripheral neuropathies, skeletal dysplasia and arthropathy with varied phenotypes and syndromes (Dai et al., 2010; Nilius and Owsianik, 2010; Nilius and Voets, 2013). Currently reported TRP hereditary channelopathies are summarized in Table 1, highlighting the importance of TRP channels as drug targets. Direct modulation of the activities of TRP channels through drugs has also been pursued as an effective strategy to intervene the progressions of pain, respiratory disease, cancer and diabetes (Santoni and Farfariello, 2011; Brederson et al., 2013; Colsoul et al., 2013; Shapovalov et al., 2016; Belvisi and Birrell, 2017). The current status of drug discovery and clinical trials of TRP channels has been well-covered in recent reviews (Yue and Xu, 2021; Koivisto et al., 2022). Herein, we will focus on the most well-known TRP-related acquired diseases – pain and respiratory diseases.
Some TRP channels, such as TRPV1-4, TRPA1 and TRPM8, are richly expressed in sensory neurons (Mickle et al., 2015), and are prime analgesic targets to eliminate pain sensation (Dai, 2016; Moran and Szallasi, 2018). It has been known that both agonists and antagonists of TRPV1 could silence TRPV1-mediated nociception due to its prolonged desensitization after applying agonists (Noto et al., 2009; Chung and Campbell, 2016; Bonezzi et al., 2020). Downregulating or antagonizing TRPA1 has been shown to reduce cold hyperalgesia in nerve injury models (Obata et al., 2005; Katsura et al., 2006; Caspani et al., 2009; Staaf et al., 2009), mechanical allodynia (Eid et al., 2008; Kerstein et al., 2009; Kwan et al., 2009; Wei et al., 2011; Zappia et al., 2017), and painful diabetic neuropathy (Koivisto et al., 2012) and chemotherapeutic-induced peripheral neuropathy (Staff et al., 2017). Antagonists of TRPM8 have been documented in the treatment of chronic pain and migraine (Weyer and Lehto, 2017). Some natural agonists of TRPM8, such as menthol, have been used for centuries due to their analgesic effects (Patel et al., 2007). These and other TRP channels involved in pain sensation and relief have been extensively reviewed in many seminal reviews (Willis, 2009; Brederson et al., 2013; Fernández-Peña and Viana, 2013; Dai, 2016; González-Ramírez et al., 2017; Moran and Szallasi, 2018; Souza Monteiro de Araujo et al., 2020), which speaks volume to the importance of these ion channel’s role in nociception and the great promise of TRP-targeting drugs in the treatment of pain of various natures.
Interestingly, many nociceptive TRP channels are also expressed widely in sensory neurons that innervate the airway as well as in non-neuronal cells in the lung including structural and immune cells (Belvisi and Birrell, 2017). These channels thus play important roles in the pathophysiology of respiratory diseases [such as asthma and chronic obstructive pulmonary disease (COPD) and chronic refractory cough] (Grace et al., 2014; Koivisto et al., 2022). Antagonizing TRPV1, TRPA1, and TRPV4 was shown to have anti-coughing effects in animal models (Andrè et al., 2009; Khalid et al., 2014; Mukhopadhyay et al., 2014; Bonvini et al., 2016; Mason et al., 2020, p. 4). Airway hypersensitivity, as a respiratory symptom of asthma, can be suppressed by TRPV1 and TRPA1 inhibitors (Raemdonck et al., 2012; Baker et al., 2016). TRPV4 has been frequently linked to pulmonary diseases including acute lung injury, pulmonary edema formation, and pulmonary hypertension, due to its role of sensing osmolarity to regulate the pulmonary capillary permeability (Goldenberg et al., 2015b; Rosenbaum et al., 2020). Inhibition of TRPV4 has also been suggested to be a promising therapeutic route for treating acute lung injury/acute respiratory distress syndrome (ARDS) (Goldenberg et al., 2015a), and more recently for treating COVID-19 patients with lung edema (Kuebler et al., 2020).
The therapeutic potential of TRP channels for other acquired diseases has also been reported. Pharmacological inhibition of TRPM2 shows beneficial effects toward ischemia/reperfusion (I/R) injury in brain, heart and kidney (Zhan et al., 2016). Inhibition of TRPCs, such as TRPC4 and TRPC5, have anxiolytic and antidepressant effects in mice, which could potentially be used for treatment of anxiety disorders (Just et al., 2018). Many TRPs are also intimately connected to itching (Tóth et al., 2015), cardiovascular diseases (Watanabe et al., 2008; Yue et al., 2015), kidney diseases (Hsu et al., 2007; Chubanov et al., 2017), diabetes (Colsoul et al., 2013; Zsombok and Derbenev, 2016), and cancers (Lehen’kyi and Prevarskaya, 2011; Santoni and Farfariello, 2011; Shapovalov et al., 2016; Yang and Kim, 2020).
Developing drugs for TRP-related acquired channelopathies requires deeper understanding of the signaling pathways or the interaction/regulation networks of the TRP channels. For example, TRPV1 antagonists and agonists seemingly have similar therapeutic effects for pain relief (Moran and Szallasi, 2018; Iftinca et al., 2021). The implication follows that developing both inhibitors and activators for TRP channels allows for dealing with complicated syndromes with different therapeutic strategies and/or to minimize side-effects. Cautions, however, should be taken when interpreting the therapeutic effects of agonists or antagonists on these channels. For example, menthol, a known TRPM8 activator, has been used as an antitussive drug, but its mechanism of action may not derive from TRPM8 activation as menthol can also interact with TRPA1 (Karashima et al., 2007). Complication due to promiscuity of various antagonists and agonists toward TRP channels has to be considered, which will likely benefit from better understanding of the molecular mechanism of interaction and regulation of the ligand.
Recent progresses in TRP channel structure and ligand binding
The first high-resolution TRP channel structure was not determined until 2013, when the structure of TRPV1 was resolved at near-atomic resolution thanks to breakthroughs in cryo-electron microscopy (cryo-EM) (Cao et al., 2013; Liao et al., 2013). This landmark work ushered in a new era in structural biology, where cryo-EM can now be readily applied to obtain high-resolution structures of membrane proteins and other complex bio-macromolecules (Cao, 2020; Diver et al., 2022). Ten years on, there are over 350 structures of TRP channels deposited in the Protein Data Bank (PDB) as of October 2023 (Supplementary Appendix Table 1). At least one structure exists for all the TRPVs, most TRPMs, TRPC3-6, TRPA1, all TRPMLs and TRPP1-3 members. For many TRP channels, structures are available in multiple functional and/or ligand-bound states (either agonists or antagonists), especially those within the TRPV, TRPM and TRPA subfamilies (Supplementary Appendix Table 1). These structures have provided crucial insights into the molecular basis of ion conductance, activation and regulation of TRP channels.
The TRP channel superfamily can be divided into two subgroups based on their structural features as well as cellular distributions (Montell, 2005). The first subgroup consists of TRPCs, TRPVs, TRPMs, and TRPA. They mainly distribute in the plasma membrane and share similar structural features. Structurally, this subgroup of TRP channels exists as tetramers, featuring six transmembrane (TM) helices in each protomer. Following the S6 helix, a TRP helix or so-called TRP box runs parallel to the membrane surface and is believed to play an important role in gating of TRP channels. The second subgroup includes TRPML and TRPP, which are located in the endosome membrane and do not have the TRP box. While TRP channels in the first subgroup contain large cytosolic domains from each protomer assembling as a skirt-like or multiple-layered structure enveloping a large cytosolic cavity (e.g., Figures 1–3), TRP channels from the second subgroup have large “extracellular” segments inserted between S1 and S2 and form a “cap”-like domain (Figure 4). In this review, we will focus on the ligand binding pockets shared among TRP channels and analyze the degree of binding site similarity and conservation among each subfamily, to provide some guidance for future TRP drug discovery and pharmaceutical research.
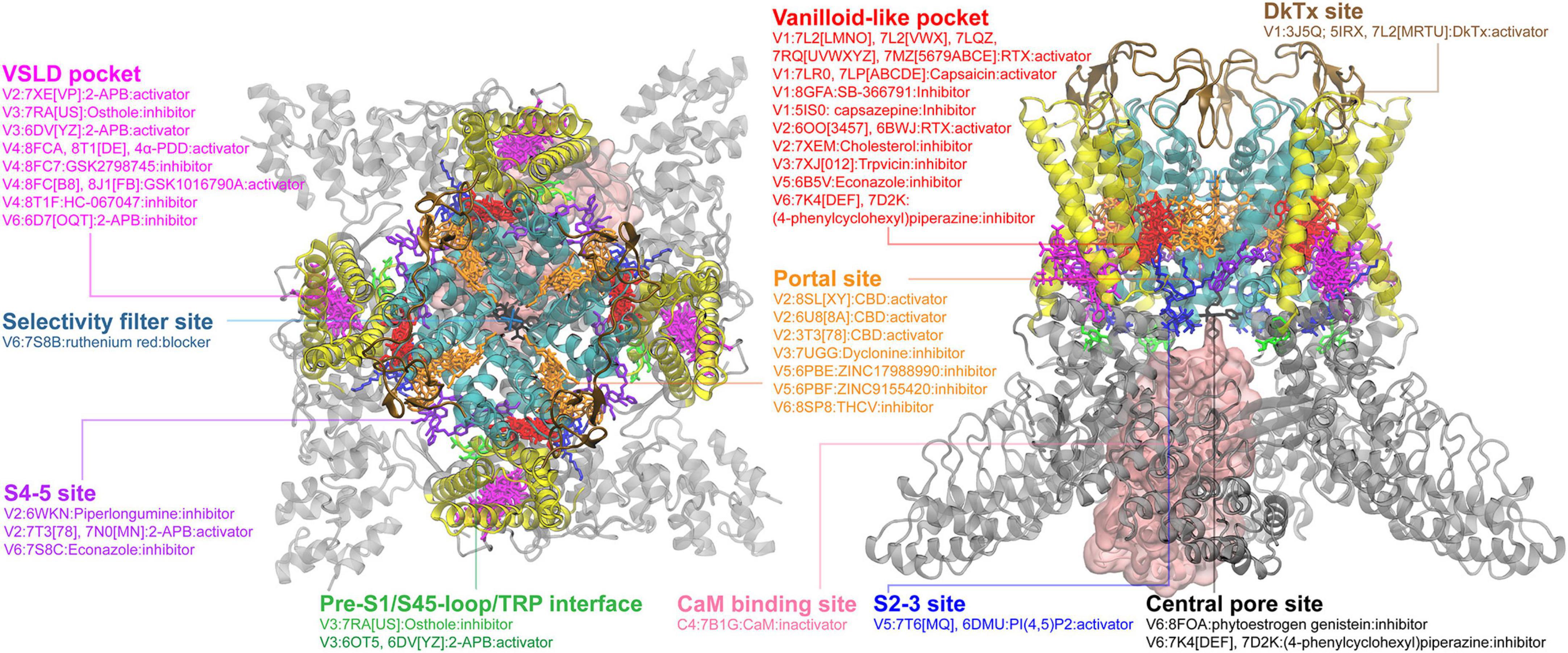
Figure 1. Ligand binding sites in TRPV channels. The TRPV1 structure in complex with DkTx (PDB: 3J5Q) is used for visualizing the binding sites, with the pore region, the VSLD region and cytosolic domain colored in cyan, yellow and gray, respectively. All other structures were first structurally aligned using US-align (Zhang et al., 2022) to allow the clustering of all ligands observed. The major binding pockets or interfaces in the TRPV channels are highlighted using clusters of ligands shown in different colors. The side view in the right panel is rendered by rotating along the x-axis of the top view (the left panel) by 90° and then along the y-axis by 45° for a better view of the binding sites. Some ligands can have multiple binding poses within the same pocket; only one configuration is shown for clarity. Binding sites for ions or ion blockers are not shown.
TRPVs
As the most intensively investigated TRP subfamily, structures are available in different functional or ligand-bound states for all TRPV members. A thorough review of the ligand binding pockets in TRPV channels has been recently published, which summarizes 16 distinct binding sites in TRPV channels (Yelshanskaya and Sobolevsky, 2022). Though several new ligand-bound structures have been deposited in the PDB since, no additional binding site has been discovered. Here we will briefly summarize the most important sites (Figure 1), and discuss some of the very recent studies not included in the 2022 review. The so-called vanilloid site is the most frequently observed one. It is an interface cavity formed between S3, S4, S4-5 linker and the neighboring S5 and S6 helices. Ligands that bind to the vanilloid site include both activators, such as capsaicin (Cao et al., 2013; Kwon et al., 2021; Nadezhdin et al., 2021a), resiniferatoxin (Nadezhdin et al., 2021a; Zhang et al., 2021; Kwon et al., 2022), and inhibitors, such as capsazepine and SB-366791 in TRPV1 (Gao L. et al., 2016; Neuberger et al., 2023), econazole in TRPV5 and (4-phenylcyclohexyl)piperazine derivatives (PCHPDs) in TRPV6 (Hughes et al., 2018; Neuberger et al., 2021). It is worth mentioning that, when a ligand molecule is not present, the vanilloid site is generally occupied by lipid molecules, such as phosphatidylinositol lipid (PI) observed for TRPV1 (Gao L. et al., 2016; Zhang et al., 2021) and phosphatidylcholine lipid (PC) for TRPV3 (Nadezhdin et al., 2021b). The effects of lipid binding in the vanilloid site can be either inhibitory or excitatory and appear to have different physiological and functional implications among TRPV members (Cheng et al., 2022). Su et al. (2023) recently showed that binding of endogenous cholesterol to the vanilloid pocket inhibited the TRPV2 channel activity.
The second important binding site in TRPV channels is the S1-S4 bundle site or the VSLD pocket, formed by the S1-S4 helix bundle and the TRP helix. Several chemicals showing either activation (2-APB in TRPV3) or inhibition (2-APB-Br, Osthole in TRPV3 and ZINC17988990 in TRPV5) have been discovered to bind in this VSLD cavity (Figure 1; Supplementary Appendix Table 1). Recently, the agonists (4-alpha-PDD and GSK1016790A) and antagonists (HC-067047 and GSK2798745) bound structures of human TRPV4 were resolved, showing that both agonists and antagonists can bind to the VSLD cavity (Kwon et al., 2023; Nadezhdin et al., 2023b). The cryo-EM structures of TRPV channels also show lipids can occupy the VSLD cavity in absence of other ligands, the native functional implications of which need to be further investigated (Supplementary Appendix Table 1). The third major binding site is the portal site, which is the pocket formed by the S5 and the pore helix (PH) of one subunit plus the neighboring S6 helix. Cannabidiol or cannabidiol derivatives have been shown to bind to this portal site in TRPV2 (Gochman et al., 2023). Other compounds such as ZINC17988990 or ZINC9155420 inhibit TRPV5 by binding to this site (Hughes et al., 2019). Recently, the anesthetic dyclonine was also found to bind to the portal site in TRPV3, providing the structural basis of how this compound can relieve pain and itch in the traditionally topical applications (Neuberger et al., 2022). Some other binding sites (Figure 1) have also been found within TRPV members, such as central pore sites (sites along the central permeation pathway), the S4-5 site (the interface between VSLD and S5-6 pore helices, also referred as “deep” or “shallow” S4-5 in the 2022 review), the S2-3 site [the PI(4,5)P2 binding site in TRPV5], and the cytosolic calmodulin (CaM) binding site (Yelshanskaya and Sobolevsky, 2022). Those additional sites indicate the TRPV channels have the potential to be targeted by drugs in other less common but important interfaces or pockets. It is noteworthy that ligand binding to the same site can have different or sometimes completely opposite effects on the channel function, suggesting a high level of adaptability of the binding pockets and the likely presence of multiple coupling pathways and/or regulatory mechanisms with the TRP channel proteins.
TRPMs
The TRPM subfamily members have also attracted intensive attention in recent years due to their important roles in sensing temperature, taste, oxidative state and osmolarity, cellular proliferation, cell death, neurological diseases and cancer progression (Jimenez et al., 2020). Cryo-EM structures have been determined for all TRPM channels in both the apo and bound states with different ligands, except for TRPM1 and TRPM6 (Huang et al., 2020). TRPM and TRPV channels share the similar architecture in the TM region (Figures 1, 2). Two of the major ligand binding pockets identified for TRPV channels, the vanilloid-like pocket and the VSLD pocket, are also present in TRPM channels (Figure 2). For the vanilloid-like pocket, inhibitor-bound structures such as VER155008 and NS8593 in TRPM7, N′-(3,4-dimethoxybenzylidene)-2-(naphthalen-1-yl)acetohydrazide (NDNA) in TRPM5, and activator-bound structure, Naltriben in TRPM7, have been reported (Ruan et al., 2021; Nadezhdin et al., 2023a), showing again the adaptability of this pocket. The VSLD cavity, so far only observed in the TRPM8 cryo-EM structures, can also accommodate ligands with either inhibitory (AMTB) or excitatory (TC-1, WS-12, icilin) effects. Interestingly, the portal site in TRPMs has not been found to bind any inhibitors or activators but can be occupied by lipids (Diver et al., 2019). Together with the large accessible groove on the inter-protomer surface, the portal site clearly has the potential to bind ligands and modulate the gating/activation process of the TRPM channels.
