- 1Plant Molecular Biology Unit, Department of Botany, Sri Krishnadevaraya University, Anantapur, India
- 2Department of Plant, Food, and Environmental Sciences, Dalhousie University, Truro, NS, Canada
Drought stress has adverse effects on growth, water relations, photosynthesis and yield of groundnut. WRKY transcription factors (TFs) are the plant-specific TFs which regulate several down-stream stress-responsive genes and play an essential role in plant biotic and abiotic stress responses. We found that WRKY3 gene is highly up-regulated under drought stress conditions and therefore isolated a new WRKY3TF gene from a drought-adapted horsegram (Macrotyloma uniflorum Lam. Verdc.). Conserved domain studies revealed that protein encoded by this gene contains highly conserved regions of two WRKY domains and two C2H2 zinc-finger motifs. The fusion protein localization studies of transient MuWRKY3-YFP revealed its nuclear localization. Overexpression of MuWRKY3 TF gene in groundnut (Arachis hypogaea L.) showed increased tolerance to drought stress compared to wild-type (WT) plants. MuWRKY3 groundnut transgenics displayed lesser and delayed wilting symptoms than WT plants after 10-days of drought stress imposition. The transgenic groundnut plants expressing MuWRKY3 showed less accumulation of malondialdehyde, hydrogen peroxide (H2O2), and superoxide anion (O2•-), accompanied by more free proline, total soluble sugar content, and activities of antioxidant enzymes than WT plants under drought stress. Moreover, a series of stress-related LEA, HSP, MIPS, APX, SOD, and CAT genes found up-regulated in the transgenic groundnut plants. The study demonstrates that nuclear-localized MuWRKY3 TF regulates the expression of stress-responsive genes and the activity of ROS scavenging enzymes which results in improved drought tolerance in groundnut. We conclude that MuWRKY3 may serve as a new putative candidate gene for the improvement of stress resistance in plants.
Introduction
The abiotic stresses such as drought, heat, salt, and cold are the major causes for declined crop productivity worldwide. Combat to the stress, plants have evolved a sophisticated physiological and molecular networks. Drought is a severe environmental factor that significantly restricts the plant growth and productivity (Surendran et al., 2017). Drought stress causes changes in the cell membrane, osmolytes accumulation, inhibit photosynthesis traits, decrease biomass production and yield components in crop plants. (Binott et al., 2017). At the molecular level, several TFs like AP2/EREBP, NAC, WRKY, bZIP, MYB, and bHLH play a vital role in regulating downstream genes to protect plants from drought stress (Hennig, 2012). The WRKY TFs are one of the predominant families of plant-specific regulatory proteins in the plant kingdom and are known to participate in biotic and abiotic stress responses (Rushton et al., 2010; Liu et al., 2015; Sarris et al., 2015; Joshi et al., 2016). TFs play a vital role in stress responses by interacting with specific cis-acting elements present in gene promoters thereby regulating expression of down-stream elements (Birkenbihl et al., 2017; Chen et al., 2017). All WRKY TFs contain one or two conserved domains having approximately 60 amino acid residues with a conserved heptapeptide WRKYGQK, and a C2H2 or C2HC zinc finger-like motif (Eulgem et al., 2000). WRKY domain binds to the TTGACC/T W-box of the target gene and regulates its transcription (Brand et al., 2013; Bakshi and Oelmüller, 2014). WRKY TFs have been classified into three groups based on the number of WRKY domains present and the features of their zinc finger motif (Rushton et al., 2010; Rinerson et al., 2015a). Each domain of group I WRKY TFs appear to be functionally diverse; the C-terminal domain helps in specific binding to the W box, and the N-terminal domain is involved in increasing the affinity (Eulgem et al., 2000). In normal growth conditions, some members of WRKY TFs play regulatory roles like trichome development (Bakshi and Oelmüller, 2014), root growth (Grunewald et al., 2013), seed development (Schluttenhofer and Yuan, 2014), leaf senescence (Miao and Zentgraf, 2007; Rinerson et al., 2015b), dormancy (Ding et al., 2014), and secondary metabolites biosynthesis (Eulgem et al., 2000). In general, WRKY TFs can play multiple roles in plants by regulating the downstream related genes either positively or negatively (Dai et al., 2015).
The majority of deduced WRKY TFs are well-known for their functions in biotic stress response. Expression of NtWRKY3 and NtWRKY6 TFs was reported in Nicotiana attenuata during herbivore attacks (Skibbe et al., 2008). Lai et al. (2008) reported the elevated transcript abundance of AtWRKY3 and AtWRKY4, in Arabidopsis infected with Botrytis cinerea. Currently, research focus is on the functional characterization of WRKY proteins in response to abiotic stresses. Either up-regulation or down-regulation of an individual WRKY gene can result in improved abiotic stress tolerance (Wang et al., 2013; Dai et al., 2015). Several WRKY TF gene family members responsive to abiotic stresses have also been reported WRKY TF genes like WRKY25, WRKY26, and WRKY33 were recognized to regulate cross-talk between ethylene and heat shock protein response related signaling pathways (Li et al., 2011). A multiple abiotic stress responsive TaWRKY10 up-regulated during PEG, NaCl, cold, and H2O2 treatment was reported in wheat (Wang et al., 2013). In contrast to other abiotic stresses, few WRKY TFs were identified specifically to drought stress response. The expression of WRKYs in response to drought such as AtWRKY57 from Arabidopsis (Jiang et al., 2012), GsWRKY20 from soybean (Luo et al., 2013), and ZmWRKY58 from maize (Cai et al., 2014) have been investigated. The overexpression of WRKY genes in plants showed enhanced tolerance to several abiotic stresses. For example, overexpression of OsWRKY11 in rice displayed an improved tolerance to high temperature and salt (Wu et al., 2009). Also, overexpression of several cotton WRKY genes (GhWRKY17, GhWRKY34, and GhWRKY41) showed an increased salt and drought tolerance in tobacco (Yan et al., 2014; Chu et al., 2015; Zhou et al., 2015). Further, overexpression of two wheat WRKY genes (TaWRKY19 and TaWRKY93) in Arabidopsis confers higher tolerance to salt and drought stress (Niu et al., 2012; Qin et al., 2015). These genes conferred tolerance to abiotic stresses in plants through detoxification of cytotoxic compounds (ROS and RCC scavenging), with improved osmotic adjustment, maintaining membrane stability, and by regulating the stress-responsive genes. Though, a few researchers reported the role of WRKY TFs in abiotic stress responses in model plants, the functions of most WRKY TFs from non-model plants remains underexplored. Horsegram, a drought-adapted grain-legume has the unique ability to grow in poor soils under receding moisture conditions. Groundnut is important oil yielding seed-legume crop grown in semi-arid regions of the world in rain-fed areas and is frequently encounters to drought spells of different duration and intensities. Considering the importance of groundnut in dry-land agriculture, its tolerance to drought raises an important issue for groundnut improvement programs. Keeping in mind the various functions of WRKY TFs under complex environmental conditions and to further understand the function of WRKY TFs, we isolated and funcationally characterized a drought stress-responsive MuWRKY3 gene from horsegram. Transgenic groundnut plants overexpressing MuWRKY3 exhibited improved drought stress tolerance compared to wild-type (WT) suggesting that MuWRKY3 can serve as a new candidate gene for improving the tolerance to drought stress in crop plants.
Materials and Methods
Plant Material and Stress Treatments
The pot-cultured horsegram plants were grown for 19-days in botanical garden under natural photoperiod (10–12 h; 27 ± 4°C). Stress treatments included were, (1) drought stress imposed to plants by withholding water and leaf samples were collected at wilting stage, (2) salt stress imposed by adding 2% NaCl solution to the pots and collected samples at 72 h after treatment, (3) for dehydration stress, detached leaves were allowed to dry on filter paper in ambient conditions for 8 h, (4) for cold stress treatment potted plants were subjected to 10°C for 48 h, and (5) for heat stress treatment plants were subjected to 50°C for 8 h in a temperature controlled growth chamber. The samples were flash frozen in liquid nitrogen and stored at -80°C for further analysis.
RNA Extraction and Quantitative Real-Time PCR Analysis
Total RNA from horsegram leaf samples was extracted using TRIzol reagent method. DNase treatment of extracted total RNA was performed using the TURBO DNA-free kit (Ambion, United States). 200 ng of RNA was used to synthesize cDNA and subsequently used as a template for PCR and quantitative RT-PCR analysis (Hu et al., 2012). Expression analysis was performed using Real-time PCR-System (AB StepOne, United States) with three biological replicates and actin as an internal control. The relative quantification was studied using 2-ΔΔCt method (Livak and Schmittgen, 2001) to evaluate quantitative variations in transcript level between three replicates. Primers used in the RT-PCR assay are included in (Supplementary Table S1).
MuWRKY3 Gene Cloning and Phylogenetic Analysis
Gene-specific primers were used to amplify the full-length gene from horsegram cDNA (Supplementary Table S1). The amplified full-length product was cloned into pTZ57R/T vector and sequenced. WRKY3 sequences from different plants were downloaded from Plant TF Database and compared with the MuWRKY3 sequence. Multiple sequence alignment was done using ClustalX software. Unrooted Neighbor-joining Tree used to perform the phylogenetic tree analysis. Tree view software produced a graphical representation, and internal branching support was estimated using 1000 bootstrap replicates and 111 random odd numbers. Conserved domains were predicted by using NCBI conserved domain database. Molecular weight and isoelectric point were predicted using online software Expasy1. Subcellular localization was predicted by Plant-mPLoc 2.02 (Chen et al., 2010) (Supplementary Figure S4).
Vector Construction and Plant Transformation
A gene construct overexpressing MuWRKY3 was generated by cloning full-length gene to binary vector pCAMBIA2301 under a constitutive 35S promoter. Agrobacterium EHA105 is carrying CaMV35S:MuWRKY3 with kanamycin as a selectable marker was used for plant transformation. Transgenic plants were generated by in planta transformation (Rohini and Rao, 2001). MuWRKY3 gene fused with YFP (35S:MuWRKY3::YFP) was used for localization studies. MuWRKY3 gene was cloned in pDONOR207 (Invitrogen) using gateway cloning method, and the gene was subsequently cloned into a binary vector pAM-PAT-p35S-YFP (Bernoux et al., 2008). The plant expression vector expressing pAM-PAT-p35S-MuWRKY3-YFP and pAM-PAT-p35S-YFP were transformed into Agrobacterium tumefaciens strain EHA105.