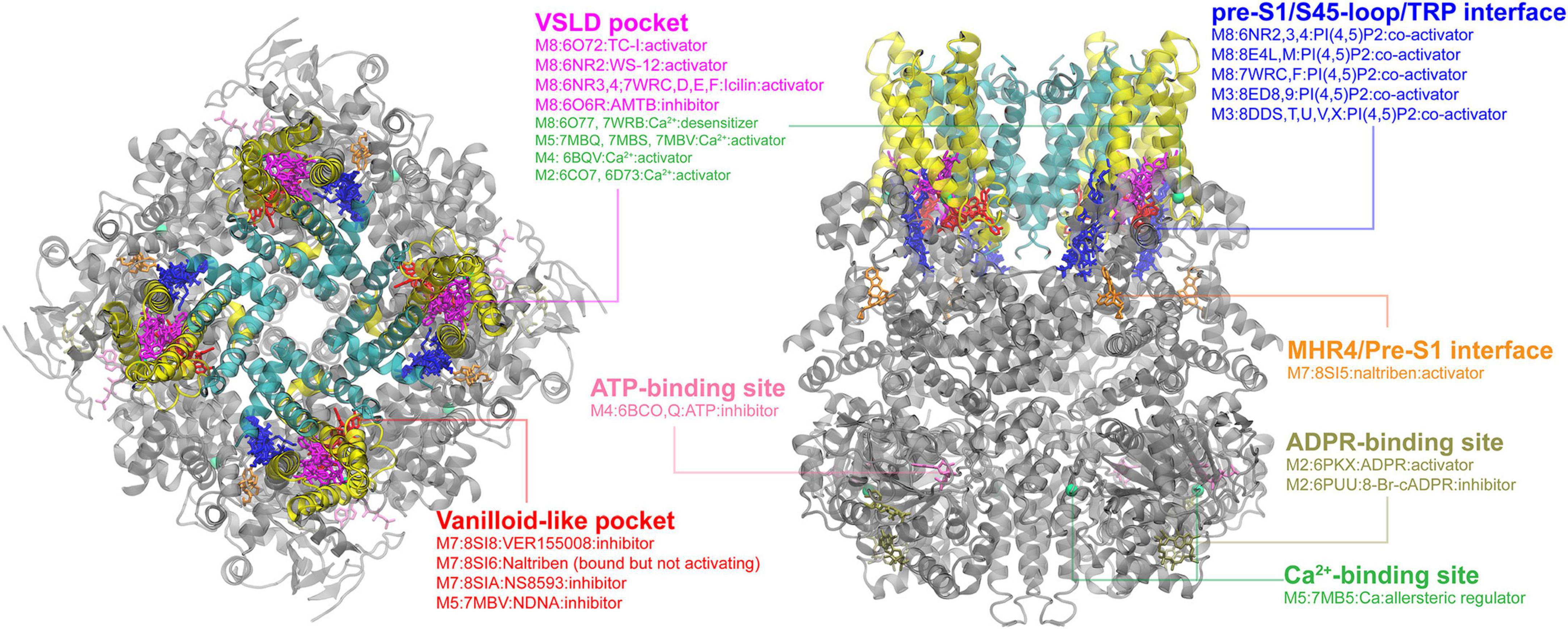
Figure 2. Ligand binding sites in TRPM channels. The TRPM8 structure with TC-I 2014-bound (PDB: 6O72) is used for visualization, with the pore region, the VSLD region and cytosolic domain colored in cyan, yellow, and gray, respectively. The major binding pockets or interfaces are highlighted using different colors. The top and side views are rendered in the same way as in the TRPV channel (see Figure 1).
Another interesting feature of TRPMs is that the cytosolic domain of TRPMs usually contains of 4 melastatin homology regions (MHR1-4) instead of ankyrin repeats in TRPV channels. This variance provides several unique binding sites in TRPMs. The first one is the pre-S1/S4-5 loop/TRP helix interface, which provides a positively charged electrostatic environment to bind the PI(4,5)P2 molecule. So far, fourteen PI(4,5)P2-bound structures (seven for each TRPM3 and 8) reveal that the PI(4,5)P2 head group all binds into a similar position on this interface. However, the PI(4,5)P2 binding site might not be conserved in other TRPM channels, because the interacting residues are not conserved among TRPMs (Yin et al., 2019a). It would be interesting to dissect the PI(4,5)P2 binding in TRPMs because the important regulatory effect of PI(4,5)P2 on TRPMs has been long recognized (Runnels et al., 2002; Liu and Liman, 2003; Zhang et al., 2005; Nilius et al., 2006; Xie et al., 2011). Other less common cytosolic binding sites have also been reported individually in several TRPMs. The naltriben-bound TRPM7 structure reveals that the ligand binds to MHR4/pre-S1 interface and activates the channel by pulling MHR4 to the neighboring MHR repeat and triggering a rigid body rotation of the whole N-terminal domain (Nadezhdin et al., 2023a). In TRPM2, there is a unique ADPR (ADP ribose)-binding site, which is located at the cleft of the MHR1/2 and is far away from the central pore domain (Yin et al., 2019b). It has been shown that binding of ADPR to this site can activate the channel, while binding of its derivative 8-Br-cADPR can block the allosteric coupling from MHR3-4 to the central pore and lock the conformation in apo state (Huang et al., 2019; Yin et al., 2019b). In TRPM4, a nucleotide-binding site in the N-terminal region was found to bind to ATP, allosterically inhibiting the channel activity (Guo et al., 2017). These cytosolic sites are in general far away from the pore domain and thus pose an intriguing question on what long range coupling mechanism(s) would allow the ligand binding to control the pore domain.
TRPCs
All members of the “canonical” TRP channels, TRPCs, were discovered in the late 1990s (Wes et al., 1995; Zhu et al., 1995, 1996; Okada et al., 1999). Their general structural features, functions and regulation have been reviewed elsewhere (Wang H. et al., 2020). Though not as extensively studied as the TRPV and TRPM subfamilies, over 30 structures of TRPCs have been determined, covering TRPC3-6 members (Supplementary Appendix Table 1). As shown in Figure 3, ligands in the bound structures of TRPCs mainly cluster into two common binding sites, namely, the portal and VSLD binding sites, as seen in TRPVs and TRPMs (Figures 1, 2). For TRPC4-6, there have been more extensive studies attempting to determine the ligand-bound structures, including both activators and inhibitors. Only one structure of the agonist (AM-0883)-bound human TRPC6 was captured a more open state among all reported TRPC structures. The binding of the AM-0883 in the portal site, situated between the S6 of one monomer and the pore helix (PH) of another, tilts S6 as well as the VSLD and S4-S5 linker, suggesting a further rotation of S6 to release the hydrophobic seal in the open state pore of TRPC6 (Bai et al., 2020). Interestingly, the portal site also accommodates inhibitors HC-070 and HC-608 in TRPC5 and lipids in TRPC3 (Wright et al., 2020; Song et al., 2021). A second highly populated binding site among TRPC4-6 is the VSLD pocket. There are a handful of inhibitors and an activator that bind to this pocket (Bai et al., 2020; Vinayagam et al., 2020; Song et al., 2021; Guo et al., 2022; Yang et al., 2022; Figure 3). The activator/inhibitor pair riluzole and clemizole have been determined to bind to this pocket in TRPC5. These ligands are used pharmacologically to combat amyotrophic lateral sclerosis (ALS) and anxiety and depression, respectively (Song et al., 2021; Yang et al., 2022). The vanilloid-like pocket prominent in TRPV and TRPM channels has been observed to be occupied by [2-(1,3-benzodioxol-5-ylamino)-1,3-thiazol-4-yl]-[(3R,5S)-3,5-dimethylpiperidin-1-yl]methanone (BTDM) in TRPC6 (Figure 3; Guo et al., 2022). TRPC3 has only been resolved in the closed state(s) with observed binding by unidentified lipids in a binding pocket between S1 and the pre-S1 elbow (Fan et al., 2018) and by Ca2+ in several intracellular regions (Guo et al., 2022; Figure 3).
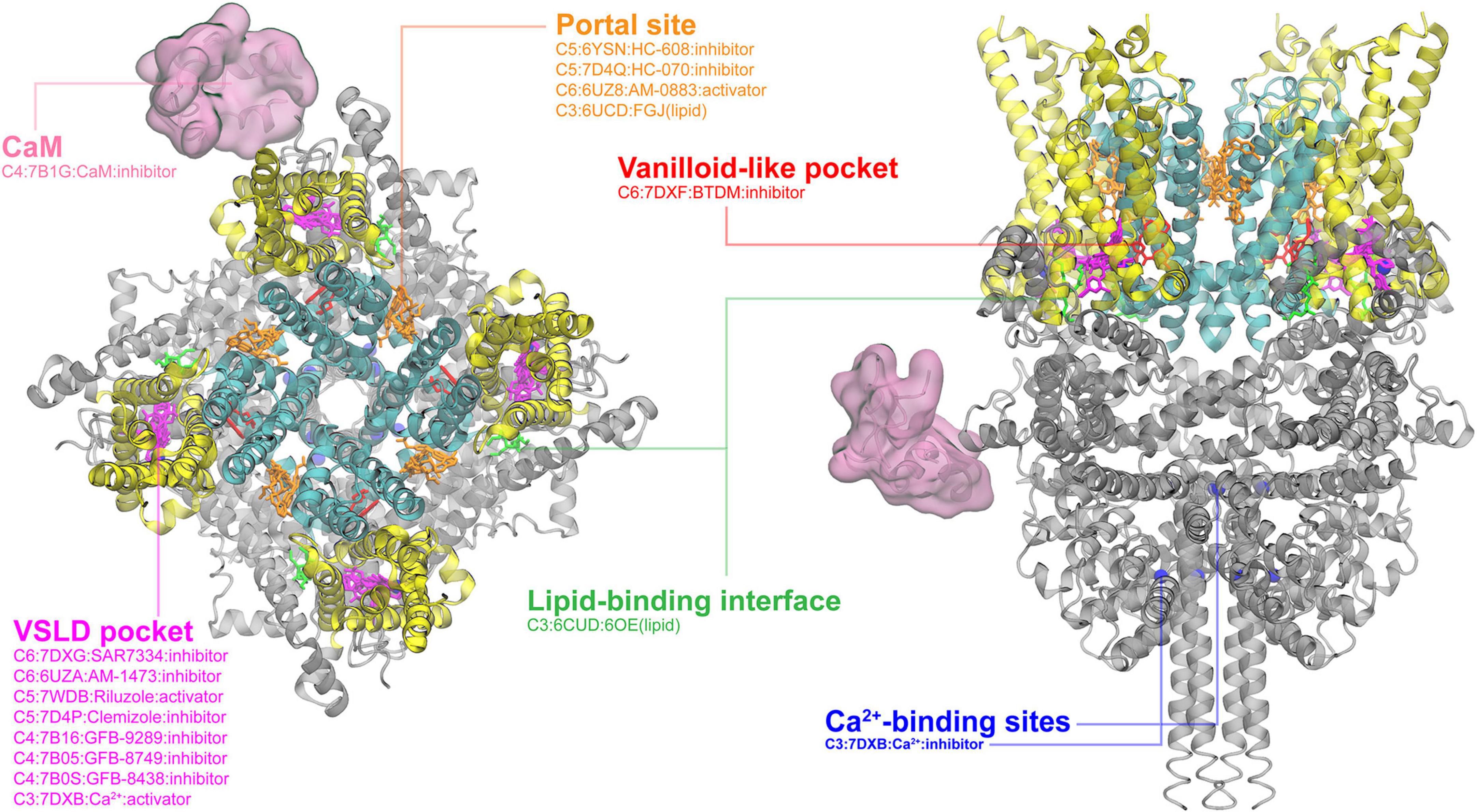
Figure 3. Ligand binding sites in TRPC channels. The SAR7334-bound TRPC structure (PDB: 7DXG) is used for visualization, with the pore region, the VSLD region and cytosolic domain colored in cyan, yellow, and gray, respectively. The major binding pockets or interfaces are highlighted using different colors. The Ca2+ binding sites (blue) are also included because Ca2+ plays a regulation role in the TRPC3 activity. The top and side views are rendered in the same way as in the TRPV channel (see Figure 1).
TRPA1
TRPA1 is the sole member of the TRPA subfamily, characterized by its 16 ankyrin repeats (the longest among TRP channels) and a TM region is structurally very similar to TRPVs (Paulsen et al., 2015). Due to its previously observed pain- and irritant-sensitivity, it has been studied extensively with various ligands (Meents et al., 2019). TRPA1 structure usually could be divided into three layers with the top, middle and bottom layers consisting of the TM domain, the coupling domain and the ankyrin repeat domain, respectively. As shown in Figure 4, all cryo-EM-resolved ligand-bound structures together show the four familiar binding sites as already discussed above: the vanilloid-like site, the VSLD pocket, the portal site and the pre-S1/S4-5 loop/TRP helix interface, in addition to a unique coupling domain pocket. The portal site, formed by S5/S6/PH, can bind with the GDC-0334 inhibitor, which reduces airway inflammation as asthma treatment (Balestrini et al., 2021). Interestingly, a separate study of compound-21 (C21) has also shown to reduce airway inflammation, though by binding to the pre-S1/S4-5 linker/TRP box interface (Terrett et al., 2021), which is often occupied by regulatory lipids in TRPM and TRPV channels. GNE551, an agonist of TRPA1, binds in the vanilloid-like pocket, though still resulting in a non-conductive state of TRPA1 (Liu et al., 2021). The most unique binding site of TRPA1 is the coupling domain pocket (Figure 4). The coupling domain pocket is believed to be important for the “electrophile sensing” of TRPA1 due to the presence of several cysteine residues (Bahia et al., 2016). TRPA1 is a great example of the structural similarities and differences among the TRP subfamilies that allow both conserved and unique binding pockets.
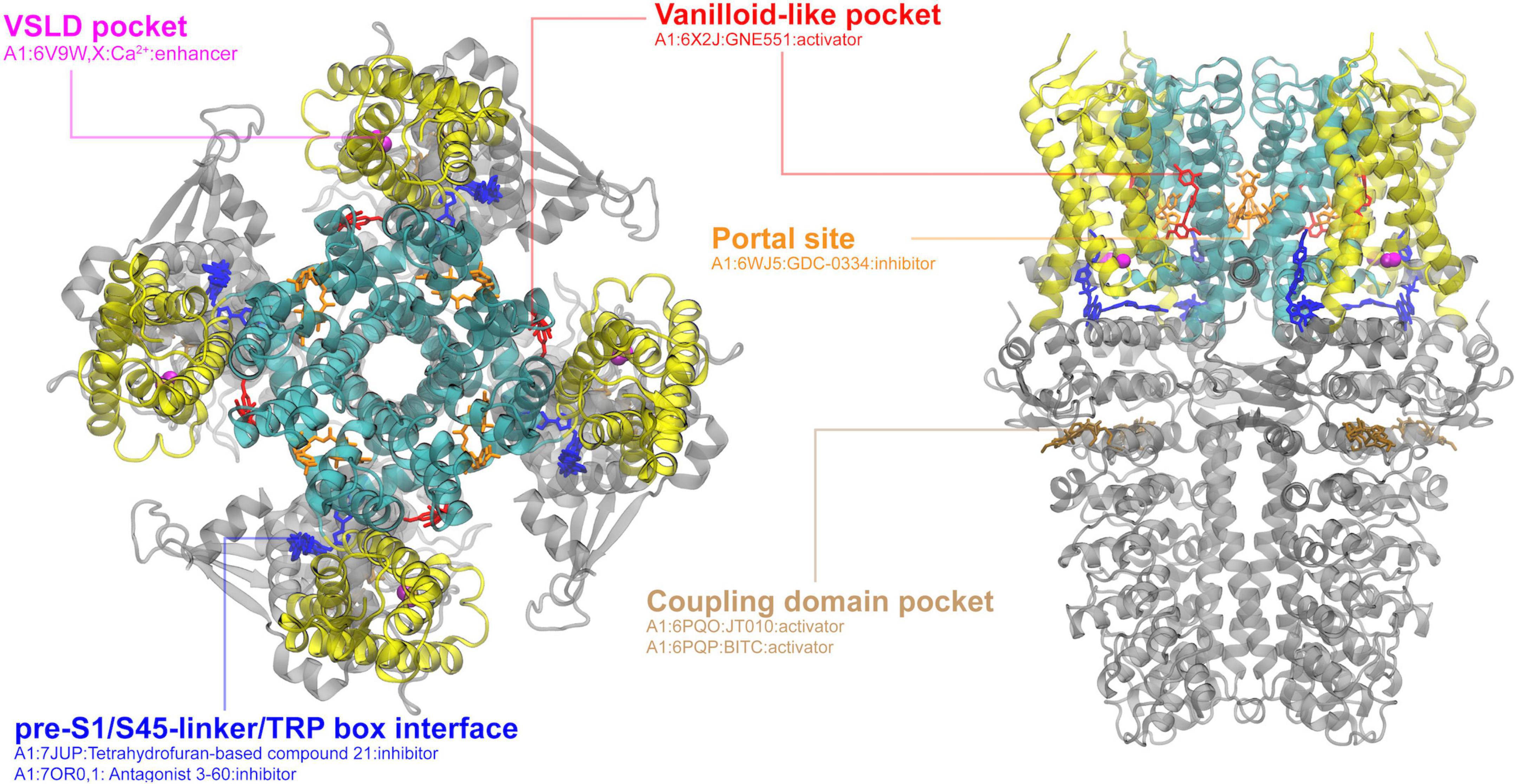
Figure 4. Ligand binding sites in the TRPA1 channel. The structure in complex with covalent agonist JT010 (PDB: 6PQO) is used for visualization, with the pore region, the VSLD region and cytosolic domain colored in cyan, yellow, and gray, respectively. The major binding pockets or interfaces are highlighted using different colors. The top and side views are rendered in the same way as in the TRPV channel (see Figure 1).
TRPMLs
Primarily localized in the endolysosomal membrane of mammalian cells, TRPML is one of the least studied TRP subfamilies (Zhang X. et al., 2018). Cryo-EM structures of all members of the TRPML family have been resolved, but only TRPML1 has solved structures in both open and closed states with various lipids and ligands present. As a homotetrameric Ca2+-permeable, nonselective, cation channel, TRPML1 regulates lysosomal calcium signaling, lipid trafficking, and autophagy-related processes. As such, loss-of-function mutation of TRPML1 is associated with a neurodegenerative disorder, known as Mucolipidosis type IV (MLIV) (Schmiege et al., 2021). As shown in Figure 5, current cryo-EM structures enriched all ligands or internal mediators (such as PIP2 molecules) into two major binding pockets: the VSLD pocket and the portal site. Lipids PI(3,5)P2 and PI(4,5)P2 both have been resolved to bind in the VSLD pocket, where polar and hydrophilic residues are distributed similar to the corresponding pocket in other TRP channels (see above). Interestingly, PI(3,5)P2 has been observed to promote channel opening, while PI(4,5)P2 can act as channel suppressor (Fine et al., 2018). It was proposed that due to the different phosphate group locations, R403 and Y355 are positioned to promote pi-cation interactions in PI(3,5)P2 but not PI(4,5)P2, affecting the movement of the S4-5 linker and further facilitating pore opening (Fine et al., 2018). The portal site in TRPML1, which is similar to the one in TRPV channels, is formed by the TM interface between S5 of one domain and the neighboring S6. It hosts both the agonist ML-SA1 and antagonists ML-SI3 and temsirolimus through mostly hydrophobic interactions (Fine et al., 2018; Schmiege et al., 2021). ML-SA1 was observed, unlike ML-SI3, to promote pi-pi interactions in the portal site, pulling S6 away from the central axis and thus opening the pore (Fine et al., 2018). A similar open state was observed with temsirolimus binding in tandem with PI(3,5)P2 as was observed with ML-SA1 alone (Gan et al., 2022). Interestingly, the VSLD pocket and the portal site in TRPML1 have been shown to both independently and synergistically modulate the channel, with PI(3,5)P2 binding observed even in a ML-SI3 bound state (Schmiege et al., 2021; Gan et al., 2022).
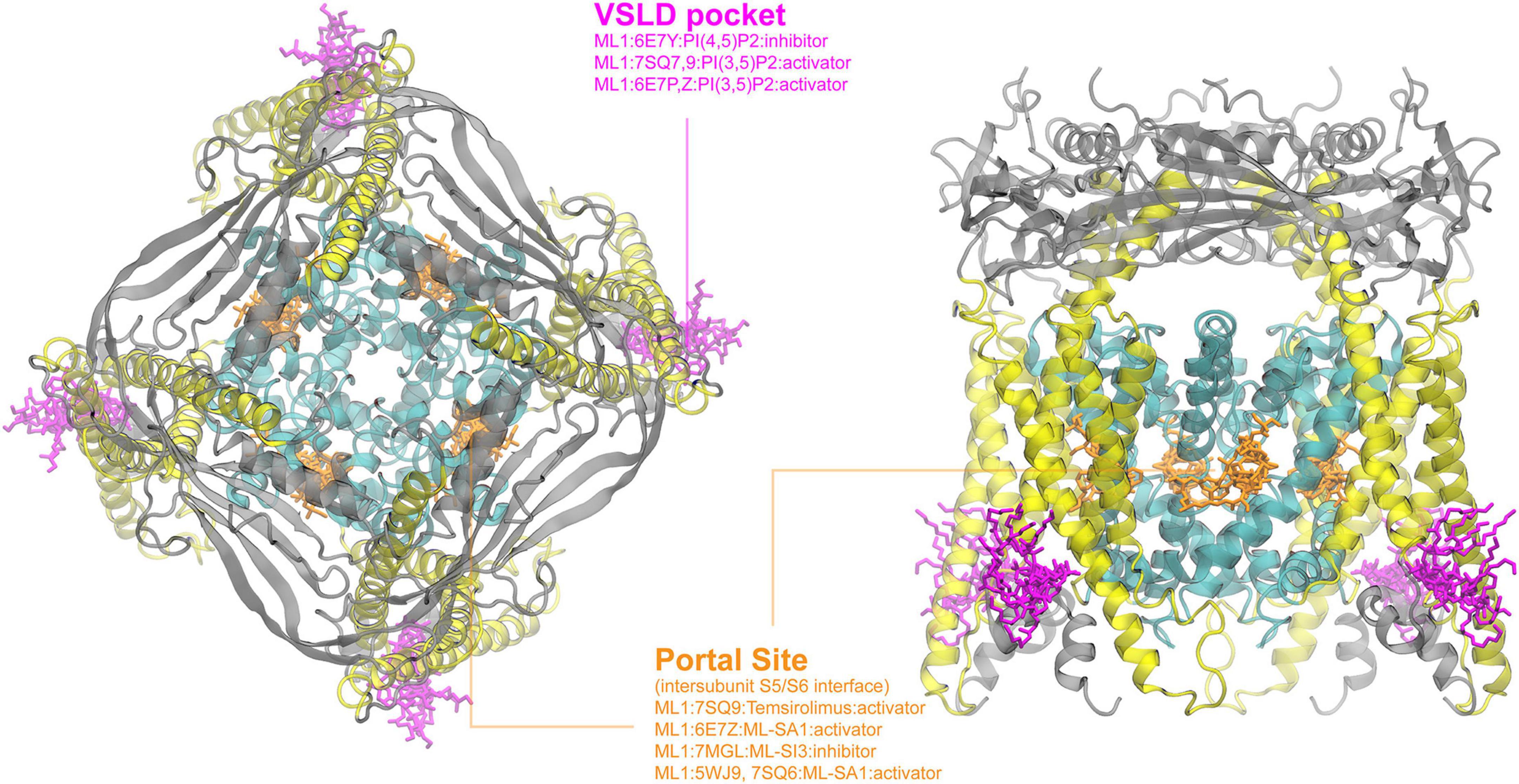
Figure 5. Ligand binding sites of TRPML channels. The PI(4,5)P2-bound TRPML1 structure (PDB: 6E7Y) is used for visualization, with the pore region, the VSLD region and extracellular domains colored in cyan, yellow, and gray, respectively. The top (left) and side (right) views are rendered as described in Figure 1. Existing complex structures of TRPML only show two major binding pockets.
TRPPs
As the most primitive and ancient member of the TRP family, TRPP family was found to present in both animals and yeast, and mutations implicate the autosomal dominant polycystic kidney disease (ADPKD) (Gees et al., 2012; Samanta et al., 2018). TRPP2 (polycystin-2, polycystin kidney disease-2 or PKD2) and TRPP3 (polycystin-2 like, or PKD2L1) are Ca2+-activated cation channels which are structural homologous to other TRP ion channels in terms of the six transmembrane helices. Structural studies have revealed cryo-EM structures of TRPP2 and TRPP3 either in the apo state or in the PIP2-bound state (Supplementary Appendix Table 1). Unfortunately, the PI(4,5)P2 and PI(3,5)P2 were not well-resolved based on cryo-EM density maps of TRPP2 (Wang Q. et al., 2020). Despite this ambiguity, the authors found the density of those PIP2 molecules to be located at the vanilloid-like pocket (Wang Q. et al., 2020). The structural information is still very limited to have a better understanding of ligand binding in TRPP ion channels and thus requires further investigation, though the conserved six transmembrane helices structure suggests that the common binding sites comprised of elements from the TM region discussed in all the above families might be also very likely the binding sites in TRPP channels.
Molecular mechanisms of TRP activation and regulation
The remarkable sensory roles of TRP channels are conferred by the complex dynamic properties of the protein conformations and how they can be delicately controlled by various physical and chemical stimuli. A deep understanding of the molecular mechanisms of these controls is critical for successful rational approaches targeting TRP channels. Activation of an ion channel can be divided into three general steps. First, the “sensor” domain or element needs to respond to the given stimuli, which typically involve certain local conformational changes and movements. Second, the conformational response of the sensor, which is typically distal from the ion-conducting pore, needs to be transduced to the pore domain through intramolecular coupling pathway(s). Lastly, the pore needs to undergo to an opening transition and release the gating element for ion permeation. A drug molecule could interfere with any or all of these three steps of channel activation. The abundant structural data on TRP channels have provided a solid basis for understanding the inner work of these channels. However, a recent global analysis of TRP channel TM domain structures revealed that most available structures represent non-conducting states, leaving much to be learned about the gating transitions alone (Huffer et al., 2020). Even more questions remain to be answered regarding the identities and movements of the sensors as well as the sensor-pore coupling. Below, we summarize the current understanding of the three general steps in TRP channel activation.
Sensor elements in the TRP channels
The sensory roles of TRP channels are well-documented in term of somatosensation. TRPV1-4, TRPM2, TRPM3 and TRPM5 have been reported to be heat sensing, whereas TRPC5, TRPM8 and TRPA1 can be cold sensing (Talavera et al., 2005; Wetsel, 2011; Voets, 2014; Wang and Siemens, 2015; Kashio and Tominaga, 2022). TRPVs and TRPA1 channels can sense touch, pain and itch (Mickle et al., 2015; Sun and Dong, 2016; Moore et al., 2018). In addition, TRPV4 has been shown to be capable of mechanosensation, including osmo-sensation (Christensen and Corey, 2007). Sensation of the redox status has also been reported for TRPC5, TRPV1 and TRPA1 (Takahashi and Mori, 2011; Ogawa et al., 2016). In addition to the physical stimuli, many chemicals, synthetic and natural, can regulate TRP channel functions, as extensively discussed above.
Thermo-sensor
Extensive efforts have been dedicated to pinpoint the thermo-sensor elements of the thermo-TRPs. Many candidates have been evaluated through deletion, mutagenesis and chimeragenesis, so far without reaching a conclusive identification (Diaz-Franulic et al., 2021; Luu et al., 2023). Temperature sensing elements have been proposed throughout the channel structure including: the ankyrin repeat domain for TRPA1 (Cordero-Morales et al., 2011) and TRPV1 (Saito et al., 2016; Ladrón-de-Guevara et al., 2020; Hori et al., 2023), a membrane proximal domain (the N-terminal region connects ankyrin repeats to the S1 helix) for TRPV1-V3 (Yao et al., 2011; He et al., 2017; Liu and Qin, 2021), the whole VSLD for TRPV1 (Kim et al., 2020), the pore turret (Yang et al., 2010; Cui et al., 2012; Du et al., 2020) [although contradicting with a study showing the torrent-deleted TRPV1 remains thermosensitivity (Liao et al., 2013)], the pore helix domain for TRPV1 (Myers et al., 2008) and TRPA1 (Wang et al., 2013), a loop after the pore helix plus the S6 helix for TRPV3 (Grandl et al., 2008), the outer pore loop region for TRPV1 (Grandl et al., 2010), the whole pore domain (S5-S6) for TRPV1 (Zhang F. et al., 2018) and the C-termini for TRPV1 (Vlachová et al., 2003; Brauchi et al., 2006; Joseph et al., 2013). These studies have been plagued by the different experimental conditions/procedures being employed and ambiguity in interpretation. It is possible that there is no single thermo-sensing element in a given TRP channel; instead, the temperature driven conformational transition may emerge from the cooperative property of the entire oligomer assembly within its native membrane environment.
On the other hand, it was also proposed that the thermosensitivity of the thermoTRP channels may not be necessarily attributed to a specific sensor element or domain (Clapham and Miller, 2011; Yeh et al., 2023). Instead, thermoTRP channel activation may be accompanied by large molar heat capacity differences, such that both the activation enthalpy and entropy would be both temperature dependent and the temperature dependence of the open-close equilibrium would be always non-monotonic. Such a model could give rise to both cold and hot activation behaviors, depending on temperature where the open-close equilibrium constant minimizes. It was further proposed that a major contribution to the molar heat capacity is solvation or desolvation of hydrophobic residues and charged ones during activation, which could be delocalized throughout the whole channel protein. This model has been successfully applied to rationally engineer a canonical voltage-sensing potassium channel to confer temperature sensitivity, by varying the polarity of residues in the VSD that undergoes state-dependent changes in solvation (Chowdhury et al., 2014). The molecular basis for the successful design was further confirmed by NMR and molecular dynamics simulations, which reveal increased hydration in the VSD of the engineered channel at high temperatures (Chen et al., 2021).
It is worthy of noticing that cryo-EM studies of TRPV3 have revealed the closed, the heat-induced sensitized state as well as the open state, providing a structural foundation for understanding the molecular mechanism of temperature-sensing (Singh et al., 2019; Nadezhdin et al., 2021b). Comparison of those different states revealed a mutually dependent conformational wave, which involved secondary structural rearrangements of the S2-3 linker and N-/C-termini, and rigid body translational movements involving ARD, the S4-5 linker and pore domain helices (Nadezhdin et al., 2021b). It was thus proposed that the distributed multi-domain conformational wave could be triggered at any localized “sensor” within the wave itself (Nadezhdin et al., 2021b). Nevertheless, heat-induced secondary structure rearrangements could shape the energetics of close-to-open equilibrium. For example, exposure of 10∼15 hydrophobic residues per subunit, to give a ΔH value as large as ∼90 kcal/mol (Nadezhdin et al., 2021b). Nonetheless, it remains challenging to dissect the contributions of individual regions involved in the conformational wave and to explore whether the heat capacity differences in thermoTRP channels can be attributed to localized thermo-sensors or it arises from delocalized contributions throughout the channel.
pH-sensor
pH, as one important aspect of the physiological conditions for living cells, has shown to regulate many TRP channels. TRPV1 was the first TRP channel found to be potentiated and even directly activated by extracellular acidification (Caterina et al., 1997; Tominaga et al., 1998; Baumann and Martenson, 2000). Two extracellular Glu residues (E600 and E648, located at the pore turret and the pre-S6 loop, respectively) were proposed to be the pH-sensing elements of the pH-induced potentiation and activation in TRPV1 (Jordt et al., 2000). Another study suggests that F660 located in the pore domain is the key pH-sensor of TRPV1 (Aneiros et al., 2011). Extracellular protons have also been shown to activate TRPV4 (Suzuki et al., 2003), and potentiate TRPA1 (Takahashi et al., 2008; de la Roche et al., 2013), TRPM6 (Li et al., 2007), TRPM7 (Jiang et al., 2005; Li et al., 2007; Numata and Okada, 2008), TRPC4 (Semtner et al., 2007), TRPC5 (Semtner et al., 2007; Kim et al., 2008) and TRPP3 (Inada et al., 2008). Negatively charged residues (Asp or Glu) in the pore turret, the pre-S6 loop as well as the selectivity filter have been frequently proposed to be the pH-sensing elements in these channels (Zheng, 2013), although specific locations of those charge residues may differ greatly among those members. Extracellular protons could also be inhibitory for some TRP channels, including TRPV3 (Wang et al., 2021), TRPV5 (Yeh et al., 2003), TRPM2 (Du et al., 2009), TRPM5 (Liu et al., 2005), and TRPC6 (Semtner et al., 2007).
Furthermore, intracellular alkalization can activate TRPV1 with H378 in the ARD proposed to be the sensor (Dhaka et al., 2009). Intracellular proton-induced potentiation and activation was also reported in TRPV3 involving a N-terminal H426 (Cao et al., 2012) or the S2-3 linker (Gao L. et al., 2016), whereas intracellular proton-induced inhibition has been observed for TRPV5 involving a proximal C-terminal K607 (Yeh et al., 2005) and TRPM2 involving the S4-5 linker (Du et al., 2009).
Although there is some consensus in terms of pH-sensors in individual TRP members, the proposed key residues are often scattered in both the extracellular and intracellular domains. The implication is that TRP channels do not share conserved pH-sensing elements or mechanism. More studies are required to elucidate the molecular mechanisms of proton regulation in TRP channels.
Mechanosensor
The nature and location of the mechanosensor domain in mechanosensitive TRP channels (for example, TRPV4, TRPA1, TRPC1, TRPC6, and TRPP2) also remain largely elusive (Lin and Corey, 2005; O’Neil and Heller, 2005). Several proposals have been discussed. For example, the ankyrin repeats domain in the TRP channels was proposed to function like a molecular “spring” during the mechanical force-induced gating (Corey et al., 2004; Howard and Bechstedt, 2004). Further, it has been debated whether a TRP channel is directly transducing mechanical signals or it is indirectly regulated by being a downstream receptor of the signaling pathway (Christensen and Corey, 2007). Evidence has suggested that epoxyeicosatrienoic acids, a type of cellular secondary messenger, can directly activate TRPV4 (Vriens et al., 2005). Very recently, two independent cryo-EM studies captured the human TRPV4-RhoA (a small GTPase) complexes showing RhoA interacts extensively with the ARD domain (Kwon et al., 2023; Nadezhdin et al., 2023b). Given that RhoA is a membrane-anchoring protein, it is possible that RhoA plays a role in connecting or transducing membrane surface or morphological changes to the TRPV4 channel (Kwon et al., 2023; Nadezhdin et al., 2023b). More intensive studies are required to dissect the role of mechanosensitive TRP channels in mechanical transduction and the possible existence of mechanosensor domains.
Chemosensor
As a sole member in the TRPA subfamily, TRPA1 has long been recognized as the “chemonociceptor” due to its ability to “sense” a wide range of noxious chemical compounds or environmental irritants (Meents et al., 2019; Manolache et al., 2021). Mechanistically, TRPA1 can be activated by thio-reacting electrophile irritants using an array of cysteine residues loaded at the N-terminal domain (Hinman et al., 2006; Macpherson et al., 2007; Bahia et al., 2016), and also binds non-covalently with other non-reactive chemicals in a way of using the traditional binding pocket(s) (Xu et al., 2006; Karashima et al., 2007; Lee et al., 2008; Liu et al., 2021). The chemosensor of TRPA1 discussed here only refers to the covalent binding module. Several functional studies have consistently revealed that highly reactive C621 (human TRPA1 numbering, uniprot: O75762) plays a key role in covalent binding of electrophiles, though inconsistency exists with other potential cysteine sites based on mutagenesis data (Hinman et al., 2006; Macpherson et al., 2007; Bahia et al., 2016, p. 621). The role of C612 as the “chemosensor” element of TRPA1 has been further supported by cryo-EM structures showing agonist-modified C612 (Suo et al., 2020; Zhao J. et al., 2020).