Sub Cellular Localization and Transactivation Assay
Agrobacterium EHA105 carrying pAM-PAT-p35S-MuWRKY3-YFP and pAM-PAT-p35S-YFP were introduced into Nicotiana benthamiana leaves using a needle-less syringe (Li et al., 2014). After 48 h of incubation, the leaf samples were observed for YFP signal. YFP excitation was performed at 515 nm, and emission was spotted in the range of 525–600 nm using a confocal laser scanning microscope (Olympus, FV1000, Germany). The transactivation activity of MuWRKY3 genes was investigated by yeast one-hybrid assay using strain AH109. The vector pGBKT7 carrying MuWRKY3 (pBD), His-tag reporter gene (pGBKT7-MuWRKY3) and pBD were transformed into yeast, following Clontech yeast protocol handbook. Colony PCR was performed for selecting the positive colonies. The yeast strains were streaked on SD/-Trp and SD/-His plates containing five mM 3-amino-1, 2, 4-triazole (3-AT) for transcriptional activity assay.
Analysis of Gene Integration and Overexpression
The seeds harvested from T0 plants were sown on MS medium containing 200 mg/L kanamycin, and the kanamycin survived plants were selected as true transformants (Herrera-Estrella et al., 1983). The presence and integration of transgene were further confirmed by PCR analysis from genomic DNA using gene-specific WRKY3, NptII and GUS primers (Supplementary Table S1). GUS enzyme activity was assessed in seedlings of putative transformants and WT (Jefferson, 1987). After confirmation, positive transgenic plants were advanced to further generations. Putative transgenic plants were confirmed by PCR amplifications to select T2 and T3 seeds. QRT-PCR used to quantify transcript abundance of the transgene. Southern blot analysis was done to confirm the stable transgene integration in T3 generation transgenic line. Genomic DNA digested with, BamHI at 37°C and GUS probe was used for analysis. Blotting was performed using Biotin-labeled PCR amplified gene fragment of GUS probe (Dai et al., 2015). The hybridized membrane was washed and detected according to manufacturer’s instructions (Thermo Scientifics Biotin DecaLabel DNA Labeling Kit, Germany).
Stress Tolerance Assays of Transgenic and WT Plants
To assess the drought tolerance, pot grew 30-day-old groundnut transgenic, and WT plants were subjected to drought stress by withholding water for 10 days. Phenotypic differences between WT and transgenic groundnut plants were recorded. Reactive oxygen species, superoxide anions were measured according to Doke (1983) and hydrogen peroxide content by Singh et al. (2006), lipid peroxidation were estimated by measuring the MDA content according to Hodges et al., 1999. Leaf free proline content was measured by Ninhydrin method (Bates et al., 1973). Leaf total soluble sugars were measured by Arnon (1949) and antioxidative enzyme activities, SOD by Giannopolitis and Ries (1977) and APX by Nakano and Asada (1981) were determined sectrophotometrically (Shimadzu UV-1800, Japan).
Statistical Analysis
To check the significant differences in all the physiological and biochemical experiments were subjected to the SPSS statistic data software (version 16.0) and evaluated using one-way ANOVA post hoc multiple comparisons from the Duncan’s test at a significance level of P ≤ 0.05.
Results
Expression Patterns of MuWRKY3 Under Various Stress Treatments
The quantitative RT-PCR analysis was performed to determine the transcript abundance patterns of MuWRKY3 in leaf tissues of horsegram under unstressed and several imposed abiotic stresses. The results showed that expression of MuWRKY3 was detected in leaf tissues under dehydration, drought, heat, salt, and cold treatments. Although the WRKY3 gene was highly up-regulated in all stress conditions, its expression was significantly higher under drought stress condition (Figure 1A) than under other stresses. Salt stress (200 mM NaCl) treatment caused an increase in MuWRKY3 transcript abundance by 3.4-fold, heat stress by 6.1-fold, low temperature (cold) treatment led to up-regulation by 5.0-fold. During dehydration and drought treatments, the transcription of MuWRKY3 performed a significant up-regulation by 9.4- and 13.5-fold, respectively.
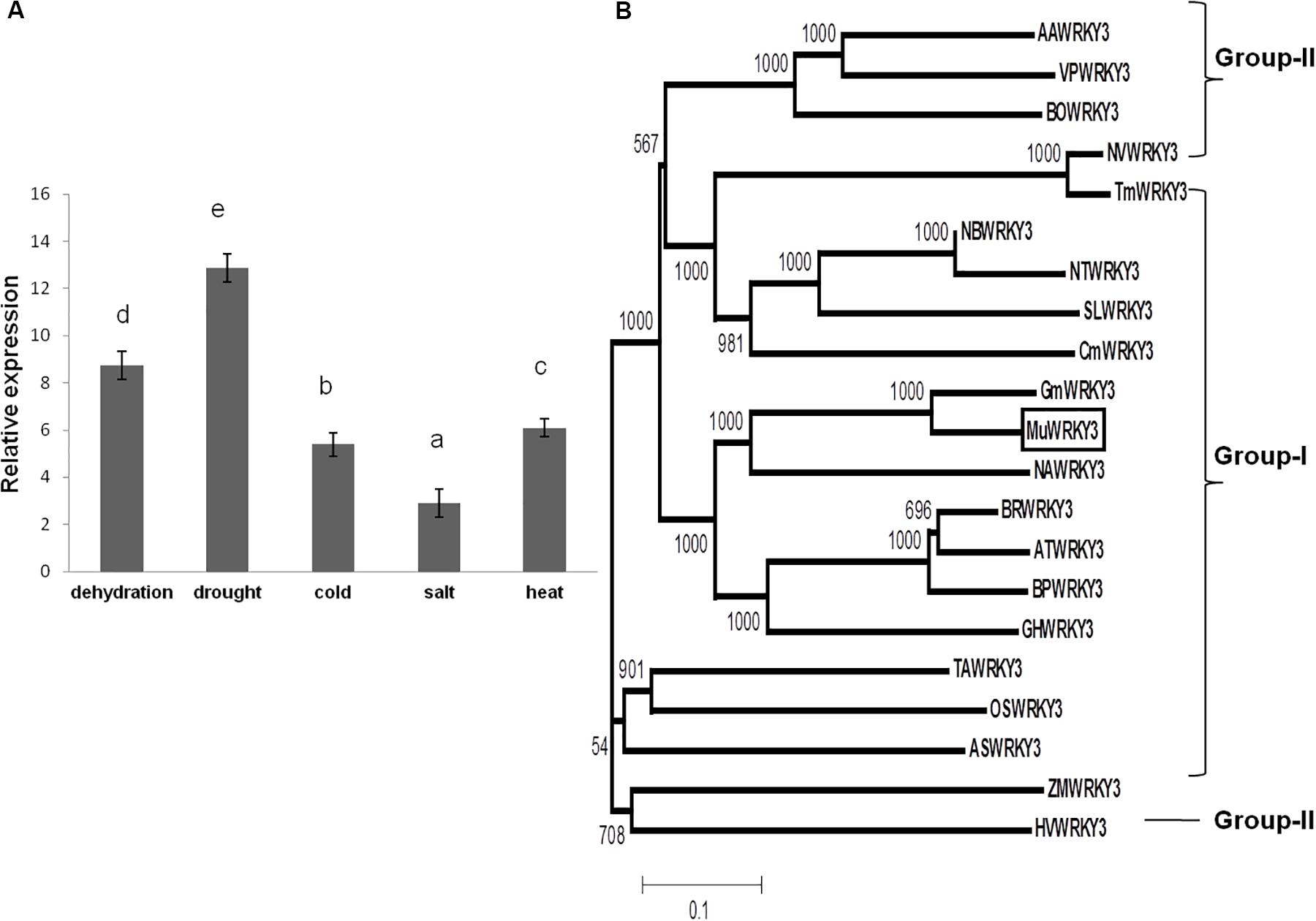
FIGURE 1. (A) Expression of MuWRKY3 in horsegram under various abiotic stresses as determined by real-time PCR analysis. Error bars represent standard deviations (SD) for three independent replicates with different letters at P < 0.05 significant level. (B) Unrooted Bootstrapped Neighbor Joining tree analysis of MuWRKY3 and WRKY protein sequences from different species. The amino acid sequences of the conserved WRKY domain region were aligned using ClustalX version 2.1. MuWRKY3 is boxed in the figure (Supplementary Figure S5).
Cloning and Sequence Annotation Analysis of MuWRKY3
Horsegram (Macrotyloma uniflorum Lam. Verdc.) is well adapted to semi-arid conditions with higher drought tolerance ability. From, our previous study, the MuWRKY3 gene was found to be highly up-regulated among eight different WRKY genes studied (Kiranmai et al., 2016). In this study, the full-length WRKY3 gene was successfully isolated from horsegram and designated as MuWRKY3 (GenBank Accession: KM520390.1). Sequence analysis presented that the MuWRKY3 gene is 1476 bp with coding a deduced protein of about 490 amino acids with molecular weight of 53.7 kDa and an isoelectric point of 6.12. MuWRKY3 protein conserved domain analysis displayed that the presence of two WRKY domains and a C2-H2 zinc finger motif and belongs to group I of WRKY TF superfamily (Rushton et al., 2010; Huang et al., 2012) (Supplementary Figures S1–S3).
Phylogenetic Relationship of MuWRKY3 With Other WRKY Proteins
To characterize the divergence of the isolated MuWRKY3 protein with the other plant WRKY3 proteins, domain proteins were analyzed by ClustalX alignment (Figure 1B). The phylogenetic analysis revealed that MuWRKY3 was clustered into group I of the WRKY TF family and most closely associated to GmWRKY3 than from NaWRKY3, GhWRKY3, AtWRKY3, BpWRKY3, and BrWRKY3.
MuWRKY3 Gene Localizes to Nucleus and Exhibit Transcriptional Activity
To determine the subcellular localization of the MuWRKY3 gene was fused to N-terminal with YFP reporter gene that was expressed under the constitutive promoter (35S CaMV). The Agrobacterium harboring 35S::MuWRKY3-YFP and 35S::YFP constructs was infiltrated to 3-week-old tobacco leaves by needleless syringe. The microscopic image clearly shows that MuWRKY3-YFP protein is accumulated in the nucleus and the YFP alone occurred all over the cell organelles including cytoplasm and nucleus (Figure 2A). Further, yeast transactivation assay was carried out for MuWYKY3 gene in yeast cells revealed the activation of His-reporter gene in the presence of MuWRKY3 protein. The yeast cells transformed with pBD-MuWRKY3 grew well in SD/-Trp and SD/-His plates containing 5 mM 3-amino-1, 2, 4-triazole (3-AT) where pBD could survive in SD/-Trp medium only (Figure 2B). Together these results suggest that MuWRKY3 is nuclear-localized protein serving as a TF.
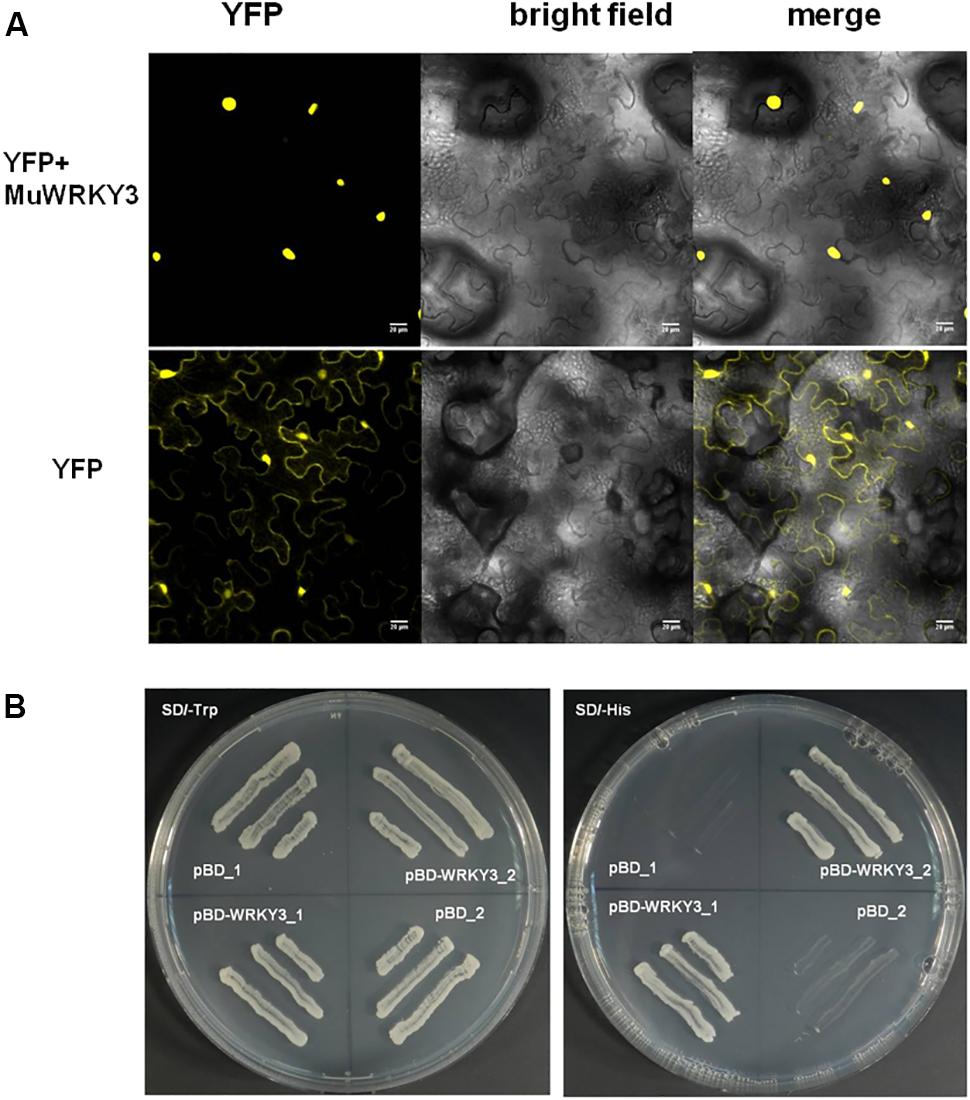
FIGURE 2. (A) Sub-cellular localization of YFP and MuWRKY3:YFP in the epidermal peels of Nicotiana Benthamiana. (B) Transactivation activity analysis of MuWRKY3 was performed using yeast strain AH109. The transformants pBD-WRKY3 and pBD (pGBKT7) were streaked on SD/-Trp and SD/-His plates containing 5 mM 3-amino-1, 2, 4-triazole (3-AT).
Generation of Transgenic Groundnut Plants Overexpressing MuWRKY3
To study the functional relevance of MuWRKY3 gene in response to drought stress the groundnut transgenic expressing MuWRKY3 driven by the CaMV 35S promoter was developed (Figure 3A). A tissue culture independent Agrobacterium mediated in planta transformation method was adopted to develope the groundnut transgenics (Rohini and Rao, 2001). The putative transformants were analyzed in T1, T2, and T3 generation for the resistance to kanamycin (Figure 3B), drought tolerance and integration of the gene by PCR. Lines resistant to kanamycin were selected and advanced next generation (Supplementary Figure S6). The transgenics were also confirmed for the activity of glucoronidase due to the expression of Gus gene. The transgenic cotyledons clearly demonstrate that the higher activity of glucoronidase (Figure 3C). The presence of the transgene in transgenic groundnut plants in T3 generation confirmed by PCR analysis using gene-specific primers of WRKY3, GUS, and NptII(Figure 3D).
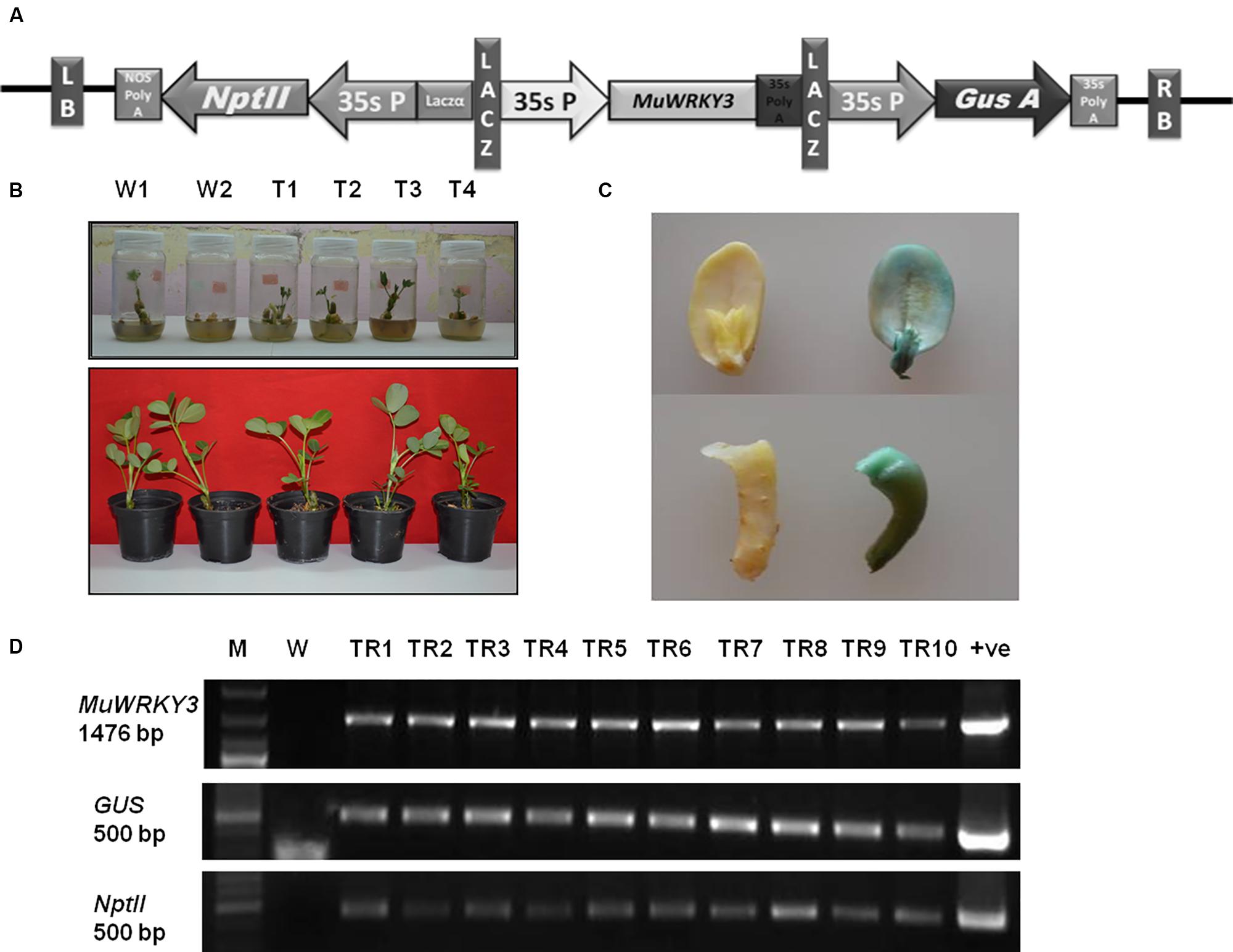
FIGURE 3. (A) A linear map of MuWRKY3 overexpressing vector with GUS and NptII marker genes for screening, 35S promoter and polyA terminator. Entire expression cassette is flanked by left and right borders in pCAMBIA2301. (B) Screening of transgenic and WT plants on half strength MS medium containing 200 mg/lit kanamycin and their acclimatization. (C) GUS histochemical staining of WT and transgenic seedlings at T3 generation. (D) PCR Amplification of MuWRKY3 gene GUS and NptII gene from genomic DNA of transgenic and WT plants: M, gene ruler mix; WT, wild-type; TR1 to TR10, transgenic lines.
Transgenic Groundnut Plants Expressing MuWRKY3 Showed Improved Drought Tolerance
Under unstressed conditions, all groundnut lines showed no visible phenotypic differences (unpublished data). However, WT and independent T3 lines exposed to drought stress for 10 days showed stress-induced wilting, but visible wilting symptoms appeared much earlier in WT plants. The transgenic plants remained green after 10 days of drought stress (Figure 4A). Under stress conditions, the transgenic plants showed significantly reduced levels of lipid peroxidation end product, malondialdehyde (MDA) (Figure 4B). The MDA content was 3.5- to 2-fold lesser in transgenic plants than in WT. The groundnut transgenics expressing MuWRKY3 maintained a higher amount of free proline and total soluble sugar content than the WT plants even after 10 days of drought stress imposition (Figures 4C,D), resulting in a range of 1.5- to 2.5-fold and 2.3- to 4.4-fold increase in the amount of proline and total soluble sugar contents, respectively, in transgenic lines was observed.