Mechanism of sensor-pore coupling during TRP channel activation
At present, there are relatively limited studies on the sensor-pore coupling mechanisms of the TRP channels, to a large degree due to much ambiguity in the sensor elements (see above). Existing analysis is largely based on structural data alone. Here, we highlight two conformational switches that have been the most extensively investigated.
C-terminal switch
An interesting structural rearrangement during the close/open transition found primarily in thermoTRPVs (TRPV1-V4) involves a loop-to-helix transition in the C-terminus, termed “C-terminal switch” (Zubcevic et al., 2019; Deng et al., 2020). TRPV channels share a C-terminal domain (CTD) following the TRP helix, which coils back to the coupling domain (defined as the domain from right after ankyrin repeat 6 to the pre-S1 helix), forming an interacting network with the neighboring ankyrin repeat domain (Cao, 2020; Pumroy et al., 2020). The CTD was proposed to be involved in temperature-induced gating in several cases (Vlachová et al., 2003; Brauchi et al., 2004, 2006, 2007). The C-terminal switch was discovered in the structural studies of a sensitized phenotype (the K169A mutant) in the human TRPV3 that breaks an important salt bridge between CTD and the neighboring ARD (Zubcevic et al., 2019). The K169A mutation induces distal CTD to undergo coil-to-helix transition, altering the position of CTD and the interactions at the inter-protomer interface. It was thus proposed that the C-terminal loop-to-helix transition represents a functional “switch” during TRPV channel gating (Zubcevic et al., 2019). Similar helical CTD was also observed in the rat TRPV2 for both the apo and the agonist-bound (cannabidiol) structures (Pumroy et al., 2019). Besides, in the recently resolved TRPV4-RhoA complex, binding of 4-αPDD also triggers the C-terminus transit from a loop to a α-helix in the captured open state (Nadezhdin et al., 2023b). In another study of mouse TRPV3, although the distal CTD was assigned as a loop in the open state, it was proposed to be a “latch” that needs to be released and unwrapped from the N-terminal beta-sheet to sensitize the channel (Singh et al., 2019). A recent cryo-EM structure of squirrel TRPV1 also revealed that the C-terminus “hook” wraps around the interdomain N-terminal beta-sheet (Nadezhdin et al., 2021a). In many other cases, the CTD was not well-resolved in thermoTRPV channels, due to either limitation of the cryo-EM resolution or the inherent structural flexibility. Nonetheless, it has been suggested that the CTD conformational transition plays in the temperature-induced gating of TRP channels.
Coupling of the S6 and TRP helices
Changes in the S6 and TRP helix coupling have also been observed in the ligand-induced open/close transitions of the TRP channels. This type of transition usually involves elongation of the S6 helix and/or shortening of the TRP helix. It was firstly discovered in the captured open state of TRPV3 where the S6 helix is elongated by two helical turns compared with the closed state (Singh et al., 2018). A similar pattern was discovered later in ligand-induced activation of the human TRPV3 (Zubcevic et al., 2019), temperature-induced opening of the mouse TRPV3 (Singh et al., 2019) and more recently in the gain-of-function mutation-induced opening of the mouse TRPM7 (Nadezhdin et al., 2023a). Besides, structural determination of PIP2-bound TRPV5 revealed that PIP2 binding induces lengthening of the S6 helix for about 1 helical turn and shortening of the TRP helix in order to form favorable salt bridge interactions with PIP2 head group (Hughes et al., 2018). Changes on the helix rearrangement between the S6 and TRP helix will likely alter the pore-sensor coupling upon stimulation of the channel, which often swivels or “pulls” the S6 helix outward to open the central pore (Hughes et al., 2018; Zubcevic et al., 2019; Nadezhdin et al., 2023a). It is worth noting that the C-terminal switch and coupling of S6-TRP helices are not mutually exclusive during the close/open transitions (Hughes et al., 2018; Zubcevic et al., 2019).
How does the pore open: gating of TRP channels
Generally speaking, TRP channels can have two constriction regions that may serve as the gate – the selectivity filter region and the bundle-crossing formed by the pore-lining S6 helices. The existence of a selectivity filter gate varies among subfamilies. The selectivity filter of TRPV1-3 allows ions to enter the pore even in the inactive state, indicating that the selectivity filter might not be a gate (Jara-Oseguera et al., 2019). Global structure alignments showed that the selectivity filter regions of nonselective TRP channels have large variations on their radii of opening, indicative of significant intrinsic flexibility (Huffer et al., 2020). Selective TRP members, such as TRPV5-6 and TRPM4-5, tend to contain narrower filter regions compared with nonselective ones (Huffer et al., 2020). Further, it has been shown that the cytosolic S6 activation gate formed by bundle-crossing (also being called “hydrophobic seal”) is a consistent feature among most all TRP channels (Cao, 2020; Huffer et al., 2020; Pumroy et al., 2020). Additional contribution to TRP channel gating may come from the hydrophobic inner pore region between the bundle-crossing and selectivity filter, which may undergo spontaneous dewetting transitions and form a vapor barrier to block ion permeation (Huang and Chen, 2023). An important caveat is that most currently resolved TRP structures, either with ligand bound or not, have a rather narrow cytosolic gate and thus represent a closed pore (Huffer et al., 2020), which present extra challenges in delineating the gating mechanisms.
One of the more notable and general structural elements in the gating of TRP channels is probably the π-helix in a single turn of the S6 helix. Since many TRP channels form a “bundle-crossing” lower gate, it was proposed that the flexibility of the π-helical turn in the middle of the S6 helix allows bending of the S6 helix and thus may enable channel opening. The π-helices resemble the glycine hinge or the proline hinge discovered previously in potassium channels. As the TM regions of TRP channels are highly conserved, almost all TRP subfamilies can find cases exhibiting state-dependent π-helices in their captured structures in either closed, sensitized or open states (Zubcevic and Lee, 2019). TRPV6 is an exemplary case where the α-to-π transition at an alanine hinge of the S6 helix dictates the close-to-open conformational transition (McGoldrick et al., 2018). The π-helix can induce bending and rotation of S6, changing the pore-lining residues to create a more ion-favorable hydrophilic environment (McGoldrick et al., 2018). In TRPV3, the α-to-π transition occurs during ligand-induced sensitization, which widens the pore slightly and exposes different groups of pore-lining residues (Singh et al., 2018; Zubcevic et al., 2018). Studies have also found some TRP members can also have π-to-α transition going from closed state to sensitized or open state, such as the most recently reported TRPV4 (Nadezhdin et al., 2023b), whereas some TRP channels exhibit π-helix in the S6 helix in both the closed and open state, including the most recent case of TRPV3 and TRPM7 (Zubcevic and Lee, 2019; Nadezhdin et al., 2021b,2023a). It is worth noting that a conserved feature among all TRP channels is that the pore-lining residues in the inner pore region of S6 in the closed state are usually hydrophobic ones, forming a so-called “hydrophobic seal.” For example, the lower portion of S6 contains a highly conserved sequence, LLLNMLI, among TRPV1-4. The hydrophobic lower pore region present in the deactivated state suggest a general role of hydrophobic gating mechanism among TRP channels (Aryal et al., 2015; Yazdani et al., 2020). The dewetting transition involved in hydrophobic gating can be readily controlled by α-to-π or π-to-α transitions modifying the pore geometry and surface hydrophobicity.
Concluding discussion
As multifunctional proteins intimately involved in diverse physiological processes, TRP channels are considered exciting and potentially rewarding therapeutic targets. Many modulators of this ion channel family are under development, and several have reached clinical trials (Moran et al., 2011; Moran and Szallasi, 2018; Iftinca et al., 2021; Koivisto et al., 2022). At the same time, TRP channels have presented critical challenges due to their complex, polymodal activation and regulation and complex roles in physiological functions, which frequently leads to potential issues with clinical efficacy, safety and side effects. Overcoming these issues has been plagued by important gaps in the current understanding of TRP channel function at the molecular level. While hundreds of structures of are now available for TRP channels in both apo and bound states, they alone do not readily reveal functional mechanisms. For example, only a handful of the “open” structures represent truly conductive states (Huffer et al., 2020). Many agonists and antagonists can bind to similar pockets, without leading to apparent conformational changes in the channel protein. We still do not have a concrete understanding of how the TRP channels may sense temperature, mechanical force, osmotic pressure or voltage. These critical gaps in the fundamental understanding of the TRP channel function make it extremely challenging for any rational attempt to optimize lead chemical matters or discover novel ones.
Notwithstanding many important challenges, intensive research into the molecular basis of the TRP channel function has generated a rich set of structural and functional data. As summarized in this review, high resolution structures are now available for all subfamilies of TRP channels, often times in multiple functional states and/or several ligand-bound states. Some mechanistic features of TRP channel gating are also emerging, such as the α-to-π transition of pore-lining S6 helix and the potential role of hydrophobic gating. These structures together reveal major binding pockets present in the TRP channels. Multiple binding sites, inside and outside of the membrane bilayer, have been identified for some members of this family with some pockets seemingly more druggable than others. In some TRP channels, the only identified pockets reside deep in the membrane, which can lead to challenges in identifying development candidates with drug-like physicochemical properties. Low solubility and permeability may result in poor bioavailability, limiting the effectiveness of the drug. There is an urgent need and exciting opportunity to leverage this rich set of structural and functional data to further elucidate the three general steps of channel activation and regulation, namely, sensor movements, sensor-pore coupling, and pore opening transitions. This will require concerted efforts from computation, structural biology, medicinal chemistry, electrophysiology, and pharmacology. An ever improving understanding the channels’ activation and regulatory mechanisms will guide the drug design efforts and open new possibilities and venues for targeting the TRP channels in therapeutics.
Author contributions
JH: Writing—review and editing, Data curation, Visualization, Writing—original draft. AK: Data curation, Visualization, Writing—original draft. MY: Conceptualization, Writing—original draft, Writing—review and editing. JC: Conceptualization, Funding acquisition, Writing—review and editing.
Funding
The author(s) declare financial support was received for the research, authorship, and/or publication of this article. This work was supported by the National Institutes of Health Grant R35 GM144045 (to JC).
Conflict of interest
MY was employed by Modeling and Informatics, Merck & Co., Inc., United States.
The remaining authors declare that the research was conducted in the absence of any commercial or financial relationships that could be construed as a potential conflict of interest.
The author(s) declared that they were an editorial board member of Frontiers, at the time of submission. This had no impact on the peer review process and the final decision.
Publisher’s note
All claims expressed in this article are solely those of the authors and do not necessarily represent those of their affiliated organizations, or those of the publisher, the editors and the reviewers. Any product that may be evaluated in this article, or claim that may be made by its manufacturer, is not guaranteed or endorsed by the publisher.
Supplementary material
The Supplementary Material for this article can be found online at: https://www.frontiersin.org/articles/10.3389/fnmol.2023.1334370/full#supplementary-material
References
Abriel, H., Syam, N., Sottas, V., Amarouch, M. Y., and Rougier, J.-S. (2012). TRPM4 channels in the cardiovascular system: physiology, pathophysiology, and pharmacology. Biochem. Pharmacol. 84, 873–881. doi: 10.1016/j.bcp.2012.06.021
Ali, F. T., El-Azeem, E. M. A., Hekal, H. F. A., El-Gizawy, M. M., Sayed, M. S., Mandoh, A. Y., et al. (2022). Association of TRPV5, CASR, and CALCR genetic variants with kidney stone disease susceptibility in Egyptians through main effects and gene–gene interactions. Urolithiasis 50, 701–710. doi: 10.1007/s00240-022-01360-z
Almidani, E., Elsidawi, W., Almohamedi, A., Bin Ahmed, I., and Alfadhel, A. (2020). Case report of transient neonatal hyperparathyroidism: medically free mother. Cureus 12:e7000. doi: 10.7759/cureus.7000
Andrè, E., Gatti, R., Trevisani, M., Preti, D., Baraldi, P. G., Patacchini, R., et al. (2009). Transient receptor potential ankyrin receptor 1 is a novel target for pro-tussive agents. Br. J. Pharmacol. 158, 1621–1628. doi: 10.1111/j.1476-5381.2009.00438.x
Aneiros, E., Cao, L., Papakosta, M., Stevens, E. B., Phillips, S., and Grimm, C. (2011). The biophysical and molecular basis of TRPV1 proton gating. EMBO J. 30, 994–1002. doi: 10.1038/emboj.2011.19
Aryal, P., Sansom, M. S., and Tucker, S. J. (2015). Hydrophobic gating in ion channels. J. Mol. Biol. 427, 121–130. doi: 10.1016/j.jmb.2014.07.030
Audo, I., Kohl, S., Leroy, B. P., Munier, F. L., Guillonneau, X., Mohand-Saïd, S., et al. (2009). TRPM1 is mutated in patients with autosomal-recessive complete congenital stationary night blindness. Am. J. Hum. Genet. 85, 720–729. doi: 10.1016/j.ajhg.2009.10.013
Bagal, S. K., Brown, A. D., Cox, P. J., Omoto, K., Owen, R. M., Pryde, D. C., et al. (2013). Ion channels as therapeutic targets: a drug discovery perspective. J. Med. Chem. 56, 593–624. doi: 10.1021/jm3011433
Bahia, P. K., Parks, T. A., Stanford, K. R., Mitchell, D. A., Varma, S., Stevens, S. M., et al. (2016). The exceptionally high reactivity of Cys 621 is critical for electrophilic activation of the sensory nerve ion channel TRPA1. J. Gen. Physiol. 147, 451–465. doi: 10.1085/jgp.201611581
Bai, Y., Yu, X., Chen, H., Horne, D., White, R., Wu, X., et al. (2020). Structural basis for pharmacological modulation of the TRPC6 channel. Elife 9:e53311. doi: 10.7554/eLife.53311
Baker, K., Raemdonck, K., Dekkak, B., Snelgrove, R. J., Ford, J., Shala, F., et al. (2016). Role of the ion channel, transient receptor potential cation channel subfamily V member 1 (TRPV1), in allergic asthma. Respir. Res. 17:67. doi: 10.1186/s12931-016-0384-x
Balestrini, A., Joseph, V., Dourado, M., Reese, R. M., Shields, S. D., Rougé, L., et al. (2021). A TRPA1 inhibitor suppresses neurogenic inflammation and airway contraction for asthma treatment. J. Exp. Med. 218:e20201637. doi: 10.1084/jem.20201637
Bassi, M. T., Manzoni, M., Monti, E., Pizzo, M. T., Ballabio, A., and Borsani, G. (2000). Cloning of the gene encoding a novel integral membrane protein, mucolipidin-and identification of the two major founder mutations causing mucolipidosis type IV. Am. J. Hum. Genet. 67, 1110–1120. doi: 10.1016/S0002-9297(07)62941-3
Baumann, T. K., and Martenson, M. E. (2000). Extracellular protons both increase the activity and reduce the conductance of capsaicin- gated channels. J. Neurosci. 20:RC80. doi: 10.1523/JNEUROSCI.20-11-j0004.2000
Bautista, D. M., Siemens, J., Glazer, J. M., Tsuruda, P. R., Basbaum, A. I., Stucky, C. L., et al. (2007). The menthol receptor TRPM8 is the principal detector of environmental cold. Nature 448, 204–208. doi: 10.1038/nature05910
Belvisi, M. G., and Birrell, M. A. (2017). The emerging role of transient receptor potential channels in chronic lung disease. Eur. Respir. J. 50:1601357. doi: 10.1183/13993003.01357-2016
Bonezzi, C., Costantini, A., Cruccu, G., Fornasari, D. M. M., Guardamagna, V., Palmieri, V., et al. (2020). Capsaicin 8% dermal patch in clinical practice: an expert opinion. Expert Opin. Pharmacother. 21, 1377–1387. doi: 10.1080/14656566.2020.1759550
Bonvini, S. J., Birrell, M. A., Grace, M. S., Maher, S. A., Adcock, J. J., Wortley, M. A., et al. (2016). Transient receptor potential cation channel, subfamily V, member 4 and airway sensory afferent activation: role of adenosine triphosphate. J. Allergy Clin. Immunol. 138, 249–261.e12. doi: 10.1016/j.jaci.2015.10.044
Brauchi, S., Orio, P., and Latorre, R. (2004). Clues to understanding cold sensation: thermodynamics and electrophysiological analysis of the cold receptor TRPM8. Proc. Natl. Acad. Sci. U.S.A. 101, 15494–15499. doi: 10.1073/pnas.0406773101
Brauchi, S., Orta, G., Mascayano, C., Salazar, M., Raddatz, N., Urbina, H., et al. (2007). Dissection of the components for PIP2 activation and thermosensation in TRP channels. Proc. Natl. Acad. Sci. U.S.A. 104, 10246–10251. doi: 10.1073/pnas.0703420104
Brauchi, S., Orta, G., Salazar, M., Rosenmann, E., and Latorre, R. (2006). A hot-sensing cold receptor: C-terminal domain determines thermosensation in transient receptor potential channels. J. Neurosci. 26, 4835–4840. doi: 10.1523/JNEUROSCI.5080-05.2006
Brederson, J.-D., Kym, P. R., and Szallasi, A. (2013). Targeting TRP channels for pain relief. Eur. J. Pharmacol. 716, 61–76. doi: 10.1016/j.ejphar.2013.03.003
Burren, C. P., Caswell, R., Castle, B., Welch, C. R., Hilliard, T. N., Smithson, S. F., et al. (2018). TRPV6 compound heterozygous variants result in impaired placental calcium transport and severe undermineralization and dysplasia of the fetal skeleton. Am. J. Med. Genet. Part A 176, 1950–1955. doi: 10.1002/ajmg.a.40484
Calixto, J. B., Kassuya, C. A. L., André, E., and Ferreira, J. (2005). Contribution of natural products to the discovery of the transient receptor potential (TRP) channels family and their functions. Pharmacol. Ther. 106, 179–208. doi: 10.1016/j.pharmthera.2004.11.008
Cao, E. (2020). Structural mechanisms of transient receptor potential ion channels. J. Gen. Physiol. 152:e201811998. doi: 10.1085/jgp.201811998
Cao, E., Liao, M., Cheng, Y., and Julius, D. (2013). TRPV1 structures in distinct conformations reveal activation mechanisms. Nature 504, 113–118. doi: 10.1038/nature12823
Cao, X., Yang, F., Zheng, J., and Wang, K. (2012). Intracellular proton-mediated activation of TRPV3 channels accounts for the exfoliation effect of α-hydroxyl acids on keratinocytes. J. Biol. Chem. 287, 25905–25916. doi: 10.1074/jbc.M112.364869
Caspani, O., and Heppenstall, P. A. (2009). TRPA1 and cold transduction: an unresolved issue? J. Gen. Physiol. 133, 245–249. doi: 10.1085/jgp.200810136
Caspani, O., Zurborg, S., Labuz, D., and Heppenstall, P. A. (2009). The contribution of TRPM8 and TRPA1 channels to cold allodynia and neuropathic pain. PLoS One 4:e7383. doi: 10.1371/journal.pone.0007383
Caterina, M. J., Schumacher, M. A., Tominaga, M., Rosen, T. A., Levine, J. D., and Julius, D. (1997). The capsaicin receptor: a heat-activated ion channel in the pain pathway. Nature 389, 816–824. doi: 10.1038/39807
Chen, H., Deng, J., Cui, Q., Chanda, B., and Henzler-Wildman, K. (2021). Mapping temperature-dependent conformational change in the voltage-sensing domain of an engineered heat-activated K+ channel. Proc. Natl. Acad. Sci. U.S.A. 118:e2017280118. doi: 10.1073/pnas.2017280118
Cheng, W. W. L., Arcario, M. J., and Petroff, J. T. (2022). Druggable lipid binding sites in pentameric ligand-gated ion channels and transient receptor potential channels. Front. Physiol. 12:798102. doi: 10.3389/fphys.2021.798102
Chowdhury, S., Jarecki, B. W., and Chanda, B. (2014). A molecular framework for temperature-dependent gating of ion channels. Cell 158, 1148–1158. doi: 10.1016/j.cell.2014.07.026
Christensen, A. P., and Corey, D. P. (2007). TRP channels in mechanosensation: direct or indirect activation? Nat. Rev. Neurosci. 8, 510–521. doi: 10.1038/nrn2149
Chubanov, V., Kubanek, S., Fiedler, S., Mittermeier, L., Gudermann, T., and Dietrich, A. (2017). “Renal functions of TRP channels in health and disease,” in Neurobiology of TRP channels, ed. T. L. R. Emir (Boca Raton, FL: CRC Press).
Chung, M.-K., and Campbell, J. N. (2016). Use of capsaicin to treat pain: mechanistic and therapeutic considerations. Pharmaceuticals (Basel) 9:66. doi: 10.3390/ph9040066
Clapham, D. E. (2003). TRP channels as cellular sensors. Nature 426, 517–524. doi: 10.1038/nature02196
Clapham, D. E., and Miller, C. (2011). A thermodynamic framework for understanding temperature sensing by transient receptor potential (TRP) channels. Proc. Natl. Acad. Sci. U.S.A. 108, 19492–19497. doi: 10.1073/pnas.1117485108
Colsoul, B., Nilius, B., and Vennekens, R. (2013). Transient receptor potential (TRP) cation channels in diabetes. Curr. Top. Med. Chem. 13, 258–269.
Cordero-Morales, J. F., Gracheva, E. O., and Julius, D. (2011). Cytoplasmic ankyrin repeats of transient receptor potential A1 (TRPA1) dictate sensitivity to thermal and chemical stimuli. Proc. Natl. Acad. Sci. U.S.A. 108, E1184–E1191. doi: 10.1073/pnas.1114124108
Corey, D. P., García-Añoveros, J., Holt, J. R., Kwan, K. Y., Lin, S.-Y., Vollrath, M. A., et al. (2004). TRPA1 is a candidate for the mechanosensitive transduction channel of vertebrate hair cells. Nature 432, 723–730. doi: 10.1038/nature03066
Cuajungco, M. P., and Samie, M. A. (2008). The varitint-waddler mouse phenotypes and the TRPML3 ion channel mutation: cause and consequence. Pflugers Arch. 457, 463–473. doi: 10.1007/s00424-008-0523-4
Cui, Y., Yang, F., Cao, X., Yarov-Yarovoy, V., Wang, K., and Zheng, J. (2012). Selective disruption of high sensitivity heat activation but not capsaicin activation of TRPV1 channels by pore turret mutations. J. Gen. Physiol. 139, 273–283. doi: 10.1085/jgp.201110724
Dai, J., Cho, T.-J., Unger, S., Lausch, E., Nishimura, G., Kim, O.-H., et al. (2010). TRPV4-pathy, a novel channelopathy affecting diverse systems. J. Hum. Genet. 55, 400–402. doi: 10.1038/jhg.2010.37
Daumy, X., Amarouch, M.-Y., Lindenbaum, P., Bonnaud, S., Charpentier, E., Bianchi, B., et al. (2016). Targeted resequencing identifies TRPM4 as a major gene predisposing to progressive familial heart block type I. Int. J. Cardiol. 207, 349–358. doi: 10.1016/j.ijcard.2016.01.052
de la Roche, J., Eberhardt, M. J., Klinger, A. B., Stanslowsky, N., Wegner, F., Koppert, W., et al. (2013). The molecular basis for species-specific activation of human TRPA1 protein by protons involves poorly conserved residues within transmembrane domains 5 and 6. J. Biol. Chem. 288, 20280–20292. doi: 10.1074/jbc.M113.479337
de Sainte Agathe, J.-M., Van-Gils, J., Lasseaux, E., Arveiler, B., Lacombe, D., Pfirrmann, C., et al. (2020). Confirmation and expansion of the phenotype associated with the recurrent p.Val837Met variant in TRPM3. Eur. J. Med. Genet. 63:103942. doi: 10.1016/j.ejmg.2020.103942
Deng, Z., Maksaev, G., Rau, M., Xie, Z., Hu, H., Fitzpatrick, J. A. J., et al. (2020). Gating of human TRPV3 in a lipid bilayer. Nat. Struct. Mol. Biol. 27, 635–644. doi: 10.1038/s41594-020-0428-2
Dhaka, A., Uzzell, V., Dubin, A. E., Mathur, J., Petrus, M., Bandell, M., et al. (2009). TRPV1 is activated by both acidic and basic pH. J. Neurosci. 29, 153–158. doi: 10.1523/JNEUROSCI.4901-08.2009
Diaz-Franulic, I., Verdugo, C., Gonzalez, F., Gonzalez-Nilo, F., and Latorre, R. (2021). Thermodynamic and structural basis of temperature-dependent gating in TRP channels. Biochem. Soc. Trans. 49, 2211–2219. doi: 10.1042/BST20210301
Diver, M. M., Cheng, Y., and Julius, D. (2019). Structural insights into TRPM8 inhibition and desensitization. Science 365, 1434–1440. doi: 10.1126/science.aax6672
Diver, M. M., Lin King, J. V., Julius, D., and Cheng, Y. (2022). Sensory trp channels in three dimensions. Annu. Rev. Biochem. 91, 629–649. doi: 10.1146/annurev-biochem-032620-105738
Du, G., Tian, Y., Yao, Z., Vu, S., Zheng, J., Chai, L., et al. (2020). A specialized pore turret in the mammalian cation channel TRPV1 is responsible for distinct and species-specific heat activation thresholds. J. Biol. Chem. 295, 9641–9649. doi: 10.1074/jbc.RA120.013037
Du, J., Xie, J., and Yue, L. (2009). Modulation of TRPM2 by acidic pH and the underlying mechanisms for pH sensitivity. J. Gen. Physiol. 134, 471–488. doi: 10.1085/jgp.200910254
Duchatelet, S., Pruvost, S., de Veer, S., Fraitag, S., Nitschké, P., Bole-Feysot, C., et al. (2014). A new TRPV3 missense mutation in a patient with Olmsted syndrome and erythromelalgia. JAMA Dermatol. 150, 303–306. doi: 10.1001/jamadermatol.2013.8709
Dyment, D. A., Terhal, P. A., Rustad, C. F., Tveten, K., Griffith, C., Jayakar, P., et al. (2019). De novo substitutions of TRPM3 cause intellectual disability and epilepsy. Eur. J. Hum. Genet. 27, 1611–1618. doi: 10.1038/s41431-019-0462-x
Eid, S. R., Crown, E. D., Moore, E. L., Liang, H. A., Choong, K.-C., Dima, S., et al. (2008). HC-030031, a TRPA1 selective antagonist, attenuates inflammatory- and neuropathy-induced mechanical hypersensitivity. Mol. Pain 4:48. doi: 10.1186/1744-8069-4-48
Fallah, H. P., Ahuja, E., Lin, H., Qi, J., He, Q., Gao, S., et al. (2022). A review on the role of TRP channels and their potential as drug targets_an insight into the TRP channel drug discovery methodologies. Front. Pharmacol. 13:914499. doi: 10.3389/fphar.2022.914499
Fan, C., Choi, W., Sun, W., Du, J., and Lü, W. (2018). Structure of the human lipid-gated cation channel TRPC3. Elife 7:e36852. doi: 10.7554/eLife.36852
Fernández-Peña, C., and Viana, F. (2013). Targeting TRPM8 for pain relief. Open Pain J. 6, 154–164. doi: 10.2174/1876386301306010154
Fine, M., Schmiege, P., and Li, X. (2018). Structural basis for PtdInsP2-mediated human TRPML1 regulation. Nat. Commun. 9:4192. doi: 10.1038/s41467-018-06493-7
Gan, N., Han, Y., Zeng, W., Wang, Y., Xue, J., and Jiang, Y. (2022). Structural mechanism of allosteric activation of TRPML1 by PI(3,5)P2 and rapamycin. Proc. Natl. Acad. Sci. U.S.A. 119:e2120404119. doi: 10.1073/pnas.2120404119
Gao, L., Yang, P., Qin, P., Lu, Y., Li, X., Tian, Q., et al. (2016). Selective potentiation of 2-APB-induced activation of TRPV1-3 channels by acid. Sci. Rep. 6:20791. doi: 10.1038/srep20791
Gao, Y., Cao, E., Julius, D., and Cheng, Y. (2016). TRPV1 structures in nanodiscs reveal mechanisms of ligand and lipid action. Nature 534, 347–351. doi: 10.1038/nature17964
Gees, M., Owsianik, G., Nilius, B., and Voets, T. (2012). “TRP channels,” in Comprehensive physiology, ed. R. Terjung (Hoboken, NJ: John Wiley & Sons, Ltd), 563–608. doi: 10.1002/cphy.c110026
Gochman, A., Tan, X., Bae, C., Chen, H., Swartz, K. J., and Jara-Oseguera, A. (2023). Cannabidiol sensitizes TRPV2 channels to activation by 2-APB. bioRxiv [preprint]. doi: 10.1101/2023.01.27.525817
Goldenberg, N. M., Wang, L., Ranke, H., Liedtke, W., Tabuchi, A., and Kuebler, W. M. (2015b). TRPV4 is required for hypoxic pulmonary vasoconstriction. Anesthesiology 122, 1338–1348. doi: 10.1097/ALN.0000000000000647
Goldenberg, N. M., Ravindran, K., and Kuebler, W. M. (2015a). TRPV4: physiological role and therapeutic potential in respiratory diseases. Naunyn Schmiedebergs Arch. Pharmacol. 388, 421–436. doi: 10.1007/s00210-014-1058-1
González-Ramírez, R., Chen, Y., Liedtke, W. B., and Morales-Lázaro, S. L. (2017). “TRP channels and pain,” in Neurobiology of TRP channels, ed. T. L. R. Emir (Boca Raton, FL: CRC Press).
Grace, M. S., Baxter, M., Dubuis, E., Birrell, M. A., and Belvisi, M. G. (2014). Transient receptor potential (TRP) channels in the airway: role in airway disease. Br. J. Pharmacol. 171, 2593–2607. doi: 10.1111/bph.12538
Grandl, J., Hu, H., Bandell, M., Bursulaya, B., Schmidt, M., Petrus, M., et al. (2008). Pore region of TRPV3 ion channel is specifically required for heat-activation. Nat. Neurosci. 11, 1007–1013.