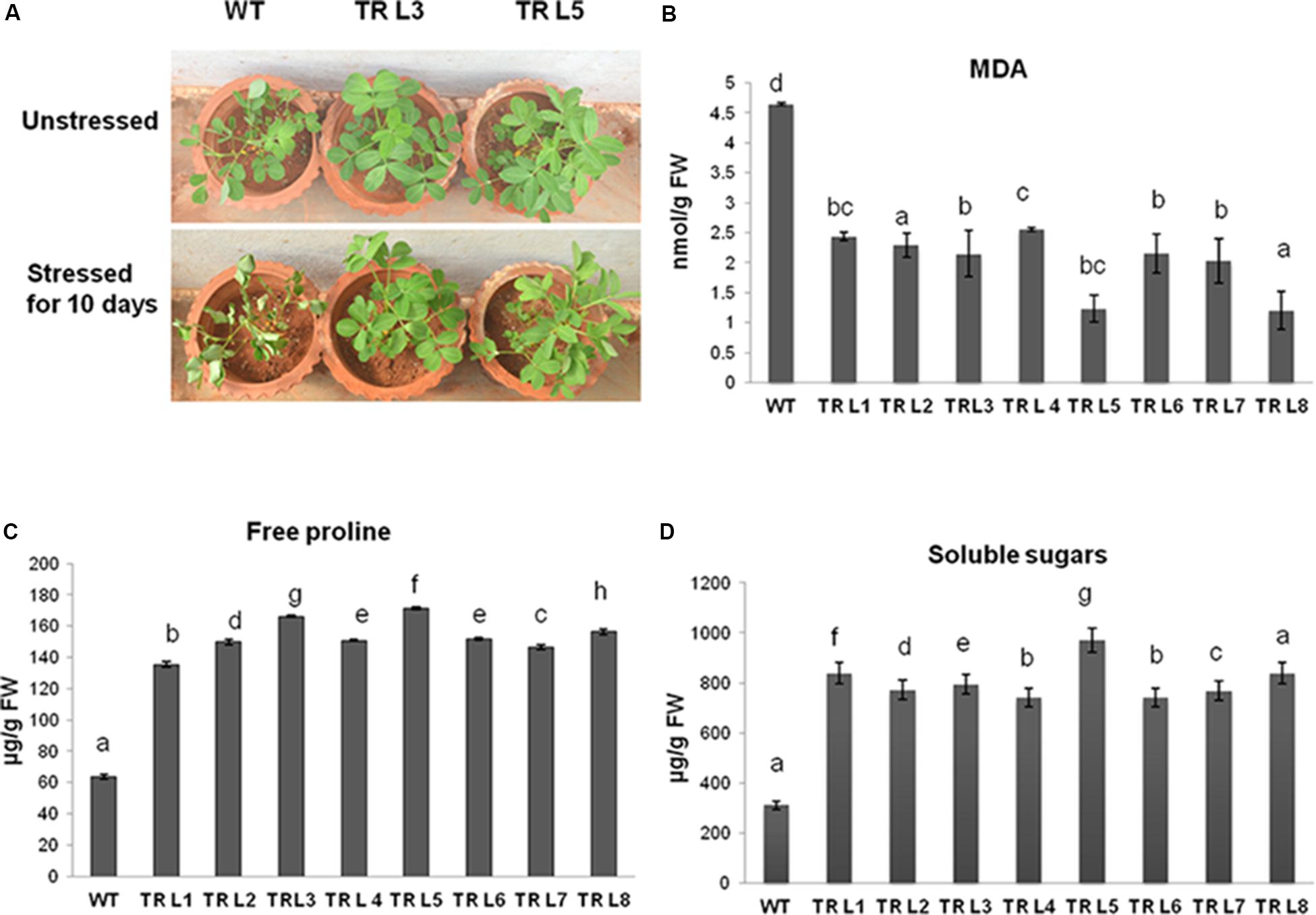
FIGURE 4. (A) Phenotypic differences in transgenic lines and WT groundnut plants under unstressed and drought stressed for 10 days. (B) MDA content in WT and transgenic plants under stress. (C) Free proline content and (D) total soluble sugars in transgenic and WT plants under drought stress conditions (WT- WT, TRL1 to TRL8 – Transgenic lines). Data is analyzed using SPSS version 16.0, values shown is the mean of three replicates and ±SE of three replicates and letters shown above the bars are significantly different at P < 0.05 (DMR).
Transgenic Groundnut Expressing MuWRKY3 Increases Antioxidant Enzymes to Reduce Oxidative Stress Damage
The ability of transgenic groundnut plants to withstand the oxidative stress damage was studied by exposing the plants to drought stress conditions. The antioxidant enzyme activity (SOD and APX) was significantly higher in transgenic lines compared to WT. The SOD activity was three- to fivefold more in transgenic lines than in WT. Similarly, APX activity was three- to sevenfold more in transgenic plants than in WT (Figures 5A,B). The stress-induced production of O2•- and H2O2 radicals were higher in WT plants than that of transgenic lines (Figures 5C,D). These results were also supported by the in situ histochemical staining of O2•- with NBT and H2O2 ions with DAB. Remarkable differences were observed where WT plants accumulated higher free radicals than that of transgenic plants (Figures 5E,F). These results suggest that transgenic plants overexpressing MuWRKY3 gene showed better tolerance to drought with increased antioxidant efficacy.
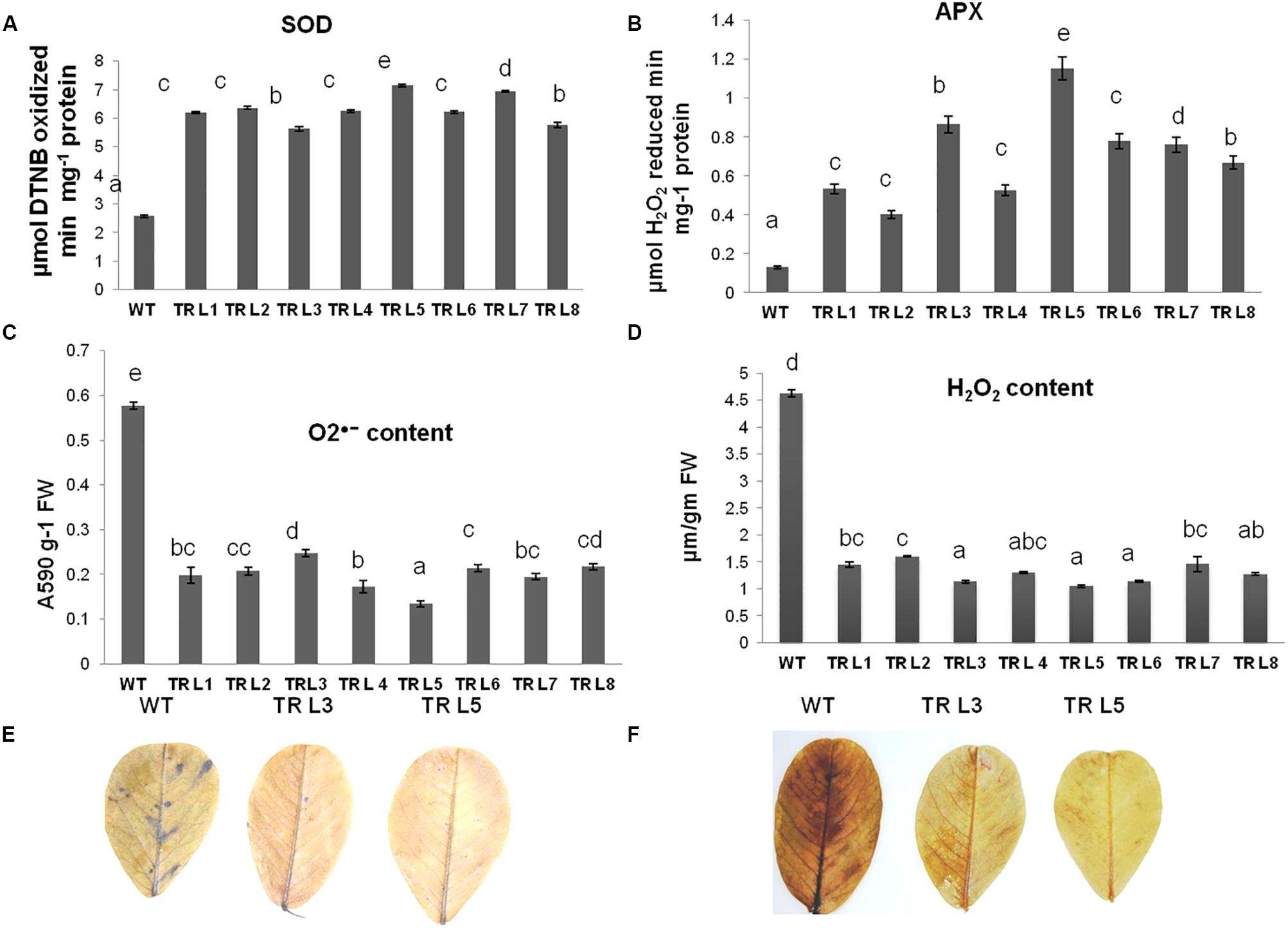
FIGURE 5. Physiological characterisation of WT and MuWRKY3 transgenic plants under drought stress conditions. Antioxidant enzymes: (A) SOD (B) APX activity in WT and transgenic lines under drought stress conditions. (C) O•- anions produced in WT and transgenic plants under stress conditions. (D) H2O2 ions produced in WT and transgenic plants under stress. (E) In situ histochemical localization of O•- generated under drought stress using DAB. (F) In situ histochemical localization of H2O2 production under stress using NBT. Data is analyzed using SPSS version 16.0, values shown is the mean of three replicates and ±SE of three replicates and letters shown above the bars are significantly different at P < 0.05 (DMR).