Grandl, J., Kim, S. E., Uzzell, V., Bursulaya, B., Petrus, M., Bandell, M., et al. (2010). Temperature-induced opening of TRPV1 ion channel is stabilized by the pore domain. Nat. Neurosci. 13, 708–714. doi: 10.1038/nn.2552
Gualandi, F., Zaraket, F., Malagù, M., Parmeggiani, G., Trabanelli, C., Fini, S., et al. (2017). Mutation load of multiple ion channel gene mutations in Brugada syndrome. Cardiology 137, 256–260. doi: 10.1159/000471792
Guo, J., She, J., Zeng, W., Chen, Q., Bai, X.-C., and Jiang, Y. (2017). Structures of the calcium-activated, non-selective cation channel TRPM4. Nature 552, 205–209. doi: 10.1038/nature24997
Guo, W., Tang, Q., Wei, M., Kang, Y., Wu, J.-X., and Chen, L. (2022). Structural mechanism of human TRPC3 and TRPC6 channel regulation by their intracellular calcium-binding sites. Neuron 110, 1023–1035.e5. doi: 10.1016/j.neuron.2021.12.023
He, L.-H., Liu, M., He, Y., Xiao, E., Zhao, L., Zhang, T., et al. (2017). TRPV1 deletion impaired fracture healing and inhibited osteoclast and osteoblast differentiation. Sci. Rep. 7:42385. doi: 10.1038/srep42385
Hebert, S. C. (1998). General principles of the structure of ion channels. Am. J. Med. 104, 87–98. doi: 10.1016/s0002-9343(97)00358-6
Hermosura, M. C., Cui, A. M., Go, R. C. V., Davenport, B., Shetler, C. M., Heizer, J. W., et al. (2008). Altered functional properties of a TRPM2 variant in Guamanian ALS and PD. Proc. Natl. Acad. Sci. U.S.A. 105, 18029–18034. doi: 10.1073/pnas.0808218105
Hermosura, M. C., Nayakanti, H., Dorovkov, M. V., Calderon, F. R., Ryazanov, A. G., Haymer, D. S., et al. (2005). A TRPM7 variant shows altered sensitivity to magnesium that may contribute to the pathogenesis of two Guamanian neurodegenerative disorders. Proc. Natl. Acad. Sci. U.S.A. 102, 11510–11515. doi: 10.1073/pnas.0505149102
Hinman, A., Chuang, H.-H., Bautista, D. M., and Julius, D. (2006). TRP channel activation by reversible covalent modification. Proc. Natl. Acad. Sci. U.S.A. 103, 19564–19568. doi: 10.1073/pnas.0609598103
Hof, T., Liu, H., Sallé, L., Schott, J.-J., Ducreux, C., Millat, G., et al. (2017). TRPM4 non-selective cation channel variants in long QT syndrome. BMC Med. Genet. 18:31. doi: 10.1186/s12881-017-0397-4
Hori, S., Tateyama, M., Shirai, T., Kubo, Y., and Saitoh, O. (2023). Two single-point mutations in Ankyrin Repeat one drastically change the threshold temperature of TRPV1. Nat. Commun. 14:2415. doi: 10.1038/s41467-023-38051-1
Howard, J., and Bechstedt, S. (2004). Hypothesis: a helix of ankyrin repeats of the NOMPC-TRP ion channel is the gating spring of mechanoreceptors. Curr. Biol. 14, R224–R226. doi: 10.1016/j.cub.2004.02.050
Hsu, Y.-J., Hoenderop, J. G. J., and Bindels, R. J. M. (2007). TRP channels in kidney disease. Biochim. Biophys. Acta Mol. Basis Dis. 1772, 928–936. doi: 10.1016/j.bbadis.2007.02.001
Huang, J., and Chen, J. (2023). Hydrophobic gating in bundle-crossing ion channels: A case study of TRPV4. Commun. Biol. 6, 1–9. doi: 10.1038/s42003-023-05471-0
Huang, Y., Fliegert, R., Guse, A. H., Lü, W., and Du, J. (2020). A structural overview of the ion channels of the TRPM family. Cell Calcium 85:102111. doi: 10.1016/j.ceca.2019.102111
Huang, Y., Roth, B., Lü, W., and Du, J. (2019). Ligand recognition and gating mechanism through three ligand-binding sites of human TRPM2 channel. Elife 8:e50175. doi: 10.7554/eLife.50175
Hübner, C. A., and Jentsch, T. J. (2002). Ion channel diseases. Hum. Mol. Genet. 11, 2435–2445. doi: 10.1093/hmg/11.20.2435
Huffer, K. E., Aleksandrova, A. A., Jara-Oseguera, A., Forrest, L. R., and Swartz, K. J. (2020). Global alignment and assessment of TRP channel transmembrane domain structures to explore functional mechanisms. ELife 9:e58660. doi: 10.7554/eLife.58660
Hughes, T. E. T., Lodowski, D. T., Huynh, K. W., Yazici, A., Del Rosario, J., Kapoor, A., et al. (2018). Structural basis of TRPV5 channel inhibition by econazole revealed by cryo-EM. Nat. Struct. Mol. Biol. 25, 53–60. doi: 10.1038/s41594-017-0009-1
Hughes, T. E., Del Rosario, J. S., Kapoor, A., Yazici, A. T., Yudin, Y., Fluck, E. C., et al. (2019). Structure-based characterization of novel TRPV5 inhibitors. Elife 8:e49572. doi: 10.7554/eLife.49572
Hutchings, C. J., Colussi, P., and Clark, T. G. (2019). Ion channels as therapeutic antibody targets. MAbs 11, 265–296. doi: 10.1080/19420862.2018.1548232
Iftinca, M., Defaye, M., and Altier, C. (2021). TRPV1-targeted drugs in development for human pain conditions. Drugs 81, 7–27. doi: 10.1007/s40265-020-01429-2
Inada, H., Kawabata, F., Ishimaru, Y., Fushiki, T., Matsunami, H., and Tominaga, M. (2008). Off-response property of an acid-activated cation channel complex PKD1L3-PKD2L1. EMBO Rep. 9, 690–697. doi: 10.1038/embor.2008.89
Janin, A., Bessière, F., Georgescu, T., Chanavat, V., Chevalier, P., and Millat, G. (2019). TRPM4 mutations to cause autosomal recessive and not autosomal dominant Brugada type 1 syndrome. Eur. J. Med. Genet. 62:103527. doi: 10.1016/j.ejmg.2018.08.008
Jara-Oseguera, A., Huffer, K. E., and Swartz, K. J. (2019). The ion selectivity filter is not an activation gate in TRPV1-3 channels. Elife 8:e51212. doi: 10.7554/eLife.51212
Jiang, J., Li, M., and Yue, L. (2005). Potentiation of TRPM7 inward currents by protons. J. Gen. Physiol. 126, 137–150. doi: 10.1085/jgp.200409185
Jimenez, I., Prado, Y., Marchant, F., Otero, C., Eltit, F., Cabello-Verrugio, C., et al. (2020). TRPM channels in human diseases. Cells 9:2604. doi: 10.3390/cells9122604
Jordt, S.-E., Tominaga, M., and Julius, D. (2000). Acid potentiation of the capsaicin receptor determined by a key extracellular site. Proc. Natl. Acad. Sci. U.S.A. 97, 8134–8139. doi: 10.1073/pnas.100129497
Joseph, J., Wang, S., Lee, J., Ro, J. Y., and Chung, M.-K. (2013). Carboxyl-terminal domain of transient receptor potential Vanilloid 1 contains distinct segments differentially involved in capsaicin- and heat-induced desensitization. J. Biol. Chem. 288, 35690–35702. doi: 10.1074/jbc.M113.513374
Just, S., Chenard, B. L., Ceci, A., Strassmaier, T., Chong, J. A., Blair, N. T., et al. (2018). Treatment with HC-070, a potent inhibitor of TRPC4 and TRPC5, leads to anxiolytic and antidepressant effects in mice. PLoS One 13:e0191225. doi: 10.1371/journal.pone.0191225
Kaczorowski, G. J., McManus, O. B., Priest, B. T., and Garcia, M. L. (2008). Ion channels as drug targets: the next GPCRs. J. Gen. Physiol. 131, 399–405. doi: 10.1085/jgp.200709946
Karashima, Y., Damann, N., Prenen, J., Talavera, K., Segal, A., Voets, T., et al. (2007). Bimodal action of menthol on the transient receptor potential channel TRPA1. J. Neurosci. 27, 9874–9884. doi: 10.1523/JNEUROSCI.2221-07.2007
Kashio, M., and Tominaga, M. (2022). TRP channels in thermosensation. Curr. Opin. Neurobiol. 75:102591. doi: 10.1016/j.conb.2022.102591
Katsura, H., Obata, K., Mizushima, T., Yamanaka, H., Kobayashi, K., Dai, Y., et al. (2006). Antisense knock down of TRPA1, but not TRPM8, alleviates cold hyperalgesia after spinal nerve ligation in rats. Exp. Neurol. 200, 112–123. doi: 10.1016/j.expneurol.2006.01.031
Kerstein, P. C., del Camino, D., Moran, M. M., and Stucky, C. L. (2009). Pharmacological blockade of TRPA1 inhibits mechanical firing in nociceptors. Mol. Pain 5:19. doi: 10.1186/1744-8069-5-19
Keynes, R. D. (1975). The ionic channels in excitable membranes. Ciba Found. Symp. 31, 191–203. doi: 10.1002/9780470720134.ch11
Khaleel, A., Wu, M.-S., Wong, H. S.-C., Hsu, Y.-W., Chou, Y.-H., and Chen, H.-Y. (2015). A single nucleotide polymorphism (rs4236480) in TRPV5 calcium channel gene is associated with stone multiplicity in calcium nephrolithiasis patients. Mediators Inflamm. 2015:375427. doi: 10.1155/2015/375427
Khalid, S., Murdoch, R., Newlands, A., Smart, K., Kelsall, A., Holt, K., et al. (2014). Transient receptor potential vanilloid 1 (TRPV1) antagonism in patients with refractory chronic cough: a double-blind randomized controlled trial. J. Allergy Clin. Immunol. 134, 56–62. doi: 10.1016/j.jaci.2014.01.038
Khattar, V., Wang, L., and Peng, J.-B. (2022). Calcium selective channel TRPV6: structure, function, and implications in health and disease. Gene 817:146192. doi: 10.1016/j.gene.2022.146192
Kim, M. J., Jeon, J.-P., Kim, H. J., Kim, B. J., Lee, Y. M., Choe, H., et al. (2008). Molecular determinant of sensing extracellular pH in classical transient receptor potential channel 5. Biochem. Biophys. Res. Commun. 365, 239–245. doi: 10.1016/j.bbrc.2007.10.154
Kim, M., Sisco, N. J., Hilton, J. K., Montano, C. M., Castro, M. A., Cherry, B. R., et al. (2020). Evidence that the TRPV1 S1-S4 membrane domain contributes to thermosensing. Nat. Commun. 11:4169. doi: 10.1038/s41467-020-18026-2
Koivisto, A., Hukkanen, M., Saarnilehto, M., Chapman, H., Kuokkanen, K., Wei, H., et al. (2012). Inhibiting TRPA1 ion channel reduces loss of cutaneous nerve fiber function in diabetic animals: sustained activation of the TRPA1 channel contributes to the pathogenesis of peripheral diabetic neuropathy. Pharmacol. Res. 65, 149–158. doi: 10.1016/j.phrs.2011.10.006
Koivisto, A.-P., Belvisi, M. G., Gaudet, R., and Szallasi, A. (2022). Advances in TRP channel drug discovery: from target validation to clinical studies. Nat. Rev. Drug Discov. 21, 41–59. doi: 10.1038/s41573-021-00268-4
Kremeyer, B., Lopera, F., Cox, J. J., Momin, A., Rugiero, F., Marsh, S., et al. (2010). A gain-of-function mutation in TRPA1 causes familial episodic pain syndrome. Neuron 66, 671–680. doi: 10.1016/j.neuron.2010.04.030
Kruse, M., Schulze-Bahr, E., Corfield, V., Beckmann, A., Stallmeyer, B., Kurtbay, G., et al. (2009). Impaired endocytosis of the ion channel TRPM4 is associated with human progressive familial heart block type I. J. Clin. Invest. 119, 2737–2744. doi: 10.1172/JCI38292
Kuebler, W. M., Jordt, S.-E., and Liedtke, W. B. (2020). Urgent reconsideration of lung edema as a preventable outcome in COVID-19: inhibition of TRPV4 represents a promising and feasible approach. Am. J. Physiol. Lung Cell. Mol. Physiol. 318, L1239–L1243. doi: 10.1152/ajplung.00161.2020
Kwan, K. Y., Glazer, J. M., Corey, D. P., Rice, F. L., and Stucky, C. L. (2009). TRPA1 modulates mechanotransduction in cutaneous sensory neurons. J. Neurosci. 29, 4808–4819. doi: 10.1523/JNEUROSCI.5380-08.2009
Kwon, D. H., Zhang, F., Fedor, J. G., Suo, Y., and Lee, S.-Y. (2022). Vanilloid-dependent TRPV1 opening trajectory from cryoEM ensemble analysis. Nat. Commun. 13:2874. doi: 10.1038/s41467-022-30602-2
Kwon, D. H., Zhang, F., McCray, B. A., Feng, S., Kumar, M., Sullivan, J. M., et al. (2023). TRPV4-Rho GTPase complex structures reveal mechanisms of gating and disease. Nat. Commun. 14:3732. doi: 10.1038/s41467-023-39345-0
Kwon, D. H., Zhang, F., Suo, Y., Bouvette, J., Borgnia, M. J., and Lee, S.-Y. (2021). Heat-dependent opening of TRPV1 in the presence of capsaicin. Nat. Struct. Mol. Biol. 28, 554–563. doi: 10.1038/s41594-021-00616-3
Ladrón-de-Guevara, E., Dominguez, L., Rangel-Yescas, G. E., Fernández-Velasco, D. A., Torres-Larios, A., Rosenbaum, T., et al. (2020). The contribution of the ankyrin repeat domain of TRPV1 as a thermal module. Biophys. J. 118, 836–845. doi: 10.1016/j.bpj.2019.10.041
Lee, S. P., Buber, M. T., Yang, Q., Cerne, R., Cortés, R. Y., Sprous, D. G., et al. (2008). Thymol and related alkyl phenols activate the hTRPA1 channel. Br. J. Pharmacol. 153, 1739–1749. doi: 10.1038/bjp.2008.85
Lehen’kyi, V., and Prevarskaya, N. (2011). “Oncogenic TRP channels,” in Transient receptor potential channels, ed. M. d. S. Islam (Dordrecht: Springer Netherlands), 929–945. doi: 10.1007/978-94-007-0265-3_48
Li, M., Du, J., Jiang, J., Ratzan, W., Su, L.-T., Runnels, L. W., et al. (2007). Molecular determinants of Mg2+ and Ca2+ permeability and pH sensitivity in TRPM6 and TRPM7. J. Biol. Chem. 282, 25817–25830. doi: 10.1074/jbc.M608972200
Li, Z., Sergouniotis, P. I., Michaelides, M., Mackay, D. S., Wright, G. A., Devery, S., et al. (2009). Recessive mutations of the gene TRPM1 abrogate ON bipolar cell function and cause complete congenital stationary night blindness in humans. Am. J. Hum. Genet. 85, 711–719. doi: 10.1016/j.ajhg.2009.10.003
Liao, M., Cao, E., Julius, D., and Cheng, Y. (2013). Structure of the TRPV1 ion channel determined by electron cryo-microscopy. Nature 504, 107–112. doi: 10.1038/nature12822
Lin, S.-Y., and Corey, D. P. (2005). TRP channels in mechanosensation. Curr. Opin. Neurobiol. 15, 350–357. doi: 10.1016/j.conb.2005.05.012
Lin, Z., Chen, Q., Lee, M., Cao, X., Zhang, J., Ma, D., et al. (2012). Exome sequencing reveals mutations in TRPV3 as a cause of Olmsted syndrome. Am. J. Hum. Genet. 90, 558–564. doi: 10.1016/j.ajhg.2012.02.006
Liu, B., and Qin, F. (2021). Identification of a helix-turn-helix motif for high temperature dependence of vanilloid receptor TRPV2. J. Physiol. 599, 4831–4844. doi: 10.1113/JP282073
Liu, C., Reese, R., Vu, S., Rougé, L., Shields, S. D., Kakiuchi-Kiyota, S., et al. (2021). A non-covalent ligand reveals biased agonism of the TRPA1 ion channel. Neuron 109, 273–284.e4. doi: 10.1016/j.neuron.2020.10.014
Liu, D., and Liman, E. R. (2003). Intracellular Ca2+ and the phospholipid PIP2 regulate the taste transduction ion channel TRPM5. Proc. Natl. Acad. Sci. U.S.A. 100, 15160–15165. doi: 10.1073/pnas.2334159100
Liu, D., Zhang, Z., and Liman, E. R. (2005). Extracellular acid block and acid-enhanced inactivation of the Ca2+-activated cation channel TRPM5 involve residues in the S3-S4 and S5-S6 extracellular domains. J. Biol. Chem. 280, 20691–20699. doi: 10.1074/jbc.M414072200
Liu, H., Chatel, S., Simard, C., Syam, N., Salle, L., Probst, V., et al. (2013). Molecular genetics and functional anomalies in a series of 248 Brugada cases with 11 mutations in the TRPM4 channel. PLoS One 8:e54131. doi: 10.1371/journal.pone.0054131
Liu, H., El Zein, L., Kruse, M., Guinamard, R., Beckmann, A., Bozio, A., et al. (2010). Gain-of-function mutations in TRPM4 cause autosomal dominant isolated cardiac conduction disease. Circ. Cardiovasc. Genet. 3, 374–385. doi: 10.1161/CIRCGENETICS.109.930867
Luu, D. D., Owens, A. M., Mebrat, M. D., and Van Horn, W. D. (2023). A molecular perspective on identifying TRPV1 thermosensitive regions and disentangling polymodal activation. Temperature 10, 67–101. doi: 10.1080/23328940.2021.1983354
Macpherson, L. J., Dubin, A. E., Evans, M. J., Marr, F., Schultz, P. G., Cravatt, B. F., et al. (2007). Noxious compounds activate TRPA1 ion channels through covalent modification of cysteines. Nature 445, 541–545. doi: 10.1038/nature05544
Manolache, A., Babes, A., and Madalina Babes, R. (2021). Mini-review: the nociceptive sensory functions of the polymodal receptor Transient Receptor Potential Ankyrin Type 1 (TRPA1). Neurosci. Lett. 764:136286. doi: 10.1016/j.neulet.2021.136286
Masamune, A., Kotani, H., Sörgel, F. L., Chen, J.-M., Hamada, S., Sakaguchi, R., et al. (2020). Variants that affect function of calcium channel TRPV6 are associated with early-onset chronic pancreatitis. Gastroenterology 158, 1626–1641.e8. doi: 10.1053/j.gastro.2020.01.005
Mason, A. E., Grier, D., Smithson, S. F., Burren, C. P., and Gradhand, E. (2020). Post-mortem histology in transient receptor potential cation channel subfamily V member 6 (TRPV6) under-mineralising skeletal dysplasia suggests postnatal skeletal recovery: a case report. BMC Med. Genet. 21:64. doi: 10.1186/s12881-020-01007-z
McGoldrick, L. L., Singh, A. K., Saotome, K., Yelshanskaya, M. V., Twomey, E. C., Grassucci, R. A., et al. (2018). Opening of the human epithelial calcium channel TRPV6. Nature 553, 233–237. doi: 10.1038/nature25182
McKemy, D. D., Neuhausser, W. M., and Julius, D. (2002). Identification of a cold receptor reveals a general role for TRP channels in thermosensation. Nature 416, 52–58. doi: 10.1038/nature719
Meents, J. E., Ciotu, C. I., and Fischer, M. J. M. (2019). TRPA1: a molecular view. J. Neurophysiol. 121, 427–443. doi: 10.1152/jn.00524.2018
Mickle, A. D., Shepherd, A. J., and Mohapatra, D. P. (2015). Sensory TRP channels: the key transducers of nociception and pain. Prog. Mol. Biol. Transl. Sci. 131, 73–118. doi: 10.1016/bs.pmbts.2015.01.002
Minor, D. L. (2010). “Chapter 30–An overview of ion channel structure,” in Handbook of cell signaling, Second Edn, eds R. A. Bradshaw and E. A. Dennis (San Diego, CA: Academic Press), 201–207. doi: 10.1016/B978-0-12-374145-5.00030-9
Mochizuki, T., Wu, G., Hayashi, T., Xenophontos, S. L., Veldhuisen, B., Saris, J. J., et al. (1996). PKD2, a gene for polycystic kidney disease that encodes an integral membrane protein. Science 272, 1339–1342. doi: 10.1126/science.272.5266.1339
Montell, C. (2005). The TRP superfamily of cation channels. Sci. STKE 2005:re3. doi: 10.1126/stke.2722005re3
Montell, C., Birnbaumer, L., Flockerzi, V., Bindels, R. J., Bruford, E. A., Caterina, M. J., et al. (2002). A unified nomenclature for the superfamily of TRP cation channels. Mol. Cell 9, 229–231. doi: 10.1016/s1097-2765(02)00448-3
Moore, C., Gupta, R., Jordt, S.-E., Chen, Y., and Liedtke, W. B. (2018). Regulation of pain and itch by TRP channels. Neurosci. Bull. 34, 120–142. doi: 10.1007/s12264-017-0200-8
Moran, M. M., and Szallasi, A. (2018). Targeting nociceptive transient receptor potential channels to treat chronic pain: current state of the field. Br. J. Pharmacol. 175, 2185–2203. doi: 10.1111/bph.14044
Moran, M. M., McAlexander, M. A., Bíró, T., and Szallasi, A. (2011). Transient receptor potential channels as therapeutic targets. Nat. Rev. Drug Discov. 10, 601–620. doi: 10.1038/nrd3456
Mukhopadhyay, I., Kulkarni, A., Aranake, S., Karnik, P., Shetty, M., Thorat, S., et al. (2014). Transient receptor potential ankyrin 1 receptor activation in vitro and in vivo by pro-tussive agents: GRC 17536 as a promising anti-tussive therapeutic. PLoS One 9:e97005. doi: 10.1371/journal.pone.0097005
Myers, B. R., Bohlen, C. J., and Julius, D. (2008). A yeast genetic screen reveals a critical role for the pore helix domain in TRP channel gating. Neuron 58, 362–373. doi: 10.1016/j.neuron.2008.04.012
Nadezhdin, K. D., Talyzina, I. A., Parthasarathy, A., Neuberger, A., Zhang, D. X., and Sobolevsky, A. I. (2023b). Structure of human TRPV4 in complex with GTPase RhoA. Nat. Commun. 14:3733. doi: 10.1038/s41467-023-39346-z
Nadezhdin, K. D., Correia, L., Narangoda, C., Patel, D. S., Neuberger, A., Gudermann, T., et al. (2023a). Structural mechanisms of TRPM7 activation and inhibition. Nat. Commun. 14:2639. doi: 10.1038/s41467-023-38362-3
Nadezhdin, K. D., Neuberger, A., Nikolaev, Y. A., Murphy, L. A., Gracheva, E. O., Bagriantsev, S. N., et al. (2021a). Extracellular cap domain is an essential component of the TRPV1 gating mechanism. Nat. Commun. 12:2154. doi: 10.1038/s41467-021-22507-3
Nadezhdin, K. D., Neuberger, A., Trofimov, Y. A., Krylov, N. A., Sinica, V., Kupko, N., et al. (2021b). Structural mechanism of heat-induced opening of a temperature-sensitive TRP channel. Nat. Struct. Mol. Biol. 28, 564–572. doi: 10.1038/s41594-021-00615-4
Nagata, K., Zheng, L., Madathany, T., Castiglioni, A. J., Bartles, J. R., and García-Añoveros, J. (2008). The varitint-waddler (Va) deafness mutation in TRPML3 generates constitutive, inward rectifying currents and causes cell degeneration. Proc. Natl. Acad. Sci. U.S.A. 105, 353–358. doi: 10.1073/pnas.0707963105
Neuberger, A., Nadezhdin, K. D., and Sobolevsky, A. I. (2021). Structural mechanisms of TRPV6 inhibition by ruthenium red and econazole. Nat. Commun. 12:6284. doi: 10.1038/s41467-021-26608-x
Neuberger, A., Nadezhdin, K. D., and Sobolevsky, A. I. (2022). Structural mechanism of TRPV3 channel inhibition by the anesthetic dyclonine. Nat. Commun. 13:2795. doi: 10.1038/s41467-022-30537-8
Neuberger, A., Oda, M., Nikolaev, Y. A., Nadezhdin, K. D., Gracheva, E. O., Bagriantsev, S. N., et al. (2023). Human TRPV1 structure and inhibition by the analgesic SB-366791. Nat. Commun. 14:2451. doi: 10.1038/s41467-023-38162-9
Ni, C., Yan, M., Zhang, J., Cheng, R., Liang, J., Deng, D., et al. (2016). A novel mutation in TRPV3 gene causes atypical familial Olmsted syndrome. Sci. Rep. 6:21815. doi: 10.1038/srep21815
Nilius, B., and Owsianik, G. (2010). Channelopathies converge on TRPV4. Nat. Genet. 42, 98–100. doi: 10.1038/ng0210-98
Nilius, B., and Owsianik, G. (2011). The transient receptor potential family of ion channels. Genome Biol. 12:218. doi: 10.1186/gb-2011-12-3-218
Nilius, B., and Voets, T. (2013). The puzzle of TRPV4 channelopathies. EMBO Rep. 14, 152–163. doi: 10.1038/embor.2012.219
Nilius, B., Mahieu, F., Prenen, J., Janssens, A., Owsianik, G., Vennekens, R., et al. (2006). The Ca2+-activated cation channel TRPM4 is regulated by phosphatidylinositol 4,5-biphosphate. EMBO J. 25, 467–478. doi: 10.1038/sj.emboj.7600963
Nishimura, G., Lausch, E., Savarirayan, R., Shiba, M., Spranger, J., Zabel, B., et al. (2012). TRPV4-associated skeletal dysplasias. Am. J. Med. Genet. Part C Semin. Med. Genet. 160C, 190–204. doi: 10.1002/ajmg.c.31335
Noto, C., Pappagallo, M., and Szallasi, A. (2009). NGX-4010, a high-concentration capsaicin dermal patch for lasting relief of peripheral neuropathic pain. Curr. Opin. Investig. Drugs 10, 702–710.