Transgenic Groundnut Plants Are Stable and Showed Increased Expression of Stress-Responsive Genes
Selected groundnut transgenic plants are tested for the stable integration of gene by Southern blot analysis in a T3 generation. The variation in the integration pattern between transgenic lines demonstrated the independent transgenic events and also confirmed transgenic nature of groundnut plants (Figure 6A).
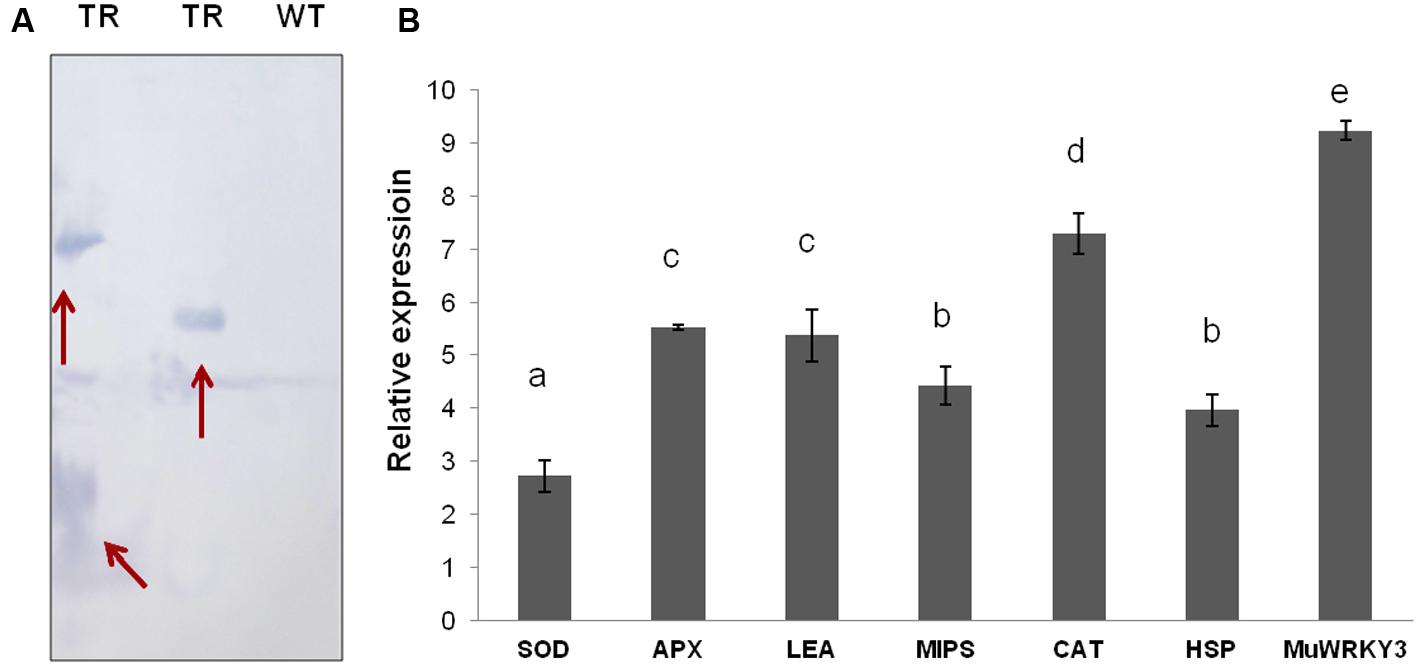
FIGURE 6. (A) Southern blotting analysis of transgenic plants for the detection of positive plants with stable integration (TR, transgenic line; WT, wild-type). (B) qRT-PCR analysis of MuWRKY3 and stress responsive genes under drought stress conditions.
Further understanding of the WRKY TF role in the mechanism of drought tolerance, the selected six down stream stress-responsive gene expression was studied and compared between WT and MuWRKY3 transgenic groundnut plants under drought stress conditions. An efficient antioxidant system is crucial to alleviate the oxidative damage caused by drought stress. QRT-PCR analysis studies used to check the gene expression of ROS-scavenging enzymes (CAT, SOD, and APX genes) and other stress responsive genes such as MIPS, LEA, and HSP. Drought stress increased the transcript levels of CAT, SOD, and POD in transgenic lines. The drought stress-induced expression of all six selected stress-responsive genes showed elevated expression in transgenic groundnut plants.The increases in transcript levels of 2.71-fold for SOD, 5.5-fold for APX, 7.3-fold for CAT, 4.4-fold for MIPS, 5.3-fold for LEA, 4.0-fold for HSP, 9.4-fold for MuWRKY3 over those in WT plants (Figure 6B). Overall these results suggest that over-expression of MuWRKY3 regulates the expression of downstream stress-responsive genes under drought conditions.
Discussion
Drought is one of the major abiotic factors that accounts for 30–70 percent of crop yield loss worldwide. At whole plant level, drought stress causes a series of morphological, physiological, biochemical, and molecular changes that effect plant growth, development and productivity (Rahdari and Hoseini, 2012; Ali et al., 2014). Effect of drought on plants growth (Rampino et al., 2006), water content (Jaleel et al., 2009) decrease nutrient uptake (Selvakumar et al., 2012), decrease in photosynthesis (Rahdari et al., 2012) resulting in the severe damage and yield loss was extensively studied. Many research efforts have been made to produce the crops tolerant to drought through plant breeding and genetic engineering technologies. Transcription factors (TFs) are the ideal candidates to engineer crops for improved tolerance as they regulate many downstream functional genes (Agarwal et al., 2011). Under stress conditions the TFs interact with cis-elements in the promoter region of the gene and up-regulate the expression of many downstream genes imparting stress tolerance (Agarwal et al., 2011; Li et al., 2013). Overexpression of TFs imparting plant drought stress was previously reported. Expression of MYB (Seo et al., 2011; Shin et al., 2011; Nakashima et al., 2014), bZIP TF genes (Banerjee and Roychoudhury, 2017) and NAC family TFs (Shao et al., 2015) imparting drought stress tolerance was extensively studied in plants. These TFs impart stress tolerance by altering the biochemical characters like osmolyte accumulation (Wang et al., 2009; Niu et al., 2014), antioxidant enzyme production (Shi et al., 2014; Chu et al., 2016) and by the regulation of stress responsive genes (Zheng et al., 2013; Wang et al., 2015).
WRKY TF are the largest TF family proteins bind to the specific TTGAC(C/T) W-box elements in the promoters of a many plant defense-related genes (Brand et al., 2013). Based on the number of WRKY domains and the nature of their zinc-finger motif the WRKY TFs are classified into three groups. The presence of two conserved WRKY domains and a C2H2 zinc finger motifs in the WRKY3 TF gene of horsegram reveals that its a group I of WRKY superfamily proteins. Phylogenetic analysis showed that MuWRKY3 is clustered with GmWRKY3 from soybean. The previous studies suggest that WRKY genes play a vital role in pathogen-defense mechanisms (Dang et al., 2013; Cai et al., 2015; Sarris et al., 2015) and abiotic stress responses (Luo et al., 2013; Banerjee and Roychoudhury, 2015; Dai et al., 2015). In our study, the rapid up-regulation of MuWRKY3 gene was induced by drought, dehydration, heat, salt, and cold stress. Similar results were reported from previous studies where multiple abiotic stresses highly induced the expression of TaWRKY10 (Wang et al., 2013) and TaWRKY2 genes (Niu et al., 2012).
Transcription factors are known to be located in the nucleus, where they bind to specific gene promoter sequences, thereby controlling the downstream elements. The result obtained from the subcellular localization of MuWRKY3 showed that the YFP was located in the nucleus, suggesting that this gene function could be nuclear localized. A similar result was observed when soya bean WRKY20 was expressed in transgenic Arabidopsis plants, where green fluorescence protein localization was observed in the nucleus (Luo et al., 2013). We employed a standardized tissue culture-independent Agrobacterium-mediated in planta transformation protocol to generate transgenic groundnut lines overexpressing MuWRKY3 (Rohini and Rao, 2001). The selection of putative transgenic plants was carried out from the T1 generation as the primary transformants (T0) are chimeric in nature (Rohini and Rao, 2001; Kumar et al., 2009).
The previous studies has reported the improvement of drought tolerance in plants by expression of WRKYTF genes (Luo et al., 2013; Wang et al., 2013; Chu et al., 2015). An increase in the expression of the OsWRKY30 in transgenic rice was identified in response to drought by Shen et al. (2012). The GmWRKY20 TF gene derived from wild soybean has improved the drought tolerance in transgenic Arabidopsis plants through ABA-mediated signaling (Luo et al., 2013). Similarly, the overexpression of MuWRKY3 gene in groundnut transgenics showed enhanced tolerance to drought stress. The improved cellular level tolerance achieved by this MuWRKY3 expression and regulation of other stress-responsive genes could be partialiy attributed to delayed wilting, stay green nature, several physiological and biochemical changes in the groundnut transgenics.
Firstly, accumulation of osmolytes such as soluble sugars and free proline is an important phenomenon to facilitate the osmoregulation in response to abiotic stress (Garg et al., 2002; Singh et al., 2015). Higher accumulation of free proline and soluble sugars was observed in MuWRKY3 transgenic plants compared to WT plants under drought stress condition. Consequently, the higher accumulation of osmolytes in the MuWRKY3 transgenic plants may partially contribute to the enhanced drought tolerance. Similarly, the transgenic alfalfa plants overexpressing WRKY20 from Glycine soja have accumulated more free proline and soluble sugars under drought stress (Tang et al., 2014). BhWRKY1 from Boea hygrometrica regulating the accumulation of osmolytes in response to water stress was also reported (Wang et al., 2009).
Secondly, higher accumulation of cytotoxic compounds (ROS and RCC) during drought stress can cause severe oxidative damage in plants. The lipid peroxidation induced by higher ROS accumulation produces a wide range of degradation products, such as MDA, methylglyoxal (MG), HNE, which are responsible for severe electrolyte leakage. The MuWRKY3 transgenics showed lower levels of MDA under drought stress; this may be due to lower O•- and H2O2 levels. Abiotic stress can cause lipid peroxidation, leading to accumulation of MDA that reflect the high degree of damage triggered by stress (Sathiyaraj et al., 2011). The continuous monitoring of ROS in the cell is essential, and equilibrium between the generations of ROS and scavenging depends on antioxidative enzymes (Foyer et al., 2008; Suzuki et al., 2012). Plants with a high level of antioxidant enzymes are relatively more tolerant to abiotic stress (Peleg and Blumwald, 2011). The oxidative stress-responsive genes such as SOD, APX, and CAT catalyze the degradation of ROS produced during stress (Zheng et al., 2013; Wang et al., 2015). The MuWRKY3 transgenic plants showed higher activity of antioxidative enzymes like CAT, SOD, and APX compared to WT plants under drought stress.). The results suggest that the over-expression of MuWRKY3 confers drought stress tolerance in transgenic plants by reducing the effect of ROS and its accumulation.