Numata, T., and Okada, Y. (2008). Proton conductivity through the human TRPM7 channel and its molecular determinants. J. Biol. Chem. 283, 15097–15103. doi: 10.1074/jbc.M709261200
O’Neil, R. G., and Heller, S. (2005). The mechanosensitive nature of TRPV channels. Pflugers Arch. Eur. J. Physiol. 451, 193–203. doi: 10.1007/s00424-005-1424-4
Obata, K., Katsura, H., Mizushima, T., Yamanaka, H., Kobayashi, K., Dai, Y., et al. (2005). TRPA1 induced in sensory neurons contributes to cold hyperalgesia after inflammation and nerve injury. J. Clin. Invest. 115, 2393–2401. doi: 10.1172/JCI25437
Oddsson, A., Sulem, P., Helgason, H., Edvardsson, V. O., Thorleifsson, G., Sveinbjörnsson, G., et al. (2015). Common and rare variants associated with kidney stones and biochemical traits. Nat. Commun. 6:7975. doi: 10.1038/ncomms8975
Ogawa, N., Kurokawa, T., and Mori, Y. (2016). Sensing of redox status by TRP channels. Cell Calcium 60, 115–122. doi: 10.1016/j.ceca.2016.02.009
Okada, T., Inoue, R., Yamazaki, K., Maeda, A., Kurosaki, T., Yamakuni, T., et al. (1999). Molecular and functional characterization of a novel mouse transient receptor potential protein homologue TRP7. Ca(2+)-permeable cation channel that is constitutively activated and enhanced by stimulation of G protein-coupled receptor. J. Biol. Chem. 274, 27359–27370. doi: 10.1074/jbc.274.39.27359
Patel, T., Ishiuji, Y., and Yosipovitch, G. (2007). Menthol: a refreshing look at this ancient compound. J. Am. Acad. Dermatol. 57, 873–878. doi: 10.1016/j.jaad.2007.04.008
Paulsen, C. E., Armache, J.-P., Gao, Y., Cheng, Y., and Julius, D. (2015). Structure of the TRPA1 ion channel suggests regulatory mechanisms. Nature 520, 511–517. doi: 10.1038/nature14367
Peier, A. M., Moqrich, A., Hergarden, A. C., Reeve, A. J., Andersson, D. A., Story, G. M., et al. (2002). A TRP channel that senses cold stimuli and menthol. Cell 108, 705–715. doi: 10.1016/s0092-8674(02)00652-9
Pumroy, R. A., Fluck, E. C., Ahmed, T., and Moiseenkova-Bell, V. Y. (2020). Structural insights into the gating mechanisms of TRPV channels. Cell Calcium 87:102168. doi: 10.1016/j.ceca.2020.102168
Pumroy, R. A., Samanta, A., Liu, Y., Hughes, T. E., Zhao, S., Yudin, Y., et al. (2019). Molecular mechanism of TRPV2 channel modulation by cannabidiol. ELife 8:e48792. doi: 10.7554/eLife.48792
Raemdonck, K., de Alba, J., Birrell, M. A., Grace, M., Maher, S. A., Irvin, C. G., et al. (2012). A role for sensory nerves in the late asthmatic response. Thorax 67, 19–25. doi: 10.1136/thoraxjnl-2011-200365
Romanovsky, A. A., Almeida, M. C., Garami, A., Steiner, A. A., Norman, M. H., Morrison, S. F., et al. (2009). The transient receptor potential vanilloid-1 channel in thermoregulation: a thermosensor it is not. Pharmacol. Rev. 61, 228–261. doi: 10.1124/pr.109.001263
Rosenbaum, T., Benitez-Angeles, M., Sanchez-Hernandez, R., Morales-Lazaro, S. L., Hiriart, M., Morales-Buenrostro, L. E., et al. (2020). TRPV4: a physio and pathophysiologically significant ion channel. Int. J. Mol. Sci. 21:3837. doi: 10.3390/ijms21113837
Ruan, Z., Haley, E., Orozco, I. J., Sabat, M., Myers, R., Roth, R., et al. (2021). Structures of the TRPM5 channel elucidate mechanisms of activation and inhibition. Nat. Struct. Mol. Biol. 28, 604–613. doi: 10.1038/s41594-021-00607-4
Runnels, L. W., Yue, L., and Clapham, D. E. (2002). The TRPM7 channel is inactivated by PIP(2) hydrolysis. Nat. Cell Biol. 4, 329–336. doi: 10.1038/ncb781
Saito, S., Ohkita, M., Saito, C. T., Takahashi, K., Tominaga, M., and Ohta, T. (2016). Evolution of heat sensors drove shifts in thermosensation between Xenopus species adapted to different thermal niches. J. Biol. Chem. 291, 11446–11459. doi: 10.1074/jbc.M115.702498
Samanta, A., Hughes, T. E. T., and Moiseenkova-Bell, V. Y. (2018). Transient receptor potential (TRP) channels. Subcell. Biochem. 87, 141–165. doi: 10.1007/978-981-10-7757-9_6
Santoni, G., and Farfariello, V. (2011). TRP channels and cancer: new targets for diagnosis and chemotherapy. Endocr. Metab. Immune Disord. Drug Targets 11, 54–67. doi: 10.2174/187153011794982068
Santos, R., Ursu, O., Gaulton, A., Bento, A. P., Donadi, R. S., Bologa, C. G., et al. (2017). A comprehensive map of molecular drug targets. Nat. Rev. Drug Discov. 16, 19–34. doi: 10.1038/nrd.2016.230
Schmiege, P., Fine, M., and Li, X. (2021). Atomic insights into ML-SI3 mediated human TRPML1 inhibition. Structure 29, 1295–1302.e3. doi: 10.1016/j.str.2021.06.003
Semtner, M., Schaefer, M., Pinkenburg, O., and Plant, T. D. (2007). Potentiation of TRPC5 by protons. J. Biol. Chem. 282, 33868–33878. doi: 10.1074/jbc.M702577200
Shapovalov, G., Ritaine, A., Skryma, R., and Prevarskaya, N. (2016). Role of TRP ion channels in cancer and tumorigenesis. Semin. Immunopathol. 38, 357–369. doi: 10.1007/s00281-015-0525-1
Singh, A. K., McGoldrick, L. L., and Sobolevsky, A. I. (2018). Structure and gating mechanism of the transient receptor potential channel TRPV3. Nat. Struct. Mol. Biol. 25, 805–813. doi: 10.1038/s41594-018-0108-7
Singh, A. K., McGoldrick, L. L., Demirkhanyan, L., Leslie, M., Zakharian, E., and Sobolevsky, A. I. (2019). Structural basis of temperature sensation by the TRP channel TRPV3. Nat. Struct. Mol. Biol. 26, 994–998. doi: 10.1038/s41594-019-0318-7
Song, K., Wei, M., Guo, W., Quan, L., Kang, Y., Wu, J.-X., et al. (2021). Structural basis for human TRPC5 channel inhibition by two distinct inhibitors. Elife 10:e63429. doi: 10.7554/eLife.63429
Souza Monteiro de Araujo, D., Nassini, R., Geppetti, P., and De Logu, F. (2020). TRPA1 as a therapeutic target for nociceptive pain. Expert Opin. Ther. Targets 24, 997–1008. doi: 10.1080/14728222.2020.1815191
Staaf, S., Oerther, S., Lucas, G., Mattsson, J. P., and Ernfors, P. (2009). Differential regulation of TRP channels in a rat model of neuropathic pain. Pain 144, 187–199. doi: 10.1016/j.pain.2009.04.013
Staff, N. P., Grisold, A., Grisold, W., and Windebank, A. J. (2017). Chemotherapy-induced peripheral neuropathy: a current review. Ann. Neurol. 81, 772–781. doi: 10.1002/ana.24951
Su, N., Zhen, W., Zhang, H., Xu, L., Jin, Y., Chen, X., et al. (2023). Structural mechanisms of TRPV2 modulation by endogenous and exogenous ligands. Nat. Chem. Biol. 19, 72–80. doi: 10.1038/s41589-022-01139-8
Sun, M., Goldin, E., Stahl, S., Falardeau, J. L., Kennedy, J. C., Acierno, J. S., et al. (2000). Mucolipidosis type IV is caused by mutations in a gene encoding a novel transient receptor potential channel. Hum. Mol. Genet. 9, 2471–2478. doi: 10.1093/hmg/9.17.2471
Sun, S., and Dong, X. (2016). Trp channels and itch. Semin. Immunopathol. 38, 293–307. doi: 10.1007/s00281-015-0530-4
Suo, Y., Wang, Z., Zubcevic, L., Hsu, A. L., He, Q., Borgnia, M. J., et al. (2020). Structural insights into electrophile irritant sensing by the human TRPA1 channel. Neuron 105, 882–894.e5. doi: 10.1016/j.neuron.2019.11.023
Suzuki, M., Mizuno, A., Kodaira, K., and Imai, M. (2003). Impaired pressure sensation in mice lacking TRPV4. J. Biol. Chem. 278, 22664–22668. doi: 10.1074/jbc.M302561200
Suzuki, Y., Chitayat, D., Sawada, H., Deardorff, M. A., McLaughlin, H. M., Begtrup, A., et al. (2018). TRPV6 variants interfere with maternal-fetal calcium transport through the placenta and cause transient neonatal hyperparathyroidism. Am. J. Hum. Genet. 102, 1104–1114. doi: 10.1016/j.ajhg.2018.04.006
Suzuki, Y., Pasch, A., Bonny, O., Mohaupt, M. G., Hediger, M. A., and Frey, F. J. (2008). Gain-of-function haplotype in the epithelial calcium channel TRPV6 is a risk factor for renal calcium stone formation. Hum. Mol. Genet. 17, 1613–1618. doi: 10.1093/hmg/ddn048
Suzuki, Y., Sawada, H., Tokumasu, T., Suzuki, S., Ninomiya, S., Shirai, M., et al. (2020). Novel TRPV6 mutations in the spectrum of transient neonatal hyperparathyroidism. J. Physiol. Sci. 70:33. doi: 10.1186/s12576-020-00761-2
Syam, N., Chatel, S., Ozhathil, L. C., Sottas, V., Rougier, J.-S., Baruteau, A., et al. (2016). Variants of transient receptor potential melastatin member 4 in childhood atrioventricular block. J. Am. Heart Assoc. 5:e001625. doi: 10.1161/JAHA.114.001625
Szolcsányi, J. (2015). Effect of capsaicin on thermoregulation: an update with new aspects. Temperature 2, 277–296. doi: 10.1080/23328940.2015.1048928
Takahashi, N., and Mori, Y. (2011). TRP channels as sensors and signal integrators of redox status changes. Front. Pharmacol. 2:58. doi: 10.3389/fphar.2011.00058
Takahashi, N., Mizuno, Y., Kozai, D., Yamamoto, S., Kiyonaka, S., Shibata, T., et al. (2008). Molecular characterization of TRPA1 channel activation by cysteine-reactive inflammatory mediators. Channels (Austin) 2, 287–298. doi: 10.4161/chan.2.4.6745
Talavera, K., Yasumatsu, K., Voets, T., Droogmans, G., Shigemura, N., Ninomiya, Y., et al. (2005). Heat activation of TRPM5 underlies thermal sensitivity of sweet taste. Nature 438, 1022–1025. doi: 10.1038/nature04248
Terrett, J. A., Chen, H., Shore, D. G., Villemure, E., Larouche-Gauthier, R., Déry, M., et al. (2021). Tetrahydrofuran-based transient receptor potential ankyrin 1 (TRPA1) antagonists: ligand-based discovery, activity in a rodent asthma model, and mechanism-of-action via cryogenic electron microscopy. J. Med. Chem. 64, 3843–3869. doi: 10.1021/acs.jmedchem.0c02023
Tominaga, M., Caterina, M. J., Malmberg, A. B., Rosen, T. A., Gilbert, H., Skinner, K., et al. (1998). The cloned capsaicin receptor integrates multiple pain-producing stimuli. Neuron 21, 531–543. doi: 10.1016/s0896-6273(00)80564-4
Tóth, B. I., Szallasi, A., and Bíró, T. (2015). Transient receptor potential channels and itch: how deep should we scratch? Handb. Exp. Pharmacol. 226, 89–133. doi: 10.1007/978-3-662-44605-8_6
van Abel, M., Hoenderop, J. G. J., and Bindels, R. J. M. (2005). The epithelial calcium channels TRPV5 and TRPV6: regulation and implications for disease. Naunyn Schmiedebergs Arch. Pharmacol. 371, 295–306. doi: 10.1007/s00210-005-1021-2
van Genderen, M. M., Bijveld, M. M. C., Claassen, Y. B., Florijn, R. J., Pearring, J. N., Meire, F. M., et al. (2009). Mutations in TRPM1 are a common cause of complete congenital stationary night blindness. Am. J. Hum. Genet. 85, 730–736. doi: 10.1016/j.ajhg.2009.10.012
van Goor, M. K. C., Hoenderop, J. G. J., and van der Wijst, J. (2017). TRP channels in calcium homeostasis: from hormonal control to structure-function relationship of TRPV5 and TRPV6. Biochim. Biophys. Acta Mol. Cell Res. 1864, 883–893. doi: 10.1016/j.bbamcr.2016.11.027
Van Hoeymissen, E., Held, K., Nogueira Freitas, A. C., Janssens, A., Voets, T., and Vriens, J. (2020). Gain of channel function and modified gating properties in TRPM3 mutants causing intellectual disability and epilepsy. Elife 9:e57190. doi: 10.7554/eLife.57190
Vetter, I., and Lewis, R. J. (2011). Natural product ligands of TRP channels. Adv. Exp. Med. Biol. 704, 41–85. doi: 10.1007/978-94-007-0265-3_3
Viana, F. (2016). TRPA1 channels: molecular sentinels of cellular stress and tissue damage. J. Physiol. 594, 4151–4169. doi: 10.1113/JP270935
Vinayagam, D., Quentin, D., Yu-Strzelczyk, J., Sitsel, O., Merino, F., Stabrin, M., et al. (2020). Structural basis of TRPC4 regulation by calmodulin and pharmacological agents. Elife 9:e60603. doi: 10.7554/eLife.60603
Vlachová, V., Teisinger, J., Sušánková, K., Lyfenko, A., Ettrich, R., and Vyklický, L. (2003). Functional role of C-terminal cytoplasmic tail of rat vanilloid receptor 1. J. Neurosci. 23, 1340–1350. doi: 10.1523/JNEUROSCI.23-04-01340.2003
Voets, T. (2014). “TRP channels and thermosensation,” in Mammalian transient receptor potential (TRP) cation channels, Vol. 223, eds B. Nilius and V. Flockerzi (Cham: Springer International Publishing), 729–741. doi: 10.1007/978-3-319-05161-1_1
Voets, T., Talavera, K., Owsianik, G., and Nilius, B. (2005). Sensing with TRP channels. Nat. Chem. Biol. 1, 85–92. doi: 10.1038/nchembio0705-85
Vriens, J., Owsianik, G., Fisslthaler, B., Suzuki, M., Janssens, A., Voets, T., et al. (2005). Modulation of the Ca2 permeable cation channel TRPV4 by cytochrome P450 epoxygenases in vascular endothelium. Circ. Res. 97, 908–915. doi: 10.1161/01.RES.0000187474.47805.30
Walder, R. Y., Landau, D., Meyer, P., Shalev, H., Tsolia, M., Borochowitz, Z., et al. (2002). Mutation of TRPM6 causes familial hypomagnesemia with secondary hypocalcemia. Nat. Genet. 31, 171–174. doi: 10.1038/ng901