Thirdly, to understand the specific role of MuWRKY3 during drought stress, we studied the expression of six stress-responsive genes such as SOD, APX, CAT, MIPS, LEA, and HSP in WT and transgenic lines. The transgenic plants have shown the up-regulation of all six stress-related genes with highest transcript abundance in CAT. Wang et al. (2015) reported that the transcript levels of antioxidant genes were increased in transgenic tobacco plants expressing the TaWRKY44 gene under drought stress treatments. Overexpression of ThWRKY4 conferred tolerance to salt and oxidative stress by modulating ROS by expression of SOD and APX genes (Zheng et al., 2013). Up-regulation of HSP proteins like HSP (LOC_ Os0g16061) 358 and HSP (LOC_Os9g31486) was observed in rice plants expressing OsWRKY30 under drought stress (Shen et al., 2012). In the present study, we report that the expression of MuWRKY3 in transgenic plants is up-regulating the MIPS gene under drought stress conditions. LEA proteins were known to accumulate during water stress conditions and help plants to survive (Garay-Arroyo and Covarrubias, 1999) by stabilizing macromolecules and cellular structures (Liu et al., 2013). Transgenic tobacco expressing TaWRKY44 showed elevated expression of LEA type genes and confers drought tolerance (Wang et al., 2015). Positive correlations between expression of HSPs and heat shock (HS) tolerance have been reported previously (Burke and Chen, 2015). MIPS gene is known to involve in membrane formation, cell wall biogenesis, stress response, and signal transduction (Kaur et al., 2013; Goswami et al., 2014). Zhai et al. (2016) as reported that an myoinositol-1-phosphate synthase gene(IbMIPS1) has enhanced the salt and drought tolerance in transgenic sweet potato plants. The results indicate that HSP and MIPS genes were up-regulated under drought stress conditions in transgenic plants compared to WT plants. Up-regulation of HSP in rice plants expressing OsWRKY30 under drought stress was previously reported (Shen et al., 2012). The gene expression studies demonstrated that MuWRKY3 gene regulates the expression of drought stress-responsive genes. These results are supported by the previous studies that the stress tolerance achieved in transgenic plants expressing WRKY genes by up-regulation of stress-related genes (Hu et al., 2013; Wang et al., 2015).
Conclusion
In summary, the horsegram MuWRKY3 gene belonging to group I of the WKRY superfamily is a stress-inducible TFs. Overexpression of MuWRKY3 in groundnut enhanced drought stress tolerance. MuWRKY3 transgenic plants showed up-regulation of many stress-inducible functional genes involved in diverse cellular mechanisms. The oxidative stress effect is reduced due to higher expression and activity of antioxidant enzymes. The study demonstrates that MuWRKY3 could be a candidate gene for transgenic approaches to improve the stress tolerance in crop plants for sustained growth and productivity under drought conditions.
Ethics statement
All the experiments were approved by RCGM, Government of India No./BT/BS.17/686/2016-PID.
Author Contributions
CS conceived and designed the experiments. KK, MP, AN, and VAR performed the research. GLR performed the transactivation assays. UL and BV produced the transgenics. AAJ performed the gene annotations. CS, GLR, and KK wrote the paper. All authors provided inputs to develop the manuscript.
Funding
A part of the research was supported by DBT, Government of India, New Delhi.
Conflict of Interest Statement
The authors declare that the research was conducted in the absence of any commercial or financial relationships that could be construed as a potential conflict of interest.
Acknowledgments
We acknowledge the DBT-(BT/PR15503/AGR/02/913/2015), Government of India, New Delhi for a part of financial assistance in the form of research grants to CS. We thank Dr. V. Ramu, University of Agricultural Sciences, Bangalore for reading the manuscript and his valuable comments.
Supplementary Material
The Supplementary Material for this article can be found online at: https://www.frontiersin.org/articles/10.3389/fpls.2018.00346/full#supplementary-material
Footnotes
References
Agarwal, P., Reddy, M. P., and Chikara, J. (2011). WRKY: its structure, evolutionary relationship, DNA-binding selectivity, role in stress tolerance and development of plants. Mol. Biol. Rep. 38, 3883–3896. doi: 10.1007/s11033-010-0504-5
Ali, S. K. Z., Sandhya, V., and Rao, L. V. (2014). Isolation and characterization of drought-tolerant ACC deaminase and exopolysaccharide-producing fluorescent Pseudomonas sp. Ann. Microbiol. 64, 493–502. doi: 10.1007/s13213-013-0680-3
Arnon, D. I. (1949). Copper enzyme polyphenoloxidases in isolated chloroplast in Beta vulgaris. Plant Physiol. 24, 1–15. doi: 10.1104/pp.24.1.1
Bakshi, M., and Oelmüller, R. (2014). WRKY transcription factors: jack of many trades in plants. Plant Signal. Behav. 9:e27700. doi: 10.4161/psb.27700
Banerjee, A., and Roychoudhury, A. (2015). WRKY proteins: signalling and regulation of expression during abiotic stress responses. Sci. World J. 2015, 1–17. doi: 10.1155/2015/807560
Banerjee, A., and Roychoudhury, A. (2017). Abscisic-acid-dependent basic leucine zipper (bZIP) transcription factors in plant abiotic stress. Protoplasma 254, 3–16. doi: 10.1007/s00709-015-0920-4
Bates, L. S., Waldren, R. P., and Teare, E. D. (1973). Rapid determination of free proline for stress studies. Plant Soil 39, 205–208. doi: 10.1007/BF00018060
Bernoux, M., Timmers, T., Jauneau, A., Briere, C., de Wit, P. J., Marco, Y., et al. (2008). RD19, an Arabidopsis cysteine protease required for RRS1-R–mediated resistance, is relocalized to the nucleus by the Ralstonia solanacearum PopP2 effector. Plant Cell 20, 2252–2264. doi: 10.1105/tpc.108.058685
Binott, J. J., Owuoche, J. O., and Bartels, D. (2017). Physiological and molecular characterization of Kenyan barley (Hordeum vulgare L.) seedlings for salinity and drought tolerance. Euphytica 213:139. doi: 10.1007/s10681-017-1924-2
Birkenbihl, R. P., Liu, S., and Somssich, I. E. (2017). Transcriptional events defining plant immune responses. Curr. Opin. Plant Boil. 38, 1–9. doi: 10.1016/j.pbi.2017.04.004
Brand, L. H., Fischer, N. M., Harter, K., Kohlbacher, O., and Wanke, D. (2013). Elucidating the evolutionary conserved DNA-binding specificities of WRKY transcription factors by molecular dynamics and in vitro binding assays. Nucleic Acid Res. 41, 9764–9778. doi: 10.1093/nar/gkt732
Burke, J. J., and Chen, J. (2015). Enhancement of reproductive heat tolerance in plants. PLoS One 10:e0122933. doi: 10.1371/journal.pone.0122933
Cai, H., Yang, S., Yan, Y., Xiao, Z., Cheng, J., Wu, J., et al. (2015). CaWRKY6 transcriptionally activates CaWRKY40, regulates Ralstonia solanacearum resistance, and confers high-temperature and high-humidity tolerance in pepper. J. Exp. Bot. 66, 3163–3174. doi: 10.1093/jxb/erv125
Cai, R., Zhao, Y., Wang, Y., Lin, Y., Peng, X., Li, Q., et al. (2014). Overexpression of a maize WRKY58 gene enhances drought and salt tolerance in transgenic rice. Plant Cell Tissue Organ Cult. 119, 565–577. doi: 10.1007/s11240-014-0556-7
Chen, H., Lai, Z., Shi, J., Xiao, Y., Chen, Z., and Xu, X. (2010). Roles of Arabidopsis WRKY18, WRKY40 and WRKY60 transcription factors in plant responses to abscisic acid and abiotic stress. BMC Plant Biol. 10:281. doi: 10.1186/1471-2229-10-281
Chen, J., Nolan, T. M., Ye, H., Zhang, M., Tong, H., Xin, P., et al. (2017). Arabidopsis WRKY46, WRKY54, and WRKY70 transcription factors are involved in brassinosteroid-regulated plant growth and drought responses. Plant Cell 29, 1425–1439. doi: 10.1105/tpc.17.00364
Chu, X., Wang, C., Chen, X., Lu, W., Li, H., Wang, X., et al. (2015). The cotton WRKY gene GhWRKY41 positively regulates salt and drought stress tolerance in transgenic Nicotiana benthamiana. PLoS One 10:e0143022. doi: 10.1371/journal.pone.0143022
Chu, Y., Zhang, W., Wu, B., Huang, Q., Zhang, B., and Su, X. (2016). Overexpression of the novel Zygophyllum xanthoxylum C2H2-type zinc finger gene ZxZF improves drought tolerance in transgenic Arabidopsis and poplar. Biologia 71, 769–776. doi: 10.1515/biolog-2016-0093
Dai, X., Wang, Y., and Zhang, W. H. (2015). OsWRKY74, a WRKY transcription factor, modulates tolerance to phosphate starvation in rice. J. Exp. Bot. 67, 947–960. doi: 10.1093/jxb/erv515
Dang, F. F., Wang, Y. N., Yu, L., Lai, Y., Liu, Z. Q., Wang, X., et al. (2013). CaWRKY40, a WRKY protein of pepper, plays an important role in the regulation of tolerance to heat stress and resistance to Ralstonia solanacearum infection. Plant Cell Environ. 36, 757–774. doi: 10.1111/pce.12011
Ding, Z. J., Yan, J. Y., Li, G. X., Wu, Z. C., Zhang, S. Q., and Zheng, S. J. (2014). WRKY41 controls Arabidopsis seed dormancy via direct regulation of ABI3 transcript levels not downstream of ABA. Plant J. 79, 810–823. doi: 10.1111/tpj.12597
Doke, N. (1983). Involvement of superoxide anion generation in the hypersensitive response of potato tuber tissues to infection with an incompatible race of Phytophthora infestans and to the hyphal wall components. Physiol. Plant Pathol. 23, 435–457. doi: 10.1016/0048-4059(83)90019-X
Eulgem, T., Rushton, P. J., Robatzek, S., and Somssich, I. E. (2000). The WRKY superfamily of plant transcription factors. Trends Plant Sci. 5, 199–206. doi: 10.1016/S1360-1385(00)01600-9
Foyer, C. H., Trebst, A., and Noctor, G. (2008). “Signalling and integration of defense functions of tocopherol, ascorbate and glutathione,” in Photoprotection, Photoinhibition, Gene Regulation, and Environment, Vol. 21, eds B. Demmig-Adams, W. W. Adams, and A. K. Mattoo (Dordrecht: Springer), 241–268. doi: 10.1007/1-4020-3579-9_16
Garay-Arroyo, A., and Covarrubias, A. A. (1999). Three genes whose expression is induced by stress in Saccharomyces cerevisiae. Yeast 15, 879–892. doi: 10.1002/(SICI)1097-0061(199907)15:10A<879::AID-YEA428>3.0.CO;2-Q
Garg, A. K., Kim, J. K., Owens, T. G., Ranwala, A. P., Choi, Y. D., Kochian, L. V., et al. (2002). Trehalose accumulation in rice plants confers high tolerance levels to different abiotic stresses. Proc. Natl. Acad. Sci. U.S.A. 99, 15898–15903. doi: 10.1073/pnas.252637799
Giannopolitis, C. N., and Ries, S. K. (1977). Superoxide dismutases. Occurrence in higher plants. Plant Physiol. 59, 309–314. doi: 10.1104/pp.59.2.309
Goswami, L., Sengupta, S., Mukherjee, S., Ray, S., Mukherjee, R., and Majumder, A. L. (2014). Targeted expression of L-myo-inositol 1-phosphate synthase from Porteresia coarctata (Roxb.) Tateoka confers multiple stress tolerance in transgenic crop plants. J. Plant Biochem. Biotech. 23, 316–330. doi: 10.1007/s13562-013-0217-7
Grunewald, W., De Smet, I., De Rybel, B., Robert, H. S., Van De Cotte, B., Willemsen, V., et al. (2013). Tightly controlled WRKY23 expression mediates Arabidopsis embryo development. EMBO Rep. 14, 1136–1142. doi: 10.1038/embor.2013.169
Hennig, L. (2012). Plant gene regulation in response to abiotic stress. Biochim. Biophys. Acta 1819:85. doi: 10.1016/j.bbagrm.2012.01.005
Herrera-Estrella, L., de Block, M., Messens, E., Hernalsteens, J. P., Montagu, M. V., and Schell, J. (1983). Chimeric genes as dominant selectable markers in plant cells. EMBO J. 2, 987–995.
Hodges, D. M., DeLong, J. M., Forney, C. F., and Prange, R. K. (1999). Improving the thiobarbituric acid-reactive-substances assay for estimating lipid peroxidation in plant tissues containing anthocyanin and other interfering compounds. Planta 207, 604–611. doi: 10.1007/s004250050524
Hu, Y., Chen, L., Wang, H., Zhang, L., Wang, F., and Yu, D. (2013). Arabidopsis transcription factor WRKY8 functions antagonistically with its interacting partner VQ9 to modulate salinity stress tolerance. Plant J. 74, 730–745. doi: 10.1111/tpj.12159
Hu, Y., Dong, Q., and Yu, D. (2012). Arabidopsis WRKY46 coordinates with WRKY70 and WRKY53 in basal resistance against pathogen Pseudomonas syringae. Plant Sci. 185, 288–297. doi: 10.1016/j.plantsci.2011.12.003
Huang, S., Gao, Y., Liu, J., Peng, X., Niu, X., Fei, Z., et al. (2012). Genome wide analysis of WRKY transcription factors in Solanum lycopersicum. Mol. Genet. Genomics 287, 495–513. doi: 10.1007/s00438-012-0696-6
Jaleel, C. A., Manivannan, P., Wahid, A., Farooq, M., Al-Juburi, H. J., Somasundaram, R., et al. (2009). Drought stress in plants: a review on morphological characteristics and pigments composition. Int. J. Agric. Biol. 11, 100–105.
Jefferson, R. A. (1987). Assaying chimeric genes in plants, the GUS gene fusion system. Plant Mol. Biol. Rep. 5, 387–405. doi: 10.1007/BF02667740
Jiang, Y., Liang, G., and Yu, D. (2012). Activated expression of WRKY57 confers drought tolerance in Arabidopsis. Mol. Plant. 5, 1375–1388. doi: 10.1093/mp/sss080
Joshi, R., Wani, S. H., Singh, B., Bohra, A., Dar, Z. A., Lone, A. A., et al. (2016). Transcription factors and plants response to drought stress: current understanding and future directions. Front. Plant Sci. 7:1029. doi: 10.3389/fpls.2016.01029
Kaur, H., Verma, P., Petla, B. P., Rao, V., Saxena, S. C., and Majee, M. (2013). Ectopic expression of the ABA-inducible dehydration-responsive chickpea L-myo-inositol 1-phosphate synthase 2 (CaMIPS2) in Arabidopsis enhances tolerance to salinity and dehydration stress. Planta 237, 321–335. doi: 10.1007/s00425-012-1781-0
Kiranmai, K., Gunupuru, L. R., Nareshkumar, A., Reddy, V. A., Lokesh, U., Pandurangaiah, M., et al. (2016). Expression analysis of WRKY transcription factor genes in response to abiotic stresses in Horsegram (Macrotyloma uniflorum (Lam.) Verdc.). Am. J. Mol. Biol. 6, 125–137. doi: 10.4236/ajmb.2016.64013
Kumar, A. M., Reddy, K. N., Sreevathsa, R., Ganeshan, G., and Udayakumar, M. (2009). Towards crop improvement in bell pepper (Capsicum annuum L.): transgenics (uid A:: hpt II) by a tissue culture independent Agrobacterium mediated in planta approach. Sci. Hortic. 119, 362–370. doi: 10.1016/j.scienta.2008.08.034
Lai, Z., Vinod, K. M., Zheng, Z., Fan, B., and Chen, Z. (2008). Roles of Arabidopsis WRKY3 and WRKY4 transcription factors in plant responses to pathogens. BMC Plant Biol. 8:68. doi: 10.1186/1471-2229-8-68
Li, J., Wang, J., Wang, N., Guo, X., and Gao, Z. (2014). GhWRKY44, a WRKY transcription factor of cotton, mediates defence response to pathogen infection in transgenic Nicotiana benthamiana. Plant Cell Tissue Organ Cult. 121, 127–140. doi: 10.1007/s11240-014-0688-9
Li, J. F., Norville, J. E., Aach, J., McCormack, M., Zhang, D., Bush, J., et al. (2013). Multiplex and homologous recombination mediated genome editing in Arabidopsis and Nicotiana benthamiana using guide RNA and Cas9. Nat. Biotechnol. 31, 688–691. doi: 10.1038/nbt.2650
Li, S. J., Fu, Q. T., Chen, L. G., Huang, W. D., and Yu, D. Q. (2011). Arabidopsis thaliana WRKY25, WRKY26, and WRKY33 coordinate induction of plant thermo tolerance. Planta 233, 1237–1252. doi: 10.1007/s00425-011-1375-2
Liu, X., Song, Y., Xing, F., Wang, N., Wen, F., and Zhu, C. (2015). GhWRKY25, a group I WRKY gene from cotton, confers differential tolerance to abiotic and biotic stresses in transgenic Nicotiana benthamiana. Protoplasma 253, 1265–1281. doi: 10.1007/s00709-015-0885-3
Liu, Y., Wang, L., Xing, X., Sun, L., Pan, J., Kong, X., et al. (2013). ZmLEA3, a multifunctional group 3 LEA protein from maize (Zea mays L.), is involved in biotic and abiotic stresses. Plant Cell Physiol. 54, 944–959. doi: 10.1093/pcp/pct047
Livak, K. J., and Schmittgen, T. D. (2001). Analysis of relative gene expression data using real-time quantitative PCR and the 2-ΔΔCT method. Methods 25, 402–408. doi: 10.1006/meth.2001.1262
Luo, X., Bai, X., Sun, X., Zhu, D., Liu, B., Ji, W., et al. (2013). Expression of wild soybean WRKY20 in Arabidopsis enhances drought tolerance and regulates ABA signalling. J. Exp. Bot. 64, 2155–2169. doi: 10.1093/jxb/ert073
Miao, Y., and Zentgraf, U. (2007). The antagonist function of Arabidopsis WRKY53 and ESR/ESP in leaf senescence is modulated by the jasmonic and salicylic acid equilibrium. Plant Cell 19, 819–830. doi: 10.1105/tpc.106.042705
Nakano, Y., and Asada, K. (1981). Hydrogen peroxide is scavenged by Ascorbate-specific peroxidase in spinach chloroplasts. Plant Cell Physiol. 22, 867–880. doi: 10.1093/oxfordjournals.pcp.a076232
Nakashima, K., Yamaguchi-Shinozaki, K., and Shinozaki, K. (2014). The transcriptional regulatory network in the drought response and its crosstalk in abiotic stress responses including drought, cold, and heat. Front. Plant Sci. 5:170. doi: 10.3389/fpls.2014.00170
Niu, C. F., Wei, W., Zhou, Q. Y., Tian, A. G., Hao, Y. J., Zhang, W. K., et al. (2012). Wheat WRKY genes TaWRKY2 and TaWRKY19 regulate abiotic stress tolerance in transgenic Arabidopsis plants. Plant Cell Environ. 35, 1156–1170. doi: 10.1111/j.1365-3040
Niu, F., Wang, B., Wu, F., Yan, J., Li, L., Wang, C., et al. (2014). Canola (Brassica napus L.) NAC103 transcription factor gene is a novel player inducing reactive oxygen species accumulation and cell death in plants. Biochem. Biophys. Res. Commun. 454, 30–35. doi: 10.1016/j.bbrc.2014.10.057
Peleg, Z., and Blumwald, E. (2011). Hormone balance and abiotic stress tolerance in crop plants. Curr. Opin. Plant Biol. 14, 290–295. doi: 10.1016/j.pbi.2011.02.001
Qin, Y., Tian, Y., and Liu, X. (2015). A wheat salinity-induced WRKY transcription factor TaWRKY93 confers multiple abiotic stress tolerance in Arabidopsis thaliana. Biochem. Biophys. Res. Commun. 464, 428–433. doi: 10.1016/j.bbrc.2015.06.128
Rahdari, P., and Hoseini, S. M. (2012). Drought stress, a review. Int. J. Agron. Plant Prod. 3, 443–446.