Walker, V., and Vuister, G. W. (2023). Biochemistry and pathophysiology of the Transient Potential Receptor Vanilloid 6 (TRPV6) calcium channel. Adv. Clin. Chem. 113, 43–100. doi: 10.1016/bs.acc.2022.11.002
Wang, H., and Siemens, J. (2015). TRP ion channels in thermosensation, thermoregulation and metabolism. Temperature 2, 178–187. doi: 10.1080/23328940.2015.1040604
Wang, H., Cheng, X., Tian, J., Xiao, Y., Tian, T., Xu, F., et al. (2020). TRPC channels: structure, function, regulation and recent advances in small molecular probes. Pharmacol. Ther. 209:107497. doi: 10.1016/j.pharmthera.2020.107497
Wang, H., Schupp, M., Zurborg, S., and Heppenstall, P. A. (2013). Residues in the pore region of Drosophila transient receptor potential A1 dictate sensitivity to thermal stimuli. J. Physiol. 591, 185–201. doi: 10.1113/jphysiol.2012.242842
Wang, H., Xu, Z., Lee, B. H., Vu, S., Hu, L., Lee, M., et al. (2019). Gain-of-function mutations in TRPM4 activation gate cause progressive symmetric erythrokeratodermia. J. Invest. Dermatol. 139, 1089–1097. doi: 10.1016/j.jid.2018.10.044
Wang, H., Yang, P., Lu, Y., Wang, J., Jeon, J., Wang, Q., et al. (2021). Mechanisms of proton inhibition and sensitization of the cation channel TRPV3. J. Gen. Physiol. 153:e202012663. doi: 10.1085/jgp.202012663
Wang, L., Holmes, R. P., and Peng, J.-B. (2017). The L530R variation associated with recurrent kidney stones impairs the structure and function of TRPV5. Biochem. Biophys. Res. Commun. 492, 362–367. doi: 10.1016/j.bbrc.2017.08.102
Wang, Q., Corey, R. A., Hedger, G., Aryal, P., Grieben, M., Nasrallah, C., et al. (2020). Lipid interactions of a ciliary membrane TRP channel: simulation and structural studies of polycystin-2. Structure 28, 169–184.e5. doi: 10.1016/j.str.2019.11.005
Watanabe, H., Murakami, M., Ohba, T., Takahashi, Y., and Ito, H. (2008). TRP channel and cardiovascular disease. Pharmacol. Ther. 118, 337–351. doi: 10.1016/j.pharmthera.2008.03.008
Wei, H., Koivisto, A., Saarnilehto, M., Chapman, H., Kuokkanen, K., Hao, B., et al. (2011). Spinal transient receptor potential ankyrin 1 channel contributes to central pain hypersensitivity in various pathophysiological conditions in the rat. Pain 152, 582–591. doi: 10.1016/j.pain.2010.11.031
Wes, P. D., Chevesich, J., Jeromin, A., Rosenberg, C., Stetten, G., and Montell, C. (1995). TRPC1, a human homolog of a Drosophila store-operated channel. Proc. Natl. Acad. Sci. U.S.A. 92, 9652–9656. doi: 10.1073/pnas.92.21.9652
Wetsel, W. C. (2011). Sensing hot and cold with TRP channels. Int. J. Hyperthermia 27, 388–398. doi: 10.3109/02656736.2011.554337
Weyer, A. D., and Lehto, S. G. (2017). Development of TRPM8 antagonists to treat chronic pain and migraine. Pharmaceuticals (Basel) 10:37. doi: 10.3390/ph10020037
Willis, W. D. (2009). The role of TRPV1 receptors in pain evoked by noxious thermal and chemical stimuli. Exp. Brain Res. 196, 5–11. doi: 10.1007/s00221-009-1760-2
Winn, M. P., Conlon, P. J., Lynn, K. L., Farrington, M. K., Creazzo, T., Hawkins, A. F., et al. (2005). A mutation in the TRPC6 cation channel causes familial focal segmental glomerulosclerosis. Science 308, 1801–1804. doi: 10.1126/science.1106215
Wright, D. J., Simmons, K. J., Johnson, R. M., Beech, D. J., Muench, S. P., and Bon, R. S. (2020). Human TRPC5 structures reveal interaction of a xanthine-based TRPC1/4/5 inhibitor with a conserved lipid binding site. Commun. Biol. 3:704. doi: 10.1038/s42003-020-01437-8
Wu, G., and Somlo, S. (2000). Molecular genetics and mechanism of autosomal dominant polycystic kidney disease. Mol. Genet. Metab. 69, 1–15. doi: 10.1006/mgme.1999.2943
Xian, W., Hui, X., Tian, Q., Wang, H., Moretti, A., Laugwitz, K.-L., et al. (2018). Aberrant deactivation-induced gain of function in TRPM4 mutant is associated with human cardiac conduction block. Cell Rep. 24, 724–731. doi: 10.1016/j.celrep.2018.06.034
Xie, J., Sun, B., Du, J., Yang, W., Chen, H.-C., Overton, J. D., et al. (2011). Phosphatidylinositol 4,5-bisphosphate (PIP(2)) controls magnesium gatekeeper TRPM6 activity. Sci. Rep. 1:146. doi: 10.1038/srep00146
Xu, H., Delling, M., Jun, J. C., and Clapham, D. E. (2006). Oregano, thyme and clove-derived flavors and skin sensitizers activate specific TRP channels. Nat. Neurosci. 9, 628–635. doi: 10.1038/nn1692
Xu, H., Delling, M., Li, L., Dong, X., and Clapham, D. E. (2007). Activating mutation in a mucolipin transient receptor potential channel leads to melanocyte loss in varitint-waddler mice. Proc. Natl. Acad. Sci. U.S.A. 104, 18321–18326. doi: 10.1073/pnas.0709096104
Yamashita, S., Mizumoto, H., Sawada, H., Suzuki, Y., and Hata, D. (2019). TRPV6 gene mutation in a dizygous twin with transient neonatal hyperparathyroidism. J. Endocr. Soc. 3, 602–606. doi: 10.1210/js.2018-00374
Yang, D., and Kim, J. (2020). Emerging role of transient receptor potential (TRP) channels in cancer progression. BMB Rep. 53, 125–132. doi: 10.5483/BMBRep.2020.53.3.016
Yang, F., Cui, Y., Wang, K., and Zheng, J. (2010). Thermosensitive TRP channel pore turret is part of the temperature activation pathway. Proc. Natl. Acad. Sci. U.S.A. 107, 7083–7088. doi: 10.1073/pnas.1000357107
Yang, Y., Wei, M., and Chen, L. (2022). Structural identification of riluzole-binding site on human TRPC5. Cell Discov. 8:67. doi: 10.1038/s41421-022-00410-5
Yao, J., Liu, B., and Qin, F. (2011). Modular thermal sensors in temperature-gated transient receptor potential (TRP) channels. Proc. Natl. Acad. Sci. U.S.A. 108, 11109–11114. doi: 10.1073/pnas.1105196108
Yazdani, M., Jia, Z., and Chen, J. (2020). Hydrophobic dewetting in gating and regulation of transmembrane protein ion channels. J. Chem. Phys. 153:110901. doi: 10.1063/5.0017537
Yeh, B.-I., Kim, Y. K., Jabbar, W., and Huang, C.-L. (2005). Conformational changes of pore helix coupled to gating of TRPV5 by protons. EMBO J. 24, 3224–3234. doi: 10.1038/sj.emboj.7600795
Yeh, B.-I., Sun, T.-J., Lee, J. Z., Chen, H.-H., and Huang, C.-L. (2003). Mechanism and molecular determinant for regulation of rabbit transient receptor potential type 5 (TRPV5) channel by extracellular pH. J. Biol. Chem. 278, 51044–51052. doi: 10.1074/jbc.M306326200
Yeh, F., Jara-Oseguera, A., and Aldrich, R. W. (2023). Implications of a temperature-dependent heat capacity for temperature-gated ion channels. Proc. Natl. Acad. Sci. U.S.A. 120:e2301528120. doi: 10.1073/pnas.2301528120
Yelshanskaya, M. V., and Sobolevsky, A. I. (2022). Ligand-binding sites in vanilloid-subtype TRP channels. Front. Pharmacol. 13:900623. doi: 10.3389/fphar.2022.900623
Yin, Y., Le, S. C., Hsu, A. L., Borgnia, M. J., Yang, H., and Lee, S.-Y. (2019a). Structural basis of cooling agent and lipid sensing by the cold-activated TRPM8 channel. Science 363:eaav9334. doi: 10.1126/science.aav9334
Yin, Y., Wu, M., Hsu, A. L., Borschel, W. F., Borgnia, M. J., Lander, G. C., et al. (2019b). Visualizing structural transitions of ligand-dependent gating of the TRPM2 channel. Nat. Commun. 10:3740. doi: 10.1038/s41467-019-11733-5
Yu, Y., Keller, S. H., Remillard, C. V., Safrina, O., Nicholson, A., Zhang, S. L., et al. (2009). A functional single-nucleotide polymorphism in the TRPC6 gene promoter associated with idiopathic pulmonary arterial hypertension. Circulation 119, 2313–2322. doi: 10.1161/CIRCULATIONAHA.108.782458
Yue, L., and Xu, H. (2021). TRP channels in health and disease at a glance. J. Cell Sci. 134:jcs258372. doi: 10.1242/jcs.258372
Yue, Z., Xie, J., Yu, A. S., Stock, J., Du, J., and Yue, L. (2015). Role of TRP channels in the cardiovascular system. Am. J. Physiol. Heart Circ. Physiol. 308, H157–H182. doi: 10.1152/ajpheart.00457.2014
Zappia, K. J. O’Hara, C. L., Moehring, F., Kwan, K. Y., and Stucky, C. L. (2017). Sensory neuron-specific deletion of TRPA1 results in mechanical cutaneous sensory deficits. eNeuro 4:ENEURO.0069-16.2017. doi: 10.1523/ENEURO.0069-16.2017
Zaydman, M. A., Silva, J. R., and Cui, J. (2012). Ion channel associated diseases: overview of molecular mechanisms. Chem. Rev. 112, 6319–6333. doi: 10.1021/cr300360k
Zhan, K., Yu, P., Liu, C., Luo, J., and Yang, W. (2016). Detrimental or beneficial: the role of TRPM2 in ischemia/reperfusion injury. Acta Pharmacol. Sin. 37, 4–12. doi: 10.1038/aps.2015.141
Zhang, C., Shine, M., Pyle, A. M., and Zhang, Y. (2022). US-align: universal structure alignments of proteins, nucleic acids, and macromolecular complexes. Nat. Methods 19, 1109–1115. doi: 10.1038/s41592-022-01585-1
Zhang, F., Jara-Oseguera, A., Chang, T.-H., Bae, C., Hanson, S. M., and Swartz, K. J. (2018). Heat activation is intrinsic to the pore domain of TRPV1. Proc. Natl. Acad. Sci. U.S.A. 115, E317–E324. doi: 10.1073/pnas.1717192115
Zhang, K., Julius, D., and Cheng, Y. (2021). Structural snapshots of TRPV1 reveal mechanism of polymodal functionality. Cell 184, 5138–5150.e12. doi: 10.1016/j.cell.2021.08.012
Zhang, X., Hu, M., Yang, Y., and Xu, H. (2018). Organellar TRP channels. Nat. Struct. Mol. Biol. 25, 1009–1018. doi: 10.1038/s41594-018-0148-z
Zhang, Z., Okawa, H., Wang, Y., and Liman, E. R. (2005). Phosphatidylinositol 4,5-bisphosphate rescues TRPM4 channels from desensitization. J. Biol. Chem. 280, 39185–39192. doi: 10.1074/jbc.M506965200
Zhang, Z.-M., Wu, X., Zhang, G., Ma, X., and He, D.-X. (2019). Functional food development: insights from TRP channels. J. Funct. Foods 56, 384–394. doi: 10.1016/j.jff.2019.03.023
Zhao, J., Lin King, J. V., Paulsen, C. E., Cheng, Y., and Julius, D. (2020). Irritant-evoked activation and calcium modulation of the TRPA1 receptor. Nature 585, 141–145. doi: 10.1038/s41586-020-2480-9
Zhao, S., Yudin, Y., and Rohacs, T. (2020). Disease-associated mutations in the human TRPM3 render the channel overactive via two distinct mechanisms. Elife 9:e55634. doi: 10.7554/eLife.55634
Zheng, J. (2013). Molecular mechanism of TRP channels. Compr. Physiol. 3:221–242. doi: 10.1002/cphy.c120001
Zhu, X., Chu, P. B., Peyton, M., and Birnbaumer, L. (1995). Molecular cloning of a widely expressed human homologue for the Drosophila trp gene. FEBS Lett. 373, 193–198. doi: 10.1016/0014-5793(95)01038-g
Zhu, X., Jiang, M., Peyton, M., Boulay, G., Hurst, R., Stefani, E., et al. (1996). trp, a novel mammalian gene family essential for agonist-activated capacitative Ca2+ entry. Cell 85, 661–671. doi: 10.1016/s0092-8674(00)81233-7
Zou, W.-B., Wang, Y.-C., Ren, X.-L., Wang, L., Deng, S.-J., Mao, X.-T., et al. (2020). TRPV6 variants confer susceptibility to chronic pancreatitis in the Chinese population. Hum. Mutat. 41, 1351–1357. doi: 10.1002/humu.24032
Zsombok, A., and Derbenev, A. V. (2016). TRP channels as therapeutic targets in diabetes and obesity. Pharmaceuticals 9:50. doi: 10.3390/ph9030050
Zubcevic, L., and Lee, S.-Y. (2019). The role of π-helices in TRP channel gating. Curr. Opin. Struct. Biol. 58, 314–323. doi: 10.1016/j.sbi.2019.06.011
Zubcevic, L., Borschel, W. F., Hsu, A. L., Borgnia, M. J., and Lee, S.-Y. (2019). Regulatory switch at the cytoplasmic interface controls TRPV channel gating. ELife 8:e47746. doi: 10.7554/eLife.47746
Keywords: Transient Receptor Potential Channels, TRP channels, TRP structures, ligand binding sites, activation and gating mechanism, drug design, drug targets
Citation: Huang J, Korsunsky A, Yazdani M and Chen J (2024) Targeting TRP channels: recent advances in structure, ligand binding, and molecular mechanisms. Front. Mol. Neurosci. 16:1334370. doi: 10.3389/fnmol.2023.1334370
Received: 07 November 2023; Accepted: 26 December 2023;
Published: 11 January 2024.
Edited by:
Deniz Yilmazer-Hanke, University of Ulm, GermanyReviewed by:
Yuichiro Fujiwara, Kagawa University, JapanKhursheed A. Wani, University of Massachusetts Medical School, United States
Alexander I. Sobolevsky, Columbia University, United States
Copyright © 2024 Huang, Korsunsky, Yazdani and Chen. This is an open-access article distributed under the terms of the Creative Commons Attribution License (CC BY). The use, distribution or reproduction in other forums is permitted, provided the original author(s) and the copyright owner(s) are credited and that the original publication in this journal is cited, in accordance with accepted academic practice. No use, distribution or reproduction is permitted which does not comply with these terms.
*Correspondence: Mahdieh Yazdani, mahdieh.yazdani@merck.com; Jianhan Chen, jianhanc@umass.edu