Rahdari, P., Hoseini, S. M., and Tavakoli, S. (2012). The studying effect of drought stress on germination, proline, sugar, lipid, protein and chlorophyll content in Purslane (Portulaca oleracea L.) leaves. J. Med. Plants Res. 6, 1539–1547. doi: 10.5897/JMPR11.698
Rampino, P., Pataleo, S., Gerardi, C., and Perotta, C. (2006). Drought stress responses in wheat: physiological and molecular analysis of resistant and sensitive genotypes. Plant Cell Environ. 29, 2143–2152. doi: 10.1111/j.1365-3040.2006.01588.x
Rinerson, C. I., Rabara, R. C., Tripathi, P., Shen, Q. J., and Rushton, P. J. (2015a). The evolution of WRKY transcription factors. BMC Plant Biol. 15:66. doi: 10.1186/s12870-015-0456-y
Rinerson, C. I., Scully, E. D., Palmer, N. A., Donze-Reiner, T., Rabara, R. C., Tripathi, P., et al. (2015b). The WRKY transcription factor family and senescence in switchgrass. BMC Genomics 16:912. doi: 10.1186/s12864-015-2057-4
Rohini, V. K., and Rao, K. S. (2001). Transformation of peanut (Arachis hypogaea L.) with tobacco chitinase gene: variable response of transformants to leaf spot disease. Plant Sci. 160, 889–898. doi: 10.1016/S0168-9452(00)00462-3
Rushton, P. J., Somssich, I. E., Ringler, P., and Shen, Q. J. (2010). WRKY transcription factors. Trends Plant Sci. 15, 247–258. doi: 10.1016/j.tplants.2010.02.006
Sarris, P. F., Duxbury, Z., Huh, S. U., Ma, Y., Segonzac, C., Sklenar, J., et al. (2015). A plant immune receptor detects pathogen effectors that target WRKY transcription factors. Cell 161, 1089–1100. doi: 10.1016/j.cell.2015.04.024
Sathiyaraj, G., Lee, O. R., Parvin, S., Khorolragchaa, A., Kim, Y. J., and Yang, D. C. (2011). Transcript profiling of antioxidant genes during biotic and abiotic stresses in Panax ginseng C. A. Meyer. Mol. Biol. Rep. 38, 2761–2769. doi: 10.1007/s11033-010-0421-7
Schluttenhofer, C., and Yuan, L. (2014). Regulation of specialized metabolism by WRKY transcription factors. Plant Physiol. 16, 295–306. doi: 10.1104/pp.114.251769
Selvakumar, G., Panneerselvam, P., and Ganeshamurthy, A. N. (2012). “Bacterial mediated alleviation of abiotic stress in crops,” in Bacteria in Agrobiology: Stress Management, ed. D. K. Maheshwari (Berlin: Springer-Verlag), 205–224. doi: 10.1007/978-3-662-45795-5_10
Seo, P. J., Lee, S. B., Suh, M. C., Park, M. J., Go, Y. S., and Park, C. M. (2011). The MYB96 transcription factor regulates cuticular wax biosynthesis under drought conditions in Arabidopsis. Plant Cell 23, 1138–1152. doi: 10.1105/tpc.111.083485
Shao, H., Wang, H., and Tang, X. (2015). NAC transcription factors in plant multiple abiotic stress responses: progress and prospects. Front. Plant Sci. 6:902. doi: 10.3389/fpls.2015.00902
Shen, H., Liu, C., Zhang, Y., Meng, X., Zhou, X., Chu, C., et al. (2012). OsWRKY30 is activated by MAP kinases to confer drought tolerance in rice. Plant Mol. Biol. 80, 241–253. doi: 10.1007/s11103-012-9941-y
Shi, W., Hao, L., Li, J., Liu, D., Guo, X., and Li, H. (2014). The Gossypium hirsutum WRKY gene GhWRKY39-1 promotes pathogen infection defense responses and mediates salt stress tolerance in transgenic Nicotiana benthamiana. Plant Cell Rep. 33, 483–498. doi: 10.1007/s00299-013-1548-5
Shin, D., Moon, S. J., Han, S., Kim, B. G., Park, S. R., Lee, S. K., et al. (2011). Expression of StMYB1R-1, a novel potato single MYB like domain transcription factor, increases drought tolerance. Plant Physiol. 155, 421–432. doi: 10.1104/pp.110.163634
Singh, M., Kumar, J., Singh, S., Singh, V. P., and Prasad, S. M. (2015). Roles of osmoprotectants in improving salinity and drought tolerance in plants: a review. Rev. Environ. Sci. Biotechnol. 14, 407–426. doi: 10.1007/s11157-015-9372-8
Singh, S., Eapen, S., and D’souza, S. F. (2006). Cadmium accumulation and its influence on lipid peroxidation and antioxidative system in an aquatic plant, Bacopa monnieri L. Chemosphere 62, 233–246. doi: 10.1016/j.chemosphere.2005.05.017
Skibbe, M., Qu, N., Galis, I., and Baldwin, I. T. (2008). Induced plant defenses in the natural environment: Nicotiana attenuata WRKY3 and WRKY6 coordinate responses to herbivory. Plant Cell 20, 1984–2000. doi: 10.1105/tpc.108.058594
Surendran, U., Kumar, V., Ramasubramoniam, S., and Raja, P. (2017). Development of drought indices for semi-arid region using drought indices calculator (DrinC)–a case study from Madurai District, a semi-arid region in India. Water Resour. Manag. 31, 3593–3605. doi: 10.1007/s11269-017-1687-5
Suzuki, N., Koussevitzky, S., Mittler, R. O. N., and Miller, G. A. D. (2012). ROS and redox signalling in the response of plants to abiotic stress. Plant Cell Environ. 35, 259–270. doi: 10.1111/j.1365-3040.2011.02336.x
Tang, L., Cai, H., Zhai, H., Luo, X., Wang, Z., Cui, L., et al. (2014). Overexpression of Glycine soja WRKY20 enhances both drought and salt tolerance in transgenic alfalfa (Medicago sativa L.). Plant Cell Tissue and Organ Cult. 118, 77–86. doi: 10.1007/s11240-014-0463-y
Wang, C., Deng, P., Chen, L., Wang, X., Ma, H., Hu, W., et al. (2013). A wheat WRKY transcription factor TaWRKY10 confers tolerance to multiple abiotic stresses in transgenic tobacco. PLoS One 8:e65120. doi: 10.1371/journal.pone.0065120
Wang, X., Zeng, J., Li, Y., Rong, X., Sun, J., Sun, T., et al. (2015). Expression of TaWRKY44, a wheat WRKY gene, in transgenic tobacco confers multiple abiotic stress tolerances. Front. Plant Sci. 6:615. doi: 10.3389/fpls.2015.00615
Wang, Z., Zhu, Y., Wang, L. L., Liu, X., Liu, Y. X., Phillips, J., et al. (2009). A WRKY transcription factor participates in dehydration tolerance in Boea hygrometrica by binding to the W-box elements of the galactinol synthase (BhGolS1) promoter. Planta 230, 1155–1166. doi: 10.1007/s00425-009-1014-3
Wu, X., Shiroto, Y., Kishitani, S., Ito, Y., and Toriyama, K. (2009). Enhanced heat and drought tolerance in transgenic rice seedlings overexpressing OsWRKY11 under the control of HSP101 promoter. Plant Cell Rep. 28, 21–30. doi: 10.1007/s00299-008-0614-x
Yan, H., Jia, H., Chen, X., Hao, L., An, H., and Guo, X. (2014). The cotton WRKY transcription factor GhWRKY17 functions in drought and salt stress in transgenic Nicotiana benthamiana through ABA signaling and the modulation of reactive oxygen species production. Plant Cell Physiol. 55, 2060–2076. doi: 10.1093/pcp/pcu133
Zhai, H., Wang, F., Si, Z., Huo, J., Xing, L., An, Y., et al. (2016). A myo-inositol-1-phosphate synthase gene, IbMIPS1, enhances salt and drought tolerance and stem nematode resistance in transgenic sweet potato. Plant Biotech. J. 14, 592–602. doi: 10.1111/pbi.12402
Zheng, L., Liu, G., Meng, X., Liu, Y., Ji, X., Li, Y., et al. (2013). A WRKY gene from Tamarix hispida, ThWRKY4, mediates abiotic stress responses by modulating reactive oxygen species and expression of stress-responsive genes. Plant Mol. Biol. 82, 303–320. doi: 10.1007/s11103-013-0063-y
Keywords: MuWRKY3 TF, drought stress tolerance, transgenic groundnut, stress-responsive genes, ROS, antioxidative metabolism
Citation: Kiranmai K, Lokanadha Rao G, Pandurangaiah M, Nareshkumar A, Amaranatha Reddy V, Lokesh U, Venkatesh B, Anthony Johnson AM and Sudhakar C (2018) A Novel WRKY Transcription Factor, MuWRKY3 (Macrotyloma uniflorum Lam. Verdc.) Enhances Drought Stress Tolerance in Transgenic Groundnut (Arachis hypogaea L.) Plants. Front. Plant Sci. 9:346. doi: 10.3389/fpls.2018.00346
Received: 17 November 2017; Accepted: 01 March 2018;
Published: 16 March 2018.
Edited by:
Jens Kossmann, Stellenbosch University, South AfricaReviewed by:
Shabir Hussain Wani, Michigan State University, United States (Abbu Zaid contributed to the review of Shabir Hussain Wani)Sanjib Kumar Panda, Assam University, India
Copyright © 2018 Kiranmai, Lokanadha Rao, Pandurangaiah, Nareshkumar, Amaranatha Reddy, Lokesh, Venkatesh, Anthony Johnson and Sudhakar. This is an open-access article distributed under the terms of the Creative Commons Attribution License (CC BY). The use, distribution or reproduction in other forums is permitted, provided the original author(s) and the copyright owner are credited and that the original publication in this journal is cited, in accordance with accepted academic practice. No use, distribution or reproduction is permitted which does not comply with these terms.
*Correspondence: Chinta Sudhakar, chintasudhakar@yahoo.